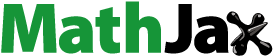
ABSTRACT
In the boiling and drying accident of high-level liquid waste in the fuel reprocessing plant, behavior of ruthenium (Ru) has attracted much attention because Ru could form volatile compounds such as ruthenium tetroxide (RuO4) and could be released into the environment. To contribute towards safety evaluation of this accident, the migration behavior and the leak path factor of the gaseous ruthenium compound has been experimentally measured in this study. The experiment was proceeded by using the ‘Ruthenium Migration Evaluating Apparatus,’ which partially simulates the atmospheric condition (temperature, flow rate, and composition of water vapor and gaseous nitric acid mixture) of migration pathway in the accident. Experiments with dry air and water vapor were also performed as the control experiment to discuss the effect of nitric acid. As a result, the experiment with dry air and the experiment with water vapor demonstrated that the majority of the ruthenium deposited along the migration pathway. On the other hand, the experiment with the water vapor containing gaseous nitric acid demonstrated that almost all of the ruthenium passed through the migration pathway without deposition. These results suggested that the migration behavior of gaseous ruthenium will be affected by the gas-phase composition.
1. Introduction
After the Fukushima-Daiichi nuclear power plant accident, the licensing standards were further strengthened, in which countermeasures against severe accidents were newly required as regulatory items [Citation1]. Severe accidents at fuel cycle facilities were defined as serious accidents that occur under conditions beyond design-basis conditions. Establishment of methods for the quantitative evaluation of the progress of the accidents including the effectiveness of countermeasures under serious conditions is an urgent issue for confirmation of the safety of the facilities.
High-level liquid waste (HLLW) in PUREX reprocessing plants is constantly heated by decay heat derived from fission products. Accordingly, the HLLW is always cooled in the HLLW tanks of reprocessing plants. A shutdown of the tank cooling system resulting from both the loss of offsite power and failure of all countermeasures may lead to the evaporation and dryness of the HLLW [Citation2,Citation3]. This accident, named ‘the evaporation to dryness due to the loss of cooling functions’ (EDLCF), was newly defined as one of the severe accidents in Japan's nuclear safety standard for reprocessing plants [Citation1].
In the EDLCF, it is assumed that radioactive substances are released together with nitric acid (HNO3) and water (H2O) vapor due to evaporation and dryness of HLLW. To establish the severe accident management measures of the EDLCF, evaluation of the behavior of ruthenium (Ru) is essential because Ru has radiotoxicity (106Ru and 103Ru) [Citation4] and it is released at a greater fraction to initial amount in HLLW than other elements by forming volatile Ru compounds [Citation2,Citation5–7]. Ruthenium tetroxide (RuO4) is known as a volatile Ru compound, and the main gaseous Ru which is generated in the EDLCF is considered to be RuO4 [Citation5]. Since RuO4 is a reactive and unstable compound [Citation8], gaseous RuO4 (RuO4(g)) released in the EDLCF can be trapped in the migration pathway of the reprocessing plant. Therefore, the migration behavior of gaseous Ru, is an important information for the source term analysis of the EDLCF.
There are, however, few reports available based on the experimental studies of the accident progress and source terms about migration behavior of Ru.
Abe et al. reported the qualitative results of the migration behavior of RuO4(g) in nitrogen (N2), H2