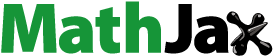
ABSTRACT
To explore the effect of pressure on the combustion and luminescence properties of magnesium/nitrate pyrotechnics, combustion tests and thermal analysis of magnesium/sodium nitrate (N) and magnesium/strontium nitrate (S) pyrotechnic samples with zero oxygen balance were conducted under the pressure range of 101kPa-1kPa in this study. The results show that the flame height of N increases continuously as the pressure decreases, and the flames gradually change from a steady-state convergent flame to a turbulent evanescent flame. However, due to the different diffusion rates of the gas-phase combustion products, the diffusion of S at low pressure is lower and the flame still maintains a stable laminar flow. Although the light intensity and burning speed of both N and S satisfy Vier’s law, the luminous intensity of S is less sensitive to pressure and still has good luminous performance at 1 kPa. Thermal analysis test results show that N and S can react almost completely at atmospheric pressure, and their enthalpies of reaction are 6.75 kJ/g and 6.07 kJ/g, respectively. The difference in the thermal decomposition histories of strontium nitrate and sodium nitrate at 1 kPa results in a reaction enthalpy of 4.24 kJ/g for S and only 2.45 kJ/g for N. This indicates that the reaction degree of S at low pressure is higher than that of N. The SEM-EDS test results further confirm this opinion. These scientific findings provide useful insights into the low-pressure combustion behavior of pyrotechnics and support the growing need for military and civilian applications.
Introduction
Magnesium/nitrate mixtures are the most common pyrotechnics and are widely used in military and civilian applications, such as illuminators, signaling agents, decoys, and propellants (Bagherpour, Mahdavi, and Abedini Citation2019; Johnston Citation2002; Sadek et al. Citation2017; Singh, Somayajulu, and Bhaskara Rao Citation1989). So far, many scholars have studied the reaction mechanism, combustion performance and luminescence of magnesium/nitrate system pyrotechnics at atmospheric pressure. It is generally considered that magnesium, which acts as a combustible agent, is oxidized and releases a large amount of heat during the combustion process, providing sufficient energy to maintain the stable combustion of pyrotechnics. Magnesium oxide is a typical incandescent emitter, producing an intense white light in a high temperature flame (Conkling and Mocella Citation2019; Tuukkanen et al. Citation2006; Wada Citation1995). As an oxidizer, nitrate decomposes during combustion to provide oxygen for the combustion of pyrotechnics. At the same time, the colorful light emitters produced by nitrate decomposition will give the flame a light signal of different colors. Sodium nitrate and strontium nitrate, as common nitrates, provide yellow and red characteristic emitters during burning process, respectively. Magnesium/nitrate pyrotechnic mixtures undergo two main reaction phases during combustion: Firstly, magnesium and molten nitrate react in the condensed phase to produce magnesium oxide, nitrogen gas and colored light emitters; then, the residual magnesium undergoes secondary combustion with oxygen of air in the gas phase flame. The reaction equations for the whole process are shown below (Pouretedal and Ebadpour Citation2014; Pouretedal and Mousavi Citation2018; Pouretedal and Ravanbod Citation2015; Tuukkanen et al. Citation2004).
With the advancement of human aerospace technology today, pyrotechnics are increasingly being used in high-altitude environments (Li et al. Citation2018; Woodley et al. Citation2017). Magnesium/nitrate system pyrotechnics have good thermal and aerodynamic properties as well as luminescence, so they have a broad application prospect. However, the low pressure and low oxygen content of the high level space can affect the combustion and luminescence performance of pyrotechnics. B. D. bond et al. performed thermal analysis tests on magnesium/sodium nitrate binary mixtures under argon atmosphere and found that the low oxygen environment leads to a lower oxidation rate of magnesium, which results in a delayed ignition temperature (Bond and Jacobs Citation1966). Bernard E. et al. found that the visible spectral radiant flux intensity and radiant power of magnesium/sodium nitrate mixtures decreased with decreasing pressure, but he did not explain the reason for this (Douda, Blunt, and Bair Citation1970). Changjiang Z. et al. found that the subatmospheric environment accelerates the rapid diffusion of high-temperature gas products, which weakens the luminescence performance of magnesium/sodium nitrate tracers (Zhu, Wang, and Pan Citation1996). Kuma et al. suggested that the rapid diffusion of gaseous decomposition products at low pressure makes it difficult to achieve stoichiometric ratios between the fuel and the oxidizer, which leads to a decrease in reaction efficiency (Kumar, Varshney, and Manash Citation2019). Guo et al. discovered that the low oxygen concentration in a low-pressure environment, along with the quick passage of gaseous products, results in a lower degree of reaction and, as a result, decreased luminosity of magnesium/sodium nitrate pyrotechnics (Guo et al. Citationn.d.).However, these studies have focused on a single system of magnesium/sodium nitrate, without comparing different pyrotechnics of magnesium/nitrate systems in order to find the system with optimal combustion and luminescence performance at low pressure.
