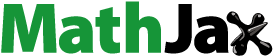
Abstract
Polycrystalline bulk samples of δ-phase Hf hydrides with various Zr contents were prepared and their high-temperature stability and thermal and mechanical properties were investigated. The phase structure was examined between room temperature and 973 K using high-temperature X-ray diffraction and thermogravimetric–differential thermal analysis. From room temperature to 673 K, the coefficient of linear thermal expansion, specific heat capacity, and thermal conductivity were evaluated. The Vickers hardness and sound velocity were measured at room temperature, and the elastic modulus was evaluated. The effect of the Zr content on the high-temperature stability and the thermal and mechanical properties of Hf hydrides was studied.
1. Introduction
In recent years, development of fast reactors has been underway as a means of achieving a stable long-term energy supply and reducing the burden on the environment. As a neutron-absorbing material in such reactors, hafnium (Hf) hydride has been investigated [Citation1,2], since it is capable of moderating fast neutrons. Since the hydrogen atom density in the hydride is comparable to that in water, a comparable neutron moderation capability can be expected. For this reason, if Hf is converted to a hydride which has a large capture cross-section for thermal neutrons, fast neutrons can be moderated and absorbed, making it suitable for control rods in fast reactors. However, because such rods must operate in the high-temperature environment of a fast reactor core, it is necessary to understand the high-temperature phase structure and stability of Hf hydride. One factor that must be considered is that Hf and zirconium (Zr) occur together in nature. Since both elements belong to group IV in the periodic table and have similar chemical properties, their complete separation is extremely difficult. Generally, a small amount of Hf or Zr is contained in widely available Zr or Hf, respectively. In components such as the cladding tubes of light-water reactors, reactor-grade Zr is used, in which the Hf content is reduced to the limit. However, the purification procedure involved is expensive. Therefore, in the case of Hf hydride, if the Zr impurities have little effect on its high-temperature stability or material properties, less expensive low-grade Hf can be used for control rods, although neutron economy must also be considered. However, there have been no reports concerning the characteristics of Zr–Hf alloy hydrides, and their phase structure and material properties are not yet understood. In addition, in both the Zr–H and Hf–H binary phase diagrams [Citation3], the phase boundaries at high temperatures around 1000 K of δ-phase hydrides are unclear and thereby it is difficult to understand the effect of Zr content on the high-temperature stability of δ-phase Hf hydride.
For this reason, the present study aimed at clarifying the effects of the Zr content of Hf hydride on its high-temperature stability and thermal and mechanical properties. Hf hydrides with various Zr contents (Zr-containing Hf hydrides) were prepared, and the phase structure and microstructure were observed. Here, the metal compositions are set to be Zr0.0175Hf0.9825, Zr0.045Hf0.955, and Zr0.10Hf0.90. Zr0.0175Hf0.9825 and Zr0.045Hf0.955 are available as high-purity and moderate-grade products, respectively. Therefore, we chose first Zr0.0175Hf0.9825 and Zr0.045Hf0.955 as the starting compositions. In addition, to systematically understand the effect of Zr on the properties of Hf hydrides and to observe the effect more clearly, Zr0.10Hf0.90 was chosen as the third composition. The high-temperature stability of the materials was evaluated by observing the phase changes that occurred as the temperature was increased and the thermal behavior. In addition, basic thermal and mechanical properties such as the average coefficient of linear thermal expansion, specific heat, thermal conductivity, hardness, and Young's modulus were evaluated. The results obtained allowed the effects of the Zr content on the high-temperature stability and thermal and mechanical properties of Hf hydride to be clarified.
