Abstract
This study investigated the availability of phosphorus (P) following soil application of a novel biosolids-derived organomineral fertilizer (OMF15; 15:4:4) in comparison with single superphosphate (0:18:0). Two soil types of contrasting characteristics were incubated over a period of 90 days at 25 °C and maintained near field capacity. Phosphorus was applied at rates equivalent to 0 (control), 150, and 300 kg ha−1 of P2O5, respectively. Availability of P from OMF15 was low throughout the experiment accounting for less than 6.5% of total OMF15-P applied. It was shown that after the 90 days incubation period, the overall increase in soil extractable P in OMF15-treated soil was marginal in both soil types. For single superphosphate (SSP), P availability ranged from 16% to 46% of total SSP-P applied. Application of SSP increased soil extractable P levels significantly (P < 0.001) compared with unfertilized control soils. The results of this study aided the development of fertilization strategies for the best use of OMF produced from nutrient-enriched biosolids granules for applications in winter cereal and grass crops in England.
Introduction
This article, the second in a series of two, reports the results of a laboratory investigation into the availability of phosphorus (P) following soil application of a novel biosolids-derived organomineral fertilizer (OMF) known as OMF15 (15:4:4) (Antille et al. Citation2013a). The first article (Antille et al. Citation2014a) addresses issues relating to nitrogen (N) availability following soil application of OMF15. The data produced by these two studies aided the development of a set of practical recommendations for the best use of OMF in winter cereal and grass crops in England.
In the United Kingdom, current recommendations for application of organic manures, including biosolids, to agricultural soils (DEFRA Citation2010) are based on the assumption that about 50% of the total phosphate content in the material is available to the next crop grown, with the reminder becoming available over subsequent years. The use of iron (Fe), aluminium (Al), or calcium (Ca) compounds during the tertiary treatment of wastewater enables removal by precipitation of 90% or more of phosphorus (P) present in the sewage sludge (De Haan Citation1980). When this phosphorus is subsequently incorporated into the sludge, its phytoavailability can be significantly reduced (Kokkora et al. Citation2010). The phytoavailability of applied biosolids-P is controlled by the interaction of numerous factors, which include environmental, crop, and soil conditions, microbial activity, and the characteristics of the native and added P sources (Elliot et al. Citation2002a; O’Connor et al. Citation2004; Smith et al. Citation2006). Soil properties such as pH (Kirk Citation2002), and concentration of ions such as Fe, Al, and Ca affect both chemical mobility and bioavailability (Hinsinger Citation2001). Routine application of biosolids can lead to build-up of soil P, a problem that has been observed in areas close to production sites that frequently exhibit relatively high soil P Indexes (Antille et al. Citation2013a; Citation2013b), that is, above the recommended target Index for the soil-crop system (DEFRA Citation2010). This occurs because of the combined effect of relatively low N:P ratios of biosolids (typically less than 1) with soil applications based entirely on N requirements by crops, which results in over-application of P relative to crop requirements. Surplus of phosphorus in soils receiving periodic applications of biosolids influences soil P dynamics and can enhance transport of P to water courses when the soil sorption capacity is exceeded (Smith et al. Citation2006; Withers et al. Citation2009). Therefore, understanding the availability of P from organic-based fertilizers applied to soil is an important agronomic and environmental consideration for nutrient management. This requires matching the timing of phosphorus applications with crop demand to enhance P uptake by crops, replenish off-take, and reduce unnecessary build-up of soil P (Oladeji et al. Citation2008). Antille et al. (Citation2013a) emphasized that OMF15 may be applied to soils that have satisfactory soil P status, as specified in current agronomic guidelines (DEFRA Citation2010), but provided crop requirements for P are not exceeded. This criterion for P fertilization is supported by the need to ensure the maintenance of sufficient nutrient reserves in the soil (Dawson Citation2011) and due to the associated effects (interaction) of soil P availability on the use efficiency of other nutrients, notably, nitrogen (Mouat and Nes Citation1983).