In order to compare the difference of burning performance and luminescence of different magnesium nitrate pyrotechnics in high altitude environment, four sets of zero oxygen balance magnesium/sodium nitrate (N) and magnesium/strontium nitrate (S) pyrotechnic mixtures were designed for this study. The flame morphology and luminescence efficiency of these four groups of samples under low-pressure environment were compared by low-pressure combustion tests. The effect of pressure on the degree of reaction was investigated using a NETZSCH STA449F3 (USA) simultaneous thermal analyzer, a scanning electron microscope, and an energy dispersive spectrometer. The results of the study provide a reference for the application of pyrotechnics in low-pressure environments.
Experimental section
Sample preparation
The difference between the oxygen content of pyrotechnics and the oxygen content required for the complete oxidation of combustible agents is defined as the oxygen balance of pyrotechnics. Taking the ternary mixture as an example, the calculation formula of oxygen balance is as follows:
Where n is the oxygen balance, A is the mass of the oxidant, a is the mass of the oxidant required to decompose 1 g of oxygen, B is the mass of the combustible agent, b is the mass of the combustible agent that can be oxidized by 1 g oxygen, and C is the mass of the binder, C is the mass of the binder that can be oxidized by 1 g of oxygen. When the oxygen balance is zero, the oxygen carried by the pyrotechnic powder can completely oxidize the combustible without the participation of external oxygen.
The mass fractions of the four zero-oxygen balance magnesium/nitrate/phenolic resin pyrotechnic mixtures are shown in . Firstly, the nitrate sieved through 160 mesh was dried in an oven at 60°C for 6 h. The appropriate amount of magnesium powder (100 mesh to 200 mesh) and the dried nitrate were weighed separately for dry mixing according to the ratio of zero oxygen balance. After the dry mixing, the mixture was poured into the ethanol solution with phenolic resin for wet mixing. The wet-mixed mixture was sieved through 30 mesh and dried in an oven at 60°C for 2 h. 2 g of the dried powder was weighed and poured into a cylindrical mold with a diameter of 12.0 ± 0.1 mm and pressed into a pillar with a diameter of 12.0 ± 0.1 mm and a height of 11.0 ± 0.1 mm using a YQ32-400T press. The surface of the pillar was wrapped three times with ZnO flame retardant tape to prevent leakage of fire. The ignition end of the pillar was covered with 0.2 g of boron/barium chromate for ignition. The functions and suppliers of the chemicals used are shown in .
Table 1. Content of each component of the samples.
Table 2. Functions and physicochemical parameters of chemicals.
Combustion test
The The combustion test system mainly includes five parts: regulated DC power supply, vacuum tank, transient light intensity meter, spectrophotometer and thermal imaging camera. The schematic diagram of its composition is shown in .
A regulated DC power supply was used as the ignition power source, and its output power was set to 9 v/2A. A 2 mm diameter NiCr resistance wire with 34.7 ohm per meter resistance was used to electrically fire the sample surface. The low-pressure combustion chamber was a cylindrical steel tank 9 m long and 3 m in diameter with 30 mm diameter tempered glass sight windows on both sides and ends. The samples were placed inside the low-pressure combustion chamber, and two sets of parallel tests were conducted on the samples under pressure conditions of 101 kPa, 80kPa, 60kPa, 40kPa, 20kPa and 1 kPa, respectively. The low pressure combustion test is carried out in an air atmosphere. The vacuum pump was started before the experiment and turned off when the value on the pressure gauge reached the required value for the test. Since the volume of the vacuum tank reaches 50 m3, the pressure inside the tank is almost constant during the test. The thermal imaging camera, located at 3 m from the sample in the observation window, recorded the flame state of the sample at a frame rate of 240 Hz. SGX-100 transient light intensity meter (production of Chinese Xi’an Research Institute of Applied Optics, test range 101-108cd, test accuracy 96%) and ocean optics USB 4000 spectrophotometer (production of USA ocean optics, test range 200 to 1100 nm, test accuracy 96%, maximum intensity 65,000 counts) are placed at a visible light perspective window 1.5 m away from the sample. The sampling frequency of SGX-100 transient light intensity meter is set to 400 Hz, and the sampling time is set to 5s. The integration time of ocean optics USB 4000 spectrophotometer is set to 1 ms.