2. Experimental
Zr chunks (purity 99.998%, Furuuchi Chemical Corporation) and Hf chunks (purity 98.250%, Furuuchi Chemical Corporation) were used as the starting materials. Zr-containing Hf alloys were prepared under an argon atmosphere (0.04 MPa) by arc melting. Three kinds of alloy compositions were used: Zr0.0175Hf0.9825, which was obtained by arc melting only Hf chunks; Zr0.045Hf0.955, which is widely available at a moderate price; and Zr0.10Hf0.90, which contains more Zr than the generally available product. In order to make each sample completely uniform, the sample was turned over and melted at least five times in the arc-melting chamber. The resulting alloy samples were formed into shapes suitable for measurement by cutting with a diamond cutter and subsequent polishing. The alloy samples were then hydrogenated using a modified ultra-high-vacuum Sieverts apparatus. The hydrogenation conditions were adjusted so that the H/M (M = Hf + Zr) ratio, which represents the hydrogen content in the hydride, was about 1.6.
Sample preparation was carried out in the following manner. In order to soak the Zr-containing Hf alloys, the material was inserted into a quartz tube, around which a molybdenum foil was rolled, sealed in the furnace, and the air pressure was reduced to 10−6 Pa. Subsequently, the temperature was increased to 1173 K at 3 K/min, and the sample was homogenized by annealing for about 72 h. The temperature was then lowered to 1073 K at −1.7 K/min. The temperature was held at 1073 K for 168 h in an ultra-high-purity hydrogen (7N) flow, the purity of which was increased by passing it through a liquid nitrogen trap. Subsequently, the temperature was lowered to room temperature at −0.3 K/min, and the sample was removed. This procedure allowed bulk Zr-containing Hf hydrides without cracks or chips to be produced by adjusting various conditions during a series of hydrogenation reactions.
The phase structure and lattice constants of the as-prepared Zr-containing Hf hydrides were investigated at room temperature using powder X-ray diffraction (XRD). The Cu-Kα1 line (λ = 1.54056 Å) was used as the X-ray source, and the measurements were carried out in the range of 2θ = 20°–120°. Surface observations were performed using scanning electron microscopy/energy dispersive X-ray spectroscopy (SEM/EDX) in a vacuum at room temperature. For the SEM/EDX analysis, bulk hydride samples were used. The sample density was calculated from the weight and dimensions. The H/M ratio, representing the hydrogen content in the sample, was calculated from the weight change before and after hydrogenation. It was also evaluated from the measured pressure change in the modified Sieverts apparatus. The hydrogen contents determined using both methods were found to be in good agreement with each other.
Thermogravimetric–differential thermal analysis (TG-DTA) was carried out in an inert argon gas flow or in an air atmosphere. The measurements were performed on small pieces of Zr-containing Hf hydride with weights of 48.3–84.9 mg while increasing the temperature from room temperature to 1073 K at 20 K/min. High-temperature XRD analysis of powder samples was carried out in a helium gas flow or in air. The measurements were performed at 100 K steps from room temperature to 973 K. The rate of temperature increase and the holding time at each temperature were 20 K/min and 7.5 min, respectively. In order to evaluate the coefficient of linear thermal expansion, an additional set of high-temperature powder XRD measurements were carried out in a helium gas flow at steps of 50 K from room temperature to 673 K. The rate of temperature increase and the holding time at each temperature were 20 K/min and 7.5 min, respectively. From the resulting XRD patterns, the lattice constant at each temperature was determined, and the coefficient of linear thermal expansion was evaluated. The specific heat capacity was measured using differential scanning calorimetry. Sapphire was used as the standard material, and the measurements were carried out from room temperature to 673 K in an argon gas flow. The thermal diffusivity was measured from room temperature to 673 K under vacuum using the laser flash method. The thermal conductivity κ was then calculated based on the relationship κ = α × Cp × ρ, from the thermal diffusivity α, the specific heat capacity Cp, and the density ρ. The Vickers hardness was measured using a Vickers hardness tester. The measurements were carried out 10 times or more for a load of 1 kgf and a holding time of 10 s, and the average value was determined. Using an ultrasonic pulse-echo method, the velocity of longitudinal sound waves through the sample was measured. The velocity of transverse sound waves could not be directly measured. The reported value of Poisson's ratio (ν) for δ-HfH1.62 is 0.35 [Citation4]. On the other hand, the Poisson's ratio for δ-Zr hydride is reported to be 0.32, regardless of the hydrogen content [Citation5], and this value is about the same as that for δ-Hf hydride. Therefore, the reported value of 0.35 for Hf hydride was used for the Zr-containing Hf hydrides in this study [Citation4]. Based on the Poisson's ratio and the longitudinal sound wave velocity, the velocity of transverse sound waves was calculated, which allowed Young's modulus to be determined.