Recycling of biosolids to land will help to tackle the challenge of finite resources of phosphorus that are under pressure from an expanding world population that needs feeding. There are various estimates of global phosphate reserves (e.g., Steén Citation1998); however, the true figure is largely unknown. This is partly because of the sensitive nature of the information and partly because there may be phosphate rock yet to be discovered (Cordell et al. Citation2009; Hilton et al. Citation2010).
The present study aims to provide robust quantitative data to describe the soil behaviour and determine the fertilizer value of phosphorus present in OMF15. Whilst acknowledging the need to investigate soil P dynamics in-field conditions, the data compiled in this article will provide mechanisms which will contribute to fill a critical gap in the knowledge base for the efficient utilization of nutrients in organic-based fertilizer materials applied to soil in crop production. The proposed methodological approach comprises a simple yet, effective, laboratory experiment which will aid the interpretation of findings derived from further studies by the authors at the glasshouse and field-scale levels (Antille et al. Citation2013b; Citation2014b). The objectives of this study were to: (1) determine phosphorus availability from OMF15 (15:4:4) following application to the soil under controlled laboratory conditions of temperature and soil moisture content, and (2) determine changes in soil P status under the same experimental conditions. The study was conducted by comparing the release characteristics and soil effects of OMF15-P with single superphosphate-P (0:18:0). It was hypothesized that soil application of OMF15-P, at the rates used in this study, would not change soil P Index (DEFRA Citation2010) significantly despite that the work was conducted under controlled laboratory conditions and in the absence of crops.
Materials and Methods
Description of the Soils
The experiment used two soil types described in King (Citation1969) as Cottenham series sandy loam and Holdenby series clay loam (). They were selected to have soils with contrasting physical and chemical characteristics for the incubation study and because of their frequent occurrence in the NW region of England (Ragg et al. Citation1984), which was the area of interest for this study. The soils were collected from the farm at Cranfield University (Silsoe, England); they had been under winter cereal and oilseed rape cropping (Antille Citation2011) and subjected to standard fertilization regimes (DEFRA Citation2010). The following determinations were conducted in soil samples taken from the farm prior to the start of the experiment: soil textural analysis (BS 1377 Section 2.0 Citation1990), soil moisture content corresponding to field capacity at 0.05 bar (BS 7755 Section 5.5 Citation1999), and soil bulk density (Blake and Hartge Citation1986), which was required to determine quantities of fertilizer added to incubation pots.
Table 1 Soil textural analysis, soil moisture content corresponding to field capacity (mass basis) and soil bulk density for the two soils used in the incubation studies (Sampling depth: 200 mm)
Fertilizer Materials
The availability of phosphorus (P) in OMF15 (15:4:4) was compared with that of single superphosphate (0:18:0) following fertilizer application to the soil. The fertilizers were applied at rates equivalent to 0 (control), 150 and 300 kg ha−1 of phosphorus pentoxide (P2O5) to provide a suitable range that would cover P-fertilization regimes recommended for arable and forage crops in the entire spectrum of soil P Indexes (DEFRA Citation2010). All treatments, including controls, were replicated four times (n = 4). The actual fertilizer quantities applied to individual pots are shown in . The differences in the amount of fertilizer applied to the clay loam and the sandy loam soils are due to the values of soil bulk density encountered in each of the two soil types (). Phosphorus converts to P2O5 by multiplying by 2.291 (DEFRA Citation2010).
Table 2 Fertilizer added to incubation pots for the study of P availability from OMF15 (15:4:4) and single superphosphate (SSP, 0:18:0)
Soil Incubation
Incubation pots of 0.25 litres capacity were filled with 200 g of air-dried soil previously ground to pass a 2 mm sieve, mixed with fertilizer and wetted-up with de-ionized water until the soil reached field capacity. The pots were placed in an incubator in the absence of light and the temperature in the chamber adjusted to 25 ± 0.1 °C (Hogan et al. Citation2001). The temperature in the chamber was selected to be consistent with that used for the study of nitrogen, which is reported in the first article of this series (Antille et al. Citation2014a). Soil moisture content was regularly replenished by adding de-ionized water on a mass basis (Smernik et al. Citation2004), and the soil maintained between field capacity (FC) and 75% of FC throughout the experiment. The study was conducted for a period of 90 days following the guidelines outlined in Organization for Economic Co-operation and Development (OECD; OECD Citation2002). Conversion of analytical values from mg kg−1 to kg ha−1 (field equivalent) was done based on the corresponding soil bulk density and assuming a depth of 200 mm ().