TG-DSC and SEM-EDS tests
The TG-DSC test was carried out with the NETZSCH STA449F3 (USA) synchronous thermal analyzer. The sample container is a 50 μL alumina crucible. The aluminum oxide powder was used as the reference material. In an air atmosphere, the samples were subjected to thermal analysis at pressures of 101kPa and 1kPa, respectively. At a heating rate of 10K/min, the sample was heated from room temperature to 800°C. Meanwhile, Hitachi Regulus-8100 SEM was used in this study to observe the microstructure of the combustion products of the samples at an accelerating voltage of 20 KV and a magnification of 5000 times. The energy dispersive spectrometer EDS was also used to test the content of each component in the combustion products.
Results and discussion
Effect of pressure on flame structure
shows the flame states of N and S during steady combustion. Under 101 kPa, a bright white conical flame is formed on the burning surface of the pillar. As the pressure decreases, the flame height gradually increases and an unstable turbulent flame appears at the top. The reason for this is that in a low-pressure environment, a drop in air density diminishes the influence of buoyancy, promoting random diffusion of gas-phase combustion products. At 101 kPa, the flame diffuses mainly along the axial direction and forms a cylindrical diffusion zone above the combustion surface due to the strong buoyancy force. This flame structure is defined as the convergent flame model (Yan et al. Citation2009). As the pressure gradually decreases, the weakened buoyancy leads to random diffusion of the flame in the gas-phase reaction zone and the appearance of a distinct turbulent zone at the flame front.
Comparing , it can be observed that there are significant differences in the sensitivity of the flame states of N and S to the pressure. At atmospheric pressure they have the identical form and almost the same height, and both are conical flames under the action of strong buoyancy. As the pressure decreases, the flame height of both gradually increases. The difference is that the flame height of the N sample, as well as its flame tip turbulence zone, are significantly larger than S in the low-pressure environment. According to reference [20], it is known that the flame produced by the combustion of magnesium/nitrate pyrotechnics is a non-premixed diffusion flame, and the height of this flame is related to the diffusion rate of the gas. The diffusion rate of the gas phase products satisfies Fick’s law of diffusion (Weissler and Carls Citation1979).
Where dA is the unit area, dM is the diffusion rate, D is the diffusion coefficient, T is the ambient temperature, P is the ambient pressure, k is the Boltzmann constant, σ is the diameter of a gas molecule, and m is the mass of a single gas molecule. According to EquationEquation (5)(5)
(5) and EquationEquation (6)
(6)
(6) , the diffusion rate of molecules of the same gas is negatively related to the ambient pressure when the ambient temperature is constant. Hence, the accelerated diffusion rate of gas-phase products in low-pressure environments leads to an increase in flame height. Meanwhile, it can be seen from EquationEquation (6)
(6)
(6) that the diffusion coefficient of the gas is negatively related to the mass of the gas molecules when the ambient temperature and pressure are constant. According to , it can be seen that the combustion products of magnesium/sodium nitrate pyrotechnics at atmospheric pressure mainly consist of incandescent emitter magnesium oxide and yellow emitter sodium atoms (Conkling and Mocella Citation2019). At 1 kPa, the characteristic peak of MgO disappears and almost only sodium atoms are present in the gas-phase flame of N. As can be seen from , the main combustion products of magnesium/strontium nitrate pyrotechnics at atmospheric pressure are the red light emitters (SrOH, SrH, SrO) and the white light emitter MgO (Sabatini et al. Citation2015). At 1 kPa, the characteristic peaks of MgO disappear and those of SrH and SrO are faint, but the band spectra of SrOH molecules remain significant. This indicates that the gas phase flame of magnesium/strontium nitrate pyrotechnics at low pressure is mainly SrOH molecules. Since the molecular mass of SrOH is much larger than that of Na atom, it is known from EquationEquation (6)
(6)
(6) that the gas phase flame diffusion rate of S sample is smaller than that of N sample under the same environment. Therefore, the flame height of N is higher and its degree of turbulence is more obvious. This idea is also well illustrated by the observations of infrared thermal imaging cameras. As shown in , the flame structures of both N and S at atmospheric pressure consist of a high-temperature combustion zone in the middle and a low-temperature product zone in the outer layer. As the pressure decreases, the high-temperature combustion zone of N is gradually reduced. Under 1kPa, the heat in the gas-phase flame is also accelerated due to the rapid diffusion of the gas. Therefore, the high-temperature combustion zone of N is concentrated only on the combustion surface, and the main body of the flame is composed of low-temperature gas-phase products. On the contrary, the high temperature combustion region of S is almost unaffected by the pressure and maintains a large area even at 1 kPa.