3. Results and discussion
3.1. Identification of Zr-containing Hf hydrides
shows XRD patterns for the as-prepared Zr-containing Hf hydrides. For reference, the peak positions corresponding to δ-phase Hf hydride and δ-phase Zr hydride are also shown [Citation6]. No peaks other than the peaks associated with δ-phase hydrides were observed. shows the dependence of the lattice constant on the Hf content, determined from the XRD patterns. The lattice constant for δ-phase Zr hydride reported in the literature is also shown for reference [Citation5]. The dotted line simply connects this reported value to the value for the high-purity Zr0.0175Hf0.9825H1.62 determined in this study. For the Zr-containing Hf hydrides, the lattice constants are seen to generally obey Vegard's law, in that they increase almost linearly with Zr content. From the above results, the as-prepared Zr-containing Hf hydrides are single δ-phase hydrides, and are solid solutions of δ-phase Hf hydride and δ-phase Zr hydride. In the solid solution, it is considered that Zr and Hf atoms randomly occupy the metal site of the δ-phase metal hydride.
Figure 1. XRD patterns for Zr-containing Hf hydrides (Zr0.0175Hf0.9825H1.63, Zr0.045Hf0.955H1.63, Zr0.10Hf0.90H1.67), together with literature data for Zr hydrides and Hf hydrides.
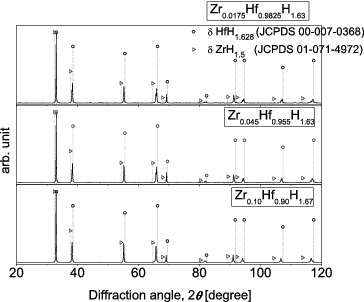
Figure 2. Cubic lattice parameter for Zr-containing Hf hydrides as a function of Hf content, x, together with literature value for Zr hydrides.
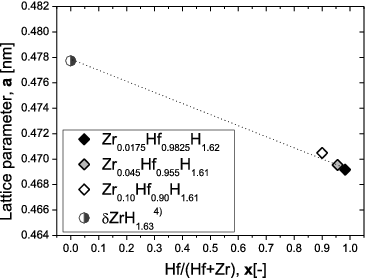
shows SEM/EDX analysis results for the Zr-containing Hf hydride with the highest Zr content (Zr0.10Hf0.90H1.68). In the SEM micrograph, a uniform microstructure can be observed, and the EDX maps for Hf and Zr indicate a uniform distribution of both elements. These results are in good agreement with the XRD results, which indicate that the samples are single δ-phase hydrides.
shows the dependence of the density of the Zr-containing Hf hydrides on the hydrogen content (H/M). For reference, the reported hydrogen content dependence of the densities of δ-phase Hf hydride and δ-phase Zr hydride is also shown [Citation5,Citation7]. The density is seen to decrease with increasing Zr content, which is reasonable given that Zr has a smaller atomic number than Hf.
3.2. High-temperature stability of Zr-containing Hf hydrides
shows the TG-DTA results for the Zr-containing Hf hydrides, when the measurements were carried out in an argon gas flow. For both the TG curves and DTA curves, no special change occurs up to about 800 K, and then both curves begin to sharply decrease. This sharp decrease is considered to be associated with the release of hydrogen from the δ-phase hydrides. The TG-DTA analysis revealed no significant differences among samples with different Zr contents.
shows the results of TG-DTA measurements in an air atmosphere. In contrast to the case for an argon gas flow, the TG curves do not begin to decrease at about 800 K, but instead increase slightly. This is considered to be due to oxidation of the samples to form Hf oxide, which has a higher molecular weight. Release of hydrogen is still considered to occur above about 800 K, but the resulting loss of mass is compensated by the mass increase associated with oxide formation. Again, from the TG-DTA results, no significant differences were found among samples with different Zr contents.