Measurements and Analyses
Soil sampling was conducted at the start of the experiment (before fertilizer application), and every 30 days thereafter. The sampling technique was non-destructive (Johnston Citation2008) as the same pots and soil were used throughout the experiment over the 90 days period. The following analyses were conducted prior to fertilizer application: total carbon (C) (BS 7755 Section 3.8 Citation1995), total P (BS 7755 Section 3.13 Citation1998), soil pH (MAFF Citation1986, Method No.: 32), and soil organic matter (SOM) (MAFF Citation1986, Method No.: 56). Soil extractable P (Olsen et al. Citation1954; BS 7755 Section 3.6 Citation1995) was determined prior to fertilizer application and every 30 days thereafter. Soil P Index was based on DEFRA (Citation2010) according to values of soil extractable P reported in the analyses. Results are reported in the form of (mean) soil extractable P recorded at times corresponding to sampling events at 0, 30, 60, and 90 days, respectively. The values of soil extractable P, as reported from the soil laboratory, needed conversion from (w v−1) to (w w−1), which was done based on Johnston (Citation1975). The percentage P available () was estimated with Equation (1) relative to total P applied (Leytem et al. Citation2004) at days 30, 60 and 90, respectively.
Where: is net soil extractable phosphorus determined at 30, 60, and 90 days after fertilizer application.
Statistical Analyses
Statistical analyses were undertaken using GenStat Release 10.1 (Citation2007) by applying repeated measurement of analysis of variance (ANOVA) and least significant differences (LSD) to compare the means using a 5% probability level (P < 0.05). The analyses conducted were graphically verified by means of residual plots. Normalization of the data was not required.
Results and Discussion
Initial Soil Analyses
shows the results of the soil chemical analyses conducted prior to the start of the experiment, which corresponded to the baseline level.
Table 3 Soil analyses conducted prior to the start of the experiment in the laboratory. The standard deviation (SD) is shown as ± the mean value, except when n=1
Phosphorus Release Characteristics
shows soil extractable P recorded at 0, 30, 60, and 90 days from the start of the experiment. Overall, fertilizer-treated soils showed higher (P < 0.001) extractable P levels compared with unfertilized control soils. There were significant differences (P < 0.001) in soil extractable P between the two soil types, which were due to overall higher fertility status of the clay loam compared with the sandy loam soil (). Fertilizer type and rate showed significant effects (P-values <0.001) on soil extractable P levels. The fertilizer type effect was mainly due to the use of single superphosphate (SSP) since increases in soil extractable P levels observed in OMF15-treated soils were marginal compared with controls, and they appeared to be nonsignificant based on the calculated LSD values. Mean values of soil extractable P across both soil types and fertilizer application rates were: 79.6, 80.1, and 88.6 mg kg−1 for control, OMF15- and SSP-treated soils, respectively (LSD 5% level: 1.3 mg kg−1 for comparisons between control and treatments, and 1.1 mg kg−1 for comparisons between treatments). Application of SSP increased (P < 0.001) soil extractable P after the 90 days incubation period compared with controls. The majority of that phosphorus was released within the first 30 days following application.
Figure 1. Soil extractable P recorded at days 0, 30, 60 and 90 after fertiliser application to clay loam (top) and sandy loam (bottom) soils, respectively (p = 0.70, n = 4). OMF is OMF15 (15:4:4) and SSP is single superphosphate (0:18:0), followed by the corresponding phosphorus application rate expressed in kg per ha of P2O5.