The effect of pressure on luminous performance
The decrease in pressure not only changes the flame structure, but also affects the luminescence performance. . shows the light intensity (I)-time (t) curves recorded by the transient light intensity meter at different pressures. As the pressure decreases, the luminous time continues to extend, but the luminous intensity gradually decreases. The average luminescence intensity of the sample was calculated using light intensity data from the platform area of the I-t curve, as illustrated in . The time of sample burning was counted and the linear burning rate was determined using the I-t curve. The burning rate and average luminous intensity at different pressures are shown in . From atmospheric pressure to 1 kPa, the luminous intensity of N was reduced from 13,053.7 cd·s−1 to 399.3 cd·s−1 and the burning rate from 10.9 mm/s−1 to 2.9 mm/s−1; the luminous intensity of S was reduced from 12,477.3 cd·s−1 to 2309.6 cd·s−1 and the burning rate from 10.8 mm/s−1 to 2.2 mm/s−1.
Table 3. Burning rate and luminous intensity.
Pyrotechnics carry out chemical reactions in the form of combustion, releasing energy out as heat, which then heats the combustion products and produces light radiation effects. Therefore the rate of combustion directly determines the speed of energy release. According to Vier’s law (Kumar, Joshi, and Kumar Citation2016), the rate of ignition of pyrotechnics satisfies EquationEquation (7)(7)
(7) :
where v is the burning velocity in mm/s, a is the burning velocity coefficient, n is the pressure burning velocity index, and P is the ambient pressure in Pa. In order to study the relationship between linear burning rate and pressure more intuitively, the Vier formula is logarithmically transformed and linearly fitted in this paper, and the results obtained are shown in . The autocorrelation coefficients of the fitted curves for N and S were 0.99619 and 0.99324, respectively, which indicated that the burning rate of magnesium/nitrate pyrotechnics in a low-pressure environment was consistent with Vier’s law. Since the burning velocity of pyrotechnics directly determines the speed of energy release, the trend of light intensity with pressure is theoretically consistent with the burning velocity. Assuming that the light intensities of the samples also obey Vier’s formula, the light intensity and pressure should satisfy EquationEquation (8)(8)
(8) :
Where I is the light intensity in cd, b is the light intensity coefficient, and m is the pressure light intensity index.The data in were substituted into EquationEquation (8)(8)
(8) and perform a linear fit to obtain the fitted curve as shown in . The fitted curves for N and S have autocorrelation coefficients of 0.99138 and 0.99011, respectively, which indicates that the light intensity of magnesium/nitrate pyrotechnics also satisfies Vier’s law. The low-pressure light intensity coefficient of N is 383.707 and the low-pressure light intensity index is 0.759, while the low-pressure light intensity coefficient of S is 2239.237 and the low-pressure light intensity index is 0.358.
N has good luminescence performance at atmospheric pressure. However, at 1 kPa, the luminous intensity of N is much lower than that of S. Comparing , it can be seen that S has a lower luminous intensity pressure index. This indicates that the luminescence performance of magnesium/strontium nitrate pyrotechnics is less sensitive to pressure. This paper speculates that this phenomenon is related to a variety of factors. First, the difference in combustion products results in a greater degree of gas-phase flame spread for N at low pressures. The rapid diffusion of gas products will not only lead to a reduction in the concentration of luminous particles per unit area, but also intensify the heat loss in the flame area, resulting in a sudden reduction in the high-temperature combustion zone. According to reference (Agrawal Citation2010), the luminous properties of pyrotechnics satisfy the Stephen Boltzmann law:
Where M is the light radiation energy, σ is the Stephen Boltzmann constant, and T is the flame temperature. According to EquationEquation (9)(9)
(9) , it is known that the luminous intensity of pyrotechnics depends on the flame temperature. The luminous properties of N also decays sharply at low pressure due to the plunge in the high temperature combustion zone. Secondly, the different optical emitters of N and S lead to their different spectral properties. As can be seen from , the main luminescent material of N is the linear spectral emitter Na atom. With the decrease of pressure, the double peak intensity as well as the half-peak width of Na are decreasing. The main light-emitting product of S is the SrOH molecule, and the intensity of its band spectrum also decreases with the decrease of pressure. However, the half-peak width is almost independent of the pressure, and even at 1 kPa, the width of the characteristic SrOH peak is consistent with that at atmospheric pressure. Therefore, the S sample has a high spectral radiant energy even in a low-pressure environment.