shows high-temperature XRD patterns for the Zr-containing Hf hydrides measured in a helium gas flow. The peak positions corresponding to δ-phase Hf hydride and α-phase metallic Hf are shown for reference [Citation6]. For Zr0.045Hf0.955H1.63 in the temperature range of 773–973 K, enlarged XRD patterns are also shown in the diffraction angle range of 20°–80°. All of the samples were single δ-phase hydrides before the high-temperature XRD measurements were carried out. Although peak shifts due to thermal expansion are observed, no major change in the XRD patterns occurs with increasing temperature up to 773 K. However, at 873 K, new peaks that appear to be associated with α-phase metallic Hf can be observed, and these peaks become more prominent at 973 K. At the same time, the intensity of peaks associated with the original δ-phase hydride decreases. From the above results, it is considered that release of hydrogen begins to take place for all samples at 873 K, which is consistent with the TG-DTA results obtained in an argon gas flow. Although there were some minor variations in peak intensities, the high-temperature XRD results indicated no major differences among samples with different Zr contents, suggesting that they had similar phase structures.
Figure 7. High-temperature XRD patterns for Zr-containing Hf hydrides in helium gas flow, together with literature data for Hf hydrides and Hf.
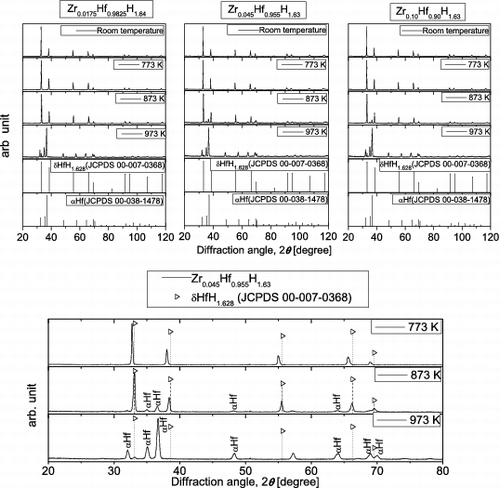
shows high-temperature XRD patterns for Zr-containing Hf hydrides obtained in an air atmosphere. For reference, peak positions corresponding to δ-phase Hf hydride, α-phase metallic Hf, Hf oxide (HfO2), and ϵ-phase Hf hydride are shown [Citation6]. For Zr0.045Hf0.955H1.63 in the temperature range of 773–973 K, enlarged XRD patterns are also shown in the diffraction angle range of 20°–80°. All of the samples were single δ-phase hydrides before the high-temperature XRD measurements. Although peak shifts due to thermal expansion are observed, no major change in the XRD patterns occurred with increasing temperature up to 673 K. However, at 773 K or higher, new peaks associated with HfO2 and apparently ϵ-phase Hf hydride are observed. These peaks become more prominent with increasing temperature, whereas the intensity of peaks associated with the original δ-phase hydride decreases. At 773 K or higher, peaks that appear to be associated with α-phase metallic Hf are observed, although they are weak. These results suggest that oxide is beginning to form in all samples at 773 K, with the concurrent release of hydrogen, which is consistent with the results for the TG-DTA measurements in air. Although there were some minor variations in peak intensities, the high-temperature XRD results indicated no major differences among samples with different Zr contents, suggesting that they had similar phase structures, as was the case for the measurements made in a helium gas flow.
Figure 8. High-temperature XRD patterns for Zr-containing Hf hydrides in air, together with literature data for Hf hydrides, Hf, and Hf oxide.