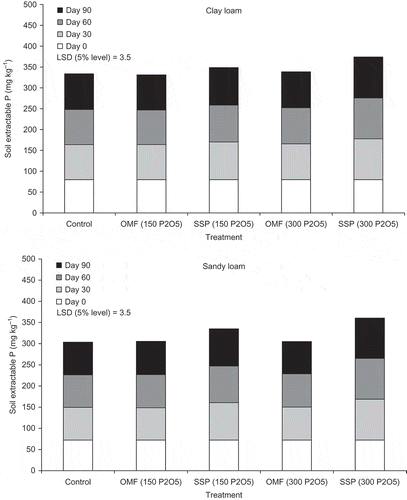
The values of soil extractable P recorded in OMF15-treated soils reflect the relatively low solubility of inorganic-P fractions present in biosolids granules. This responds to the chemical removal of P from wastewater effluents during the tertiary treatment, which is conducted by precipitation with FeCl3, and subsequent incorporation of P into the sludge (Kokkora et al. Citation2010; Antille Citation2011). This process produces Fe-phosphates, which are largely unavailable for plant up-take, effect that had been indicated in earlier studies (e.g., Hogan et al. Citation2001; Maguire et al. Citation2001; O’Connor et al. Citation2004). These phosphorus fractions cannot be detected in routine soil analyses (e.g., Olsen et al. Citation1954); hence, application of OMF15-P to the soil did not show changes in soil extractable P levels. Chemical analysis performed in samples of biosolids granules used for production of OMF (Antille et al. Citation2013a) showed that the concentration of water soluble-P (BS 7755 Section 3.6 Citation1995) was low and rarely exceeding 0.25% (by weight), which confirms that the majority of P in OMF15 is therefore not readily available.
The net availability of P following soil application of biosolids is controlled by chemisorption and precipitation reactions of P, and mineralization of organic-P fractions present in the material (Taylor et al. Citation1978). Following soil application of P containing fertilizers, phosphorus is dissolved in the soil-water; however a proportion of that P is temporarily immobilized undergoing the type of reactions indicated above, which occur with the various soil constituents (Morgan Citation1997). Subsequently, the availability to crops is governed by release of phosphorus from these reaction products to the soil solution (Morgan Citation1997), which is highly dependent on soil pH (Kirk Citation2002). The process described above, which controls the release phosphorus to the soil solution, was noticeable following application of SSP and resulted in some immobilization of P applied as fertilizer. This is supported by the fact that not all the phosphorus added as fertilizer was subsequently detected in soil analyses, despite that SSP contains between 92% and 94% water soluble P (Charleston Citation1984). The effect was more evident in clay loam compared with sandy loam soil based on the calculated values of available P, relative to total P added as fertilizer. Phosphorus availabilities in SSP-treated soils ranged between 15% and 25% in clay loam soil, and between 37% and 46% in sandy loam soil, respectively ().
Table 4 Phosphorus available in the soil as percentage of the total P applied with the fertiliser for soils treated with OMF15 (15:4:4) and single superphosphate (SSP, 0:18:0). Use n = 4
The application of fertilizer to the soil appeared to have altered the balance between the various soil phosphorus pools (Johnston and Syers Citation2006) since phosphorus availability, expressed in percentage of total P added as fertilizer, tended to decrease with time during the 90 days incubation period (). In the absence of plant uptake, the excess of phosphorus in the soil solution, which resulted from the addition of fertilizer, moved towards less readily available pools and therefore the percentage available P detected at 90 days was relatively lower compared with values measured at 30 days. Johnston and Syers (Citation2006) suggested that availability of P in the soil could be explained by the concept of ‘P equilibrium’ which involves adsorption and desorption reactions that are reversible with time. Therefore, it is suggested that application of fertilizer phosphorus induced a significant increase in the concentration of phosphorus in the soil solution (the pool that contains the readily available fraction), which occurred to a much greater extent in soil treated with SSP compared with OMF15. Subsequently, the balance between pools tended to be restored and therefore a proportion of phosphorus in the soil solution moved to less readily available pools, hence, yielding relatively lower values of extractable P in soil analysis. This occurred because the concentration of P in the soil solution is primarily driven by plant uptake (Johnston and Syers Citation2006); however in the absence of this process, phosphorus added as fertilizer moves to less readily available pools.