The effect of pressure on the degree of reaction
Since the samples designed for this study are in zero oxygen balance, the oxygen carried by themselves theoretically ensures the complete reaction of the system. However, it is known from reference (Guo et al. Citationn.d..) that the degree of reaction of magnesium/sodium nitrate pyrotechnics with zero oxygen balance decreases with decreasing pressure. It is tentatively presumed that the degree of reaction of S samples also decreases at low pressure. However, due to the different thermal decomposition histories of strontium nitrate and sodium nitrate, there are some differences in the effect of low-pressure environment on the reaction degrees of N and S. Therefore, in this study, low-pressure thermal analysis tests were performed on N and S and the degree of response of the two was compared, as shown below.
As shown in , the reaction process of magnesium/sodium nitrate/phenolic resin pyrotechnic composition under atmospheric pressure can be roughly divided into three stages: In the first stage, from 390°C to 450°C, sodium nitrate begins to slowly decompose, causing the TG curve to begin to decrease. The DSC curve shows a small exothermic peak near 420°C, which is caused by the oxidation of a small amount of phenolic resin in the sample. The main chemical reactions in this stage are shown in equations (10) and (11).
In the second stage, from 450°C to 500°C, the TG curve is almost unchanged. At this stage, magnesium powder and molten sodium nitrate react in the condensed phase, and the reaction process is shown in EquationEquation (12)(12)
(12) (Freeman Citation1956). The TG curve begins to increase slowly as the temperature continues to rise. During this process, magnesium powder begins to be oxidized by oxygen in the air and slowly releases heat, as illustrated in EquationEquation (13)
(13)
(13) .
In the third stage, from 550°C to 580°C, the heat accumulation due to the exothermic oxidation of magnesium powder promotes the decomposition of NaNO3 and NaNO2, thereby also accelerating the oxidation reaction rate of magnesium powder. It can be seen from the DSC curve that the exothermic heat of the sample increases sharply at 550°C, and reaches the peak reaction temperature at 555°C. The chemical reactions occurring at this stage are shown in equations (13), equations (14) and equations (15). Due to the rapid decomposition of sodium nitrate and sodium nitrite, the TG curve appears to drop sharply [9].
As can be seen from , the reaction course of S at atmospheric pressure has only two stages. At 450°C, the oxidative decomposition of the phenolic resin in leads to a slight decrease in the TG curve. The decomposition of strontium nitrate at 525°C causes the TG curve to drop again, at which time the magnesium powder also begins to oxidize. The DSC curve starts to rise from 550°C and reaches the peak temperature at 575°C. The chemical reaction occurring in this process is shown in EquationEquation (16)(16)
(16) .
The TG-DSC curves of magnesium/sodium nitrate pyrotechnics at 1 kPa are shown in . In contrast to the atmospheric pressure, the reaction history of the N sample at 1 kPa is divided into two main stages. In the first stage, 400°C~550°C, the decomposition of sodium nitrate and the oxidative decomposition of phenolic resin led to a slow decrease of TG curve, while a weak exothermic peak appeared in DSC. Unlike the atmospheric pressure, the TG curve continues to decrease after 500°C without an upward trend. Because the oxygen content at 1kPa is 101/1 of that at atmospheric pressure, it is clear from the reaction kinetics that it is difficult for magnesium to react with oxygen in air under this condition. In the second stage, 550°C~620°C, the magnesium powder started to be oxidized by sodium nitrate and sodium nitrite. A sharp exothermic peak appeared in the DSC curve and reached the peak temperature at 578°C. The exothermic oxidation of magnesium promotes the decomposition of sodium nitrate and sodium nitrite, resulting in a sudden drop in the TG curve.