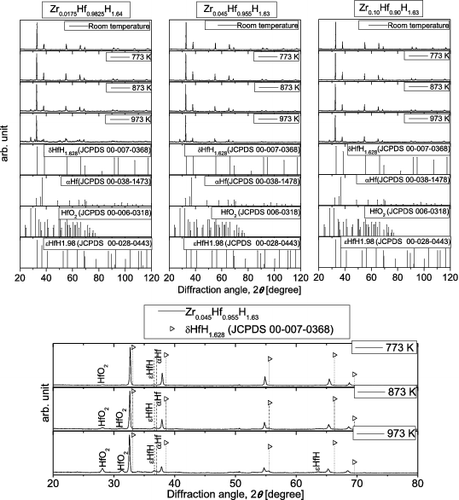
The results of high-temperature XRD measurements carried out in both helium and air indicated that at high temperatures, the release of hydrogen is accompanied by a reduction in the intensity of the peak associated with the δ-phase hydride. In addition, peaks due to HfO2 and apparently ϵ-phase Hf hydride appear only for the measurements carried out in air. In this study, the hydrogen and oxygen content in the samples could not be directly determined by the high-temperature XRD measurements in air. However, it is considered that both hydrogen release and oxidation occur as the temperature increases. Based on the Zr–O–H ternary phase diagram at 973 K obtained from thermodynamic equilibrium calculations [Citation8], ϵ-phase Zr hydride is expected to be present even when the hydrogen content is low, so long as the oxygen content is high. In a similar manner, ϵ-phase Hf hydride is considered to be present in the samples of this study, probably due to the increase in oxygen content at 973 K.
3.3. Thermal properties of Zr-containing Hf hydrides
Lattice parameter, sample bulk density, and relative density of representative samples for measurements of thermal and mechanical properties are summarized in . shows the temperature dependence of the lattice constant for the Zr-containing Hf hydrides, calculated from the high-temperature XRD results. The straight lines represent linear fits to the data for each sample. It should be noted that measurements of various thermal properties were carried out using samples with similar H/M ratios and with different Zr contents from room temperature to 673 K. The lattice constant is seen to increase almost linearly with temperature, allowing the average coefficient of linear thermal expansion in the range of 298–673 K to be determined using the following equation [Citation9]:
(1)
(1)
Table 1. Lattice parameter, sample bulk density, and relative density of representative samples for measurements of thermal and mechanical properties.
Here, αp(T) is the average coefficient of linear thermal expansion, a0 is the lattice constant at room temperature, and T is the absolute temperature. The results are listed below.
(2)
(2)
(3)
(3)
(4)
(4)
Thus, it was found that for Hf hydrides containing 4.5 and 10 at% Zr, αp(T) was lower than that for the high-purity Hf hydride containing little Zr.
shows the temperature dependence of the specific heat capacity for the Zr-containing Hf hydrides. For reference, literature data for Zr hydride [Citation10] are also shown. The specific heat capacity is seen to increase with temperature. In metal hydrides, the acoustic mode associated with the metal and the optical mode associated with the hydrogen have very different characteristic temperatures. As previously reported for Hf hydride [Citation7], the specific heat capacity in the temperature range considered in this study is believed to increase because of the effects of hydrogen vibrations with a high characteristic temperature. In addition, shows that the specific heat capacity increases slightly with Zr content.
Figure 10. Specific heat capacity for Zr-containing Hf hydrides as a function of temperature, together with literature data for Zr hydrides.
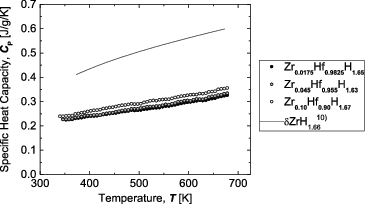
shows the temperature dependence of the thermal diffusivity for the Zr-containing Hf hydrides. For reference, literature data for Zr hydride and Hf hydride [Citation7,Citation10] are also shown. The thermal diffusivity generally decreases with increasing temperature. In addition, it decreases with increasing Zr content up to 10 at%.
Figure 11. Thermal diffusivity for Zr-containing Hf hydrides as a function of temperature, together with literature data for Hf hydrides and Zr hydrides.