Johnston and Syers (Citation2006) discarded earlier views, which sustained that phosphorus applied with fertilizers could be irreversibly fixed in the soil. On the contrary, they suggest that P is present in the soil in constant equilibrium between various pools in which soil P is retained by a continuum of bonding energies. Their work highlighted that this concept contributed to developing the idea of applying phosphorus to ensure that a critical level in soil can be maintained, which is dependent on the soil type and the soil-crop system. The proposed formulations for OMF (Antille et al. Citation2013a) were, to a large extent, based on this principle so that P applied to the soil as fertilizer would replenish, but not exceed, off-take by crops and therefore would minimize the risk of build-up of P (DEFRA Citation2010).
Replenishment of P to the soil solution occurs through mechanisms involving dissolution and desorption reactions, which include reaction products from recent phosphorus applied with fertilizers as well as phosphorus compounds from previous fertilizer applications (Morgan Citation1997). Phosphorus release from OMF15 may be sustained for several years following application in-field conditions. Wise (Citation1999) suggested that the use of biosolids is better justified to meet crop requirements for P of the entire crop rotation and not for individual years providing soil-P status was already satisfactory. This is supported by the expected long-term release of phosphorus from biosolids. Lu and O’Connor (Citation2001) concluded that phosphorus present in Fe- or Al-rich biosolids will behave as slowly available P fertilizers, and consequently less prone to leaching losses compared with more soluble P-containing fertilizer materials. Siddique et al. (Citation2000) indicated that the relatively faster rate of P saturation and P leaching in monocalcium-treated soil compared with sludge-treated soil reflect how P sources influence the velocity of adsorption–desorption reactions. With respect to residual effect of P in fertilizers and sewage sludge, research (e.g., Kamprath Citation1967; Kelling et al. Citation1977; Morgan Citation1997) showed that following soil application, phosphorus can be utilized by crops in subsequent seasons, e.g., up 8 to 10 years post-application, that is, in the absence of exports or losses of that phosphorus by mechanisms such as transport in overland flow (e.g., McDowell and Sharpley Citation2002; Elliot et al. Citation2005) or leaching (e.g., Siddique et al. Citation2000; Elliot et al. Citation2002b). shows the availability of P in the soil relative to total P applied as fertilizer based on Equation (1).
Application of OMF15 to the soil resulted in relatively low percentages of available P relative to total OMF15-P applied, which was observed in both soils. This finding contrast with current recommendations for application of thermally dried biosolids to agricultural soils (DEFRA Citation2010), which assumes 50% phosphate (P2O5) availability relative to total P2O5 applied for all soil types. The results in also reflect a textural effect on SSP-P availability relative to total SSP-P applied on sandy loam soil. Availability of OMF15-P relative to that of SSP-P, or relative P extractability (Leytem et al. Citation2004), ranged approximately between 2.5% and 12% on average over the 90 days incubation period, except when OMF15-P was applied at the lowest rate on clay loam soil, which yielded negative values ().
O’Connor et al. (Citation2004) indicated that biosolids that had undergone biological P removal but that had been supplemented with Fe and Al usually show low phytoavailability, typically lower than 25% relative to that of triple superphosphate (0:46:0), which appears to be in agreement with the results shown in for OMF15-P. Hogan et al. (Citation2001), however, highlighted that Fe-rich biosolids are potentially effective fertilizers because following application Fe-phosphates react with soil preferentially forming Fe-hydroxides and consequently releasing P in phytoavailable forms. Despite this, if biosolids had undergone thermal treatment, the reactivity of Fe-bound P minerals in the material is reduced which reduces their interaction with soil-Ca and restricts the release of phytoavailable P (Hogan et al. Citation2001). This process helps explain the results reported in this study as P was removed by precipitation with iron cloride (FeCl3) and biosolids had undergone thermal treatment. Given the relatively slow rates of P release encountered, there is a need to study residual effects of applied OMF15-P in-field conditions so that it can be accounted for as part of the fertilization plan of the entire crop rotation rather than for individual years.
Conclusions
Phosphorus availability from OMF15 was low throughout the experiment, accounting for less than 6.5% (by weight) relative to total P applied as fertilizer, and between 2.5% and 12% relative to that of single superphosphate P. The relatively low availability of OMF15-P is attributed to the technique used for removal of P during the wastewater treatment process. The changes recorded in soil extractable P remained close to initial levels, which agrees with earlier work in that phosphorus in biosolids containing iron or aluminium compounds behave as slowly available P sources, especially when the sludge has undergone thermal treatment.