The total weight loss of N at 1 kPa reaches 36.7%, which is 20.4% more than the weight loss at atmospheric pressure. This is due to the rapid rate of gas diffusion in low-pressure environments (Guo et al. Citationn.d..). According to equations (5) and (6), the gas diffusion rate increases by a factor of 101 when the pressure is reduced from 101 kPa to 1 kPa. Therefore the oxygen decomposed by the oxidizer at 1kPa will rapidly diffuse into the environment leading to a decrease in the efficiency of the oxidation reaction of magnesium powder.
The TG-DSC curves of magnesium/strontium nitrate pyrotechnics at 1 kPa are shown in . The low-pressure reaction course of S is similar to that at atmospheric pressure. Sr(NO3)2 starts to decompose at 525°C, when the TG curve starts to decrease. Unlike at atmospheric pressure, the initial reaction temperature and the peak temperature of the reaction are delayed. The DSC curve started to rise from 575°C and reached the peak temperature at 601.5°C. Similarly, the degree of reaction of S at low pressure was slightly reduced due to the rapid diffusion of gaseous products, and the weight loss increased from 17% to 23.2%. The chemical reaction that occurs in this process is shown in EquationEquation (19)(19)
(19) .
When the magnesium/sodium nitrate pyrotechnics in zero oxygen balance react completely, the binder decomposes to produce gaseous carbon oxides and water, the nitrate decomposes to produce metal oxides in the condensed phase and gaseous products N2 and O2, and Mg is completely oxidized to produce the solid product MgO. The theoretical weight loss for N and S should be 16.9% and 15.7%, respectively, with reaction enthalpy changes of −8.04 kJ/g and −7.19 kJ/g, respectively (Wagman Citation1982). Analyzing the data in , it can be found that the values of weight loss and enthalpy change of reaction for N and S at atmospheric pressure are close to the theoretically calculated values. This indicates that they can react almost completely at atmospheric pressure. However, the enthalpy change of the reaction of N at 1 kPa is only 2.45 kJ/g, while the enthalpy change of the reaction of S can reach 4.24 kJ/g. This indicates that the reaction level of S is significantly higher than that of N at low pressure. This paper speculates that this phenomenon may be caused by the difference in their thermal decomposition processes. Sodium nitrate starts to decompose at 400°C. At this point it has not yet reached the temperature of its reaction with magnesium oxidation. Therefore, the rapid diffusion of partially decomposed oxygen at low pressure leads to a further reduction in the oxidation of magnesium. The decomposition temperature of strontium nitrate is close to the oxidation temperature of magnesium, even though in low-pressure environment the decomposition of strontium nitrate and oxidation of magnesium proceed almost simultaneously. Therefore, even at 1 kPa, S still has an excellent reaction performance.
Table 4. Thermal decomposition parameters of samples.
To further illustrate the difference in the degree of reaction between N and S samples at low pressures, combustion products at 101 kPa, 40 kPa and 1 kPa were collected and subjected to SEM-EDS tests. As shown in , the combustion products of N at atmospheric pressure contain mainly three elements, Mg, Na and O. As shown in , the flame of N contains mainly MgO, Mg, and sodium atoms. The sodium atoms in the flame are only intermediate products and end up as Na2O in the air. As can be seen from , the combustion products of S samples at atmospheric pressure contain mainly Mg, Sr and O elements. As shown in , the flame of S contains mainly MgO, Mg, and SrOH. SrOH is only an intermediate product at high temperature and exists mainly as Sr(OH)2 at ambient temperature and pressure. It may be assumed that the magnesium Na element in the combustion product is in the form of Na2O and the Sr element is in the form of Sr(OH)2. By removing the amount of oxygen contained in Na2O and Sr(OH)2, the remaining oxygen is in the form of MgO; by subtracting the magnesium content of magnesium oxide, the remaining magnesium is unreacted magnesium. Based on the EDS test results, the unreacted magnesium content at different pressures was calculated, as shown in . At atmospheric pressure, both N and S react almost completely due to sufficient oxidation content in the environment. Therefore, the residual Mg content is negligible. With decreasing pressure, the content of unreacted magnesium in the product gradually increased, indicating that the degree of reaction of the sample gradually decreased. For comparison, it was found that the residual Mg content in the combustion product of S was only 8.0% at 1 kPa, while the residual Mg content in the product of N reached 15.7%. It indicates that S has a higher degree of response in a low pressure environment. This result coincides with the test results of thermal analysis.
Table 5. Content of each element in combustion products.