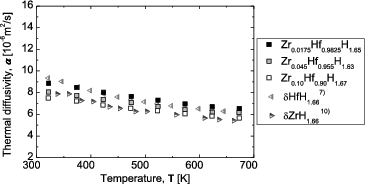
shows the temperature dependence of the thermal conductivity for the Zr-containing Hf hydrides. For reference, literature data for Zr hydride and Hf hydride [Citation7,Citation10] are shown. The thermal conductivity for the high-purity Hf hydride, containing little Zr, is seen to be slightly lower than that previously reported for Hf hydride. Thus, the purity and particle size of the Hf used for hydrogenation are thought to affect the thermal conductivity of the hydride. In , it can be seen that the thermal conductivity for all the Zr-containing Hf hydrides is relatively independent of temperature, which is consistent with the trend observed for Hf hydride and Zr hydride. The thermal conductivity can be approximately expressed as the sum of the lattice and the electronic components. From previously reported results for δ-phase Hf hydride [Citation7], in the temperature range of 300–650 K, the lattice component decreases with increasing temperature, whereas the electronic component increases. As a result, the thermal conductivity of δ-phase Hf hydride is independent of temperature and almost constant. Although additional verification such as electric conductivity measurement is necessary, it is believed that a similar argument can be applied to the Zr-containing Hf hydrides in the present study. On the other hand, the thermal conductivity of Zr-containing Hf hydrides decreases with increasing Zr content up to 10 at%. This is probably the result of scattering of the phonons responsible for the lattice thermal conductivity by the addition of Zr atoms.
3.4. Mechanical properties of Zr-containing Hf hydrides
shows the hydrogen content dependence of the Vickers hardness for the Zr-containing Hf hydrides. For reference, literature data for metallic Hf, Hf hydride, and Zr hydride [Citation4,5] are shown. The Vickers hardness is seen to decrease slightly with increasing hydrogen content and with increasing Zr content.
Figure 13. Vickers hardness for Zr-containing Hf hydrides as a function of hydrogen content (CH = H/M), together with literature data for pure Hf, Hf hydrides and Zr hydrides.
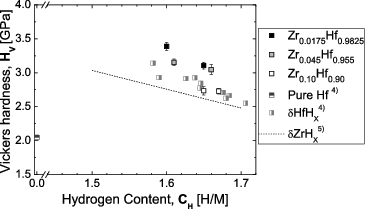
shows the hydrogen content dependence of Young's modulus for the Zr-containing Hf hydrides. For reference, literature data for metallic Hf, Hf hydride, and Zr hydride [Citation4,5] are shown. Young's modulus is seen to decrease with increasing hydrogen content. No significant dependence on the Zr content is found.
Figure 14. Young's modulus of the hydrides of Zr–Hf alloys as a function of the hydrogen content (CH = H/M), together with the literature data of pure Hf, Zr hydrides, and Hf hydrides.
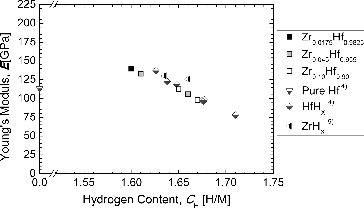
In the present study, the Vickers hardness of Zr-containing Hf hydrides decreased with increasing Zr content. This seems to be reasonable because, basically, the Vickers hardness of δ-phase Zr hydrides is smaller than that of δ-phase Hf hydrides. On the other hand, the Zr-containing Hf hydrides showed almost the same values in the Young's modulus regardless of the Zr content. This seems to be reasonable because the Young's moduli of δ-phase Zr hydrides and δ-phase Hf hydrides are similar when they contain a similar amount of hydrogen.
4. Summary
In this study, Zr-containing Hf hydrides were prepared by hydrogenating Zr-containing Hf alloys (Zr0.0175Hf0.9825, Zr0.045Hf0.955, and Zr0.10Hf0.90), and the phase structure and microstructure were investigated. The results allowed clarification of the effects of the Zr content on the high-temperature stability and thermal and mechanical properties of Hf hydride.