For single superphosphate, P availability ranged between 16% and 46% depending mainly on soil type (textural effect), and to a lesser extent on phosphorus application rate, and there was a significant increase in soil extractable P levels, consequently soil P Index, following application. Phosphorus release from OMF15 was less dependent on soil texture compared with single superphosphate. This work suggests that due to slow availability of P from OMF15, there is minimal risk of P leaching.
Based on the results of this study, and those reported by the authors on OMF15-N (Antille et al. Citation2014a), there appears to be potential for further development of biosolids-derived organomineral fertilizer products. The timing of OMF application requires investigation at the field-scale to optimise nutrient use efficiency and reduce the risk of nutrient losses to the environment.
Acknowledgments
Comments and suggestions made by A.E. Johnston (Rothamsted Research, Harpenden, UK) and P. Bellamy (Cranfield University, Cranfield, UK) are appreciated.
Funding
This research received funding from the European Union Seventh Framework Programme (FP7-ENV.2010.3.1.1-2 ENV) under grant agreement no.: 265269 (http://www.end-o-sludg.eu/). The article represents the opinion of the authors and does not necessarily represent the view of the European Union or United Utilities Group PLC. The authors are grateful to United Utilities Group PLC (Warrington, UK), The Engineering and Physical Science Research Council (Swindon, UK), and Cranfield University (Cranfield, UK) for financial and operational support to conduct this research.
Additional information
Funding
References
- Antille, D.L. 2011. Formulation, utilisation and evaluation of organomineral fertilisers. Engineering Doctorate Thesis, Cranfield University, UK, 572 pp.
- Antille, D.L., R. Sakrabani, S.F. Tyrrel, M.S. Le, and R.J. Godwin. 2013a. Characterisation of organomineral fertilisers derived from nutrient-enriched biosolids granules. Applied and Environmental Soil Science, Vol. 2013, Article ID 694597, 11 pp., http://dx.doi.org/10.1155/2013/694597.
- Antille, D.L., R. Sakrabani, and R.J. Godwin. 2013b. Field-scale evaluation of biosolids-derived organomineral fertilisers applied to ryegrass (Lolium perenne L) in England. Applied and Environmental Soil Science, Vol. 2013, Article ID 960629, 10 pp., http://dx.doi.org/10.1155/2013/960629.
- Antille, D.L., R. Sakrabani, and R.J. Godwin. 2014a. Nitrogen release characteristics from biosolids-derived organomineral fertilizers. Communications in Soil Science and Plant Analysis 45(12):1687–1698.
- Antille, D.L., R. Sakrabani, and R.J. Godwin. 2014b. Effects of biosolids-derived organomineral fertilizers, urea, and biosolids granules on crop and soil established with ryegrass (Lolium perenne L.). Communications in Soil Science and Plant Analysis 45(12):1605–1621.
- Blake, G.R., and K.H. Hartge. 1986. Bulk density. In Methods of soil analysis. Part 1: Physical and mineralogical methods. 2nd Edition. A. Klute ( ed.). Agronomy 9:363–382. Madison, WI: American Society of Agronomy (ASA).
- British Standard 1377 Section 2.0. 1990. Sedimentation by pipette method. London: The British Standards Institution.
- British Standard 7755 Section 3.13. 1998. Soil quality. Chemical methods. Determination of cadmium, chromium, cobalt, copper, lead, manganese, nickel and zinc in aqua regia extracts of soil. Flame and electrothermal atomic absorption spectrometric methods. Equivalent to ISO 11047:1998. London: The British Standards Institution.
- British Standard 7755 Section 3.6. 1995. Determination of phosphorus. Spectrometric determination of phosphorus soluble in sodium hydrogen carbonate solution. Equivalent to ISO 11263:1994. London: The British Standards Institution.
- British Standard 7755 Section 3.8. 1995. Soil quality. Chemical methods. Determination of organic and total carbon after dry combustion (elementary analysis). Equivalent to ISO 10694:1995. London: The British Standards Institution.
- British Standard 7755 Section 5.5. 1999. Determination of water retention characteristics: Laboratory methods. Equivalent to ISO 11274:1998. London: The British Standards Institution.