Conclusions
The main objective of this study was to compare the combustion characteristics of Mg/NaNO3 and Mg/Sr(NO3)2 pyrotechnics under low-pressure environments. The effects of ambient pressure on flame structure, burning rate, luminous intensity, and flame spectrum were analyzed by low-pressure combustion experiments. Furthermore, the effect of low pressure on the reaction degree of Mg/NaNO3 and Mg/Sr(NO3)2 pyrotechnics was investigated by low-pressure thermal analysis tests and SEM-EDS tests. We can finally obtain the following conclusions:
At standard atmospheric pressure, the flames spread regularly mainly along the axial direction, and both N and S form converging flames. In the low pressure environment, the weakening of buoyancy causes the gas diffusion rate to accelerate and the combustion flame to be difficult to stabilize. Eventually the N sample forms a divergent flame, and the high temperature combustion zone of the flame shrinks with the decrease of pressure. In contrast, the diffusion rate of the S gas-phase flame is less sensitive to pressure due to the difference in combustion products, and a stable laminar flame can be maintained even in a low-pressure environment. And the high temperature burning zone of its flame is much larger than that of N.
In the range of 1 kPa to 101 kPa, the burning rate of magnesium/nitrate pyrotechnics decreases monotonically with ambient pressure, following Vierle’s law. Since the speed of energy release is directly determined by the burning rate of pyrotechnics, the changing trend of light intensity with pressure is compatible with the burning rate. The luminous intensity versus pressure of Mg/NaNO3 and Mg/Sr(NO3)2 pyrotechnics were obtained by linear regression fitting to satisfy the equations: I = 383.707P0.759 and I = 2239.237P0.385, respectively. Meanwhile, the differences in flame structure and spectral properties lead to better luminescence of Mg/Sr(NO3)2 pyrotechnics in low-pressure environments.
The results of thermal analysis tests and SEM-EDS tests showed that the magnesium/nitrate pyrotechnics with zero oxygen balance could react almost completely at atmospheric pressure. However, as the pressure decreases, the degree of oxidation of magnesium powder gradually decreases along with the oxygen content of air. In addition, the acceleration of the gas diffusion rate at low pressure leads to a decrease in the oxygen content involved in the reaction. Therefore, the coupling effect of gas diffusion and oxygen concentration leads to an increase in the weight loss of the sample at low pressure, a decrease in the enthalpy of reaction and a decrease in the degree of reaction. Nevertheless, the difference in the thermal decomposition properties of sodium nitrate and strontium nitrate results in Mg/Sr(NO3)2 pyrotechnics having a higher degree of reaction under low-pressure environments.
Disclosure statement
No potential conflict of interest was reported by the authors.
References
- Agrawal, J. P. 2010. High Energy Materials: Propellants, Explosives and Pyrotechnics. Weinheim, Germany: Weinheim Wiley-VCH.
- Bagherpour, S., M. Mahdavi, and E. Abedini. 2019. Investigation of thermal behavior of energetic and non-energetic binders on luminous efficiency of high performance miniature flares. Def. Technol. 15 (2):193–97. doi:10.1016/j.dt.2018.09.004.
- Bond, B. D., and P. W. M. Jacobs. 1966. Chemical reaction and ignition in mixtures of magnesium and sodium nitrate. Combust. Flame 10 (4):349–54. doi:10.1016/0010-2180(66)90042-3.
- Conkling, J. A., and C. J. Mocella. 2019. Chemistry of pyrotechnics: basic principles and theory. Boca Raton: FL CRC Press.
- Douda, B. E., R. M. Blunt, and E. J. Bair. 1970. Visible radiation from illuminating-flare flames: strong emission features*†. J. Opt. Soc. Am. 60 (8):8. doi:10.1364/JOSA.60.001116.
- Freeman, E. S. 1956. The kinetics of the thermal decomposition of Sodium Nitrate and of the reaction between Sodium Nitrite and Oxygen. J Phys Chem 60 (11):1487–93. doi:10.1021/j150545a005.
- Guo, Z., J. Ju, H. Guan, C. Shi, and Z. Li. n.d. Study of combustion characteristics of magnesium/Sodium Nitrate Pyrotechnics under sub-atmospheric pressure. Combustion Science and Technology. doi:10.1080/00102202.2022.2111661.
- Johnston, S. F. 2002. A history of light and colour measurement: science in the shadows. Meas. Sci. Technol. 13 (2):230. doi:10.1088/0957-0233/13/2/704.