From the results of XRD and SEM/EDX analyses, the as-prepared Zr-containing Hf hydrides were confirmed to be single δ-phase hydrides. Their high-temperature stability and thermal and mechanical properties were evaluated, and the following results were obtained.
In an open system, the release of hydrogen takes place at a high temperature, and the phase structure changes.
At high temperatures, the presence or absence of oxygen has a large effect on the phase structure.
A Zr content of up to 10 at% does not strongly influence the high-temperature phase structure or stability either in air or in an inert gas flow.
The specific heat capacity increases with Zr content up to 10 at%. On the other hand, the density, Vickers hardness, average coefficient of linear thermal expansion in the range of 298–673 K, thermal diffusivity, and thermal conductivity decrease. There is no significant influence of the Zr content on Young's modulus.
In this study, bulk Zr-containing Hf hydrides containing up to 10 at% Zr were prepared, and were free from cracks or chips. Thus, the effects of the Zr impurity on their high-temperature stability and thermal and mechanical properties could be evaluated. All the samples tested had a single δ-phase, and their hydrogen contents were very similar. Thus, only the effects of Zr could be clearly evaluated. The results of this study indicated that there is no big difference in the high-temperature stability between Hf hydride prepared from high-purity Hf and that prepared from Hf containing a small amount of Zr; however, there are distinct differences with regard to the thermal and mechanical properties. Thus, when high-temperature stability is important, it is not really necessary to dwell on the purity of the Hf. However, when the material properties are important, reactor-grade Hf with an extremely low Zr content should be used, or it would be necessary to carry out a precise quantitative evaluation of the effects of the Zr content on the material properties. It is considered that the results obtained in this study will provide essential basic information when Hf hydride is used as control rods in future fast reactors.
Acknowledgements
The present study includes results from “Development Study of Fast Reactor Core with Hydride Neutron Absorber” entrusted to Tohoku University by the Ministry of Education, Culture, Sports, Science and Technology of Japan (MEXT).
References
- Konashi K, Yamawaki M. Utilization of hydride materials in nuclear reactors. Adv Sci Technol. 2010;73:51–58.
- Tahara Y, Iwasaki T, Konashi K. Development of hydride absorber for fast reactor – evaluation of design method for hydride absorber rod. J Nucl Sci Technol. 2010;47:421–429.
- Massalski TB, Okamoto H, Subramanian PR, Kacprzakk L, editors. Binary alloy phase diagrams. 2nd ed. Materials Park (OH): ASM International; 1990.
- Ito M, Kurosaki K, Muta H, Uno M, Konashi K, Yamanaka S. Thermomechanical properties of hafnium hydride. J Nucl Sci Technol. 2010;47:156–159.
- Yamanaka S, Yoshioka K, Uno M, Katsura M, Anada H, Matsuda T, Kobayashi S. Thermal and mechanical properties of zirconium hydride. J Alloys Compounds. 1999;293–295:23–29.
- International Centre for Diffraction Data, Joint Committee on Powder Diffraction Standards Card No. 07-0368 (HfH1.628), No. 28-0443 (HfH1.98), No. 71-4972 (ZrH1.5), No. 38-1478 (Hf), No. 06-0318 (HfO2).
- Ito M, Kurosaki K, Muta H, Uno M, Konashi K, Yamanaka S. Thermal conductivity of hafnium hydride. J Nucl Sci Technol. 2009;46:814–818.
- Miyake M, Uno M, Yamanaka S. On the zirconium-oxygen-hydrogen ternary system. J Nucl Mater. 1999;270:233–241.
- Morocoima M, Quintero M, Guerrero E, Tovar R, Conflant P. Temperature variation of lattice parameters and thermal expansion coefficients of the compound ZnGa2Se4. J Phys Chem Solids. 1997;58:503–507.
- Yamanaka S, Yamada K, Kurosaki K, Uno M, Takeda K, Anada H, Kobayashi S. Thermal properties of zirconium hydride. J Alloys Compounds. 2001;294:94–98.