- Charleston, A.G. 1984. Solubilities of single superphosphate components in water, 2% citric acid, and neutral ammonium citrate solution. New Zealand Journal of Science 27(3):269–277.
- Cordell, D., J.O. Drangert, and S. White. 2009. The story of phosphorus: global food security and food for thought. Global Environmental Change 19(2):292–305.
- Dawson, C.J. 2011. Phosphate and potash—reconsidering their importance and use. Journal of the Royal Agricultural Society of England 172:1–9.
- DEFRA. 2010. Department for Environment, Food and Rural Affairs. 2010. Fertiliser manual. Reference book 209. 8th Edition. London: The Stationery Office.
- De Haan, S. 1980. Sewage sludge as a phosphate fertilizer. Phosphorus in Agriculture 78:33–41.
- Elliot, H.A., R.C. Brandt, and G.A. O’Connor. 2005. Runoff phosphorus losses from surface-applied biosolids. Journal of Environmental Quality 34(5):1632–1639.
- Elliot, H.A., G.A. O’Connor, P. Lu, and S. Brinton. 2002a. Influence of water treatment residuals on phosphorus solubility and leaching. Journal of Environmental Quality 31(4):1362–1369.
- Elliot, H.A., G.A. O’Connor, and S. Brinton. 2002b. Phosphorus leaching from biosolids-amended sandy soils. Journal of Environmental Quality 31(2):681–689.
- GenStat 2007. GenStat Release 10.1 (10th Edition). Hemel Hempstead, VSN International, UK.
- Hilton, J., A.E. Johnston, and C.J. Dawson. 2010. The phosphate life-cycle: rethinking the options for a finite resource. Proceeding No.: 668, York, UK: The International Fertiliser Society.
- Hinsinger, P. 2001. Bioavailability of soil inorganic P in the rhizosphere as affected by root-induced chemical changes: a review. Plant and Soil 237(2):173–195.
- Hogan, F., M. McHugh, and S. Morton. 2001. Phosphorus availability for beneficial use in biosolids products. Environmental Technology 22(11):1347–1353.
- Johnston, A.E. 1975. The Woburn Market Garden Experiment, 1942-69. II: Effects of the treatments on soil pH, soil carbon, nitrogen, phosphorus and potassium. Rothamsted Experimental Station Report for 1974 Part 2, 102–131 pp. Harpenden, UK: Rothamsted Research.
- Johnston, A.E. 2008. Personal communication. Harpenden, UK: Rothamsted Research.
- Johnston, A.E., and J.K. Syers. 2006. Changes in understanding the behaviour of soil and fertiliser phosphorus: implications for their efficient use in agriculture. Proc. No.: 589. York, UK: The International Fertiliser Society.
- Kamprath, E.J. 1967. Residual effect of large applications of phosphorus on high phosphorus fixing soils. Agronomy Journal 59(1):25–27.
- Kelling, K.A., L.M. Walsh, D.R. Keeney, J.A. Ryan, and A.E. Paterson. 1977. A field study of the agricultural use of sewage sludge: II. Effect on soil N and P. Journal of Environmental Quality 6(4):345–352.
- King, D.W. 1969. Soils of the Luton and Bedford Districts: a reconnaissance survey. Harpenden, UK: The Soil Survey of England and Wales.
- Kirk, G.J.D. 2002. Use of modelling to understand nutrient acquisition by plants. Plant and Soil 247(1):123–130.
- Kokkora, M.I., D.L. Antille, and S.F. Tyrrel. 2010. Considerations for recycling of compost and biosolids in agricultural soil. Chapter 13. In: Dedousis, A.P., and T. Bartzanas ( Eds). 2010.Soil Engineering (Soil Biology Series) 20(3):195–215. Berlin Heidelberg: Springer-Verlag.
- Leytem, A.B., J.T. Sims, J.T., and F.J. Coale. 2004. Determination of phosphorus source coefficients for organic phosphorus sources: laboratory studies. Journal of Environmental Quality 33(1):380–388.