- Kumar, P., P. C. Joshi, and R. Kumar. 2016. Thermal decomposition and combustion studies of catalyzed AN/KDN based solid propellants. Combustion & Flame 166:316–32. apr. doi: 10.1016/j.combustflame.2016.01.032.
- Kumar, P., M. Varshney, and A. Manash. 2019. Combustion performance studies of aluminum and boron based composite solid propellants in sub-atmospheric pressure regimes. Propuls. Power 8 (4):329–38. doi:10.1016/j.jppr.2019.09.001.
- Li, L., X. Chen, O. Musa, C. Zhou, and M. Zhu. 2018. The effect of pressure and oxygen concentration on the ignition and combustion of aluminum–magnesium fuel-rich propellant. Aerosp Sci Technol 76:394–401. doi:10.1016/j.ast.2017.12.032.
- Pan, G. P. 1996. Advanced pyrotechnics, 14–19Harbin: Harbin Engineering University Press.
- Pouretedal, H. R., and R. Ebadpour. 2014. Application of non-Isothermal Thermogravimetric method to interpret the decomposition kinetics of, and. Int J Thermophys 35 (5):942–51. doi:10.1007/s10765-014-1636-y.
- Pouretedal, H. R., and S. L. Mousavi. 2018. Study of the ratio of fuel to oxidant on the kinetic of ignition reaction of Mg/Ba(NO3)(2) and Mg/Sr(NO3)(2) pyrotechnics by non-isothermal TG/DSC technique. J Therm Anal Calorim 132 (2):1307–15. doi:10.1007/s10973-018-7028-y.
- Pouretedal, H. R., and M. Ravanbod. 2015. Kinetic study of ignition of Mg/NaNO3 pyrotechnic using non-isothermal TG/DSC technique. J Therm Anal Calorim 119 (3):2281–88. doi:10.1007/s10973-014-4330-1.
- Sabatini, J. J., E. -C. Koch, J. C. Poret, J. D. Moretti, and S. M. Harbol. 2015. Chlorine-free red-burning Pyrotechnics. Angew. Chem. Int. Ed. 54 (37):10968–70. doi:10.1002/anie.201505829.
- Sadek, R., M. Kassem, M. Abdo, and S. Elbasuney. 2017. Novel yellow colored flame compositions with superior spectral performance. Def. Technol. 13 (1):33–39. doi:10.1016/j.dt.2016.12.001.
- Singh, H., M. R. Somayajulu, and R. Bhaskara Rao. 1989. A study on combustion behavior of magnesium sodium nitrate binary mixtures. Combust. Flame 76 (1):57–61. doi:10.1016/0010-2180(89)90077-1.
- Tuukkanen, I., S. D. Brown, E. L. Charsley, S. J. Goodall, and H. Lemmetyinen. 2004. Studies on the ageing of a magnesium–strontium nitrate pyrotechnic composition using isothermal microcalorimetry and thermal analysis techniques. Thermochim Acta 417 (2):223–29. doi:10.1016/j.tca.2003.07.021.
- Tuukkanen, I. M., E. L. Charsley, P. G. Laye, J. J. Rooney, T. T. Griffiths, and H. Lemmetyinen. 2006. Pyrotechnic and thermal studies on the magnesium-strontium nitrate pyrotechnic system. Propellants Explosives Pyrotechnics 31 (2):110–15. doi:10.1002/prep.200600012.
- Wagman, D. D. 1982. The NBS tables of chemical thermodynamic properties. J Phys Chem Ref Data 11 (2) .
- Weissler, G. L., and R. W. Carls. 1979. Vacuum physics and technology. USA: Academic Press.
- Woodley, C., R. Claridge, N. Johnson, and A. Jones. 2017. Ignition and combustion of pyrotechnics at low pressures and at temperature extremes. Defence Technology 3 (3): 119–126. doi:10.1016/j.dt.2017.03.004.
- Yan, Q. L., X. J. Li, Y. Wang, W. H. Zhang, and F. Q. Zhao. 2009. Combustion mechanism of double-base propellant containing nitrogen heterocyclic nitroamines (I): the effect of heat and mass transfer to the burning characteristics. Combust. Flame 156 (3):633–41. doi:10.1016/j.combustflame.2008.12.004.
- Yoshida, T. 1995. Safety of Reactive Chemicals and Pyrotechnics. New York: Elsevier.