- Lu, P., and G.A. O’Connor. 2001. Biosolids effects on phosphorous retention and release in some sandy Florida soils. Journal of Environmental Quality 30(3):1059–1063.
- MAFF. 1986. Ministry of Agriculture, Fisheries and Food. 1986. The analysis of agricultural materials, 3rd Edition Reference Book 427: Methods 32 and 56. London: The Stationery Office.
- Maguire, R.O., J.T. Sims, S.K. Dentel, F.J. Coale, and J.T. Mah. 2001. Relationships between biosolids treatment process and soil phosphorus availability. Journal of Environmental Quality 30:(3)1023–1033.
- McDowell, R., and A. Sharpley. 2002. Phosphorus transport in overland flow in response to position of manure application. Journal of Environmental Quality 31(1):217–227.
- Morgan, M.A. 1997. The behaviour of soil and fertiliser phosphorus. Chapter 6. In: Tunney, H., O.T. Carton, P.C. Brookes, and A.E. Johnston ( Eds). 1997. Phosphorus loss from soil to water. Wallingford, UK: CAB International.
- Mouat, M.C.H., and P. Nes. 1983. Effect of the interaction of nitrogen and phosphorus on the growth of ryegrass. New Zealand Journal of Agricultural Research 26(3):333–336.
- OECD. 2002. Organization for Economic Co-operation and Development. 2002. OECD guidelines for the testing of chemicals. Section 3: Degradation and accumulation. Test No.: 307. Aerobic and anaerobic transformations in soil. 1-17 pp. Acc. 30 April 2009. http://puck.sourceoecd.org/vl=1597167/cl=26/nw=1/rpsv/cw/vhosts/oecdjournals/1607316x/v1n3/contp1-1.htm.
- O’Connor, G.A., D. Sarkar, S.R. Brinton, H.A. Elliot, and F.G. Martin. 2004. Phytoavailability of biosolids phosphorus. Journal of Environmental Quality 33(2):703–712.
- Oladeji, O.O., G.A. O’Connor, and J.B. Sartain. 2008. Relative phosphorus phytoavailability of different phosphorus sources. Communications in Soil Science and Plant Analysis 39(15–16):2398–2410.
- Olsen, S.R., C.V. Cole, F.S. Watanabe, and L.A. Dean. 1954. Estimation of available phosphorus in soils by extraction with sodium bicarbonate. USDA Circular No.: 939.
- Ragg, J.M., G.R. Beard, H. George, F.W. Heaven, J.M. Hollis, R.J.A. Jones, R.C. Palmer, M.J. Reeve, J.D. Robson, and W.A. Whitfield. 1984. Soils and their use in Midland and Western England. Bulletin No.: 12. Harpenden, UK: The Soil Survey of England and Wales.
- Siddique, M.T., J.S. Robinson, and B.J. Alloway. 2000. Phosphorus reactions and leaching potential in soils amended with sewage sludge. Journal of Environmental Quality 29(6):1931–1938.
- Smernik, R.J., I.W. Oliver, and M.J. McLaughlin. 2004. Changes in the nature of sewage sludge organic matter during a twenty-one-month incubation. Journal of Environmental Quality 33(5):1924–1929.
- Smith, M.T.E., B.J. Cade-Menun, and M. Tibbett. 2006. Soil phosphorus dynamics and phytoavailability from sewage sludge at different stages in a treatment stream. Biology and Fertility of Soils 42(3):186–197.
- Steén, I. 1998. Phosphorus availability in the 21st century: management of a non-renewable resource. Phosphorus and Potassium 217: 25–31.
- Taylor, J.M., L.J. Sikora, C.F. Tester, and J.F. Parr. 1978. Decomposition of sewage sludge compost in soil: II. Phosphorus and sulphur transformations. Journal of Environmental Quality 7(1):119–123.
- Wise, S.W. 1999. A systems approach to the recycling of wastewater sludge phosphorus in agriculture. Engineering Doctorate Thesis, Cranfield University Silsoe, UK.
- Withers, P.J.A., H. Hartikainen, E. Barberis, N.J. Flynn, and G.P. Warren. 2009. The effect of soil phosphorus on particulate phosphorus in land runoff. European Journal of Soil Science 60(6): 994–1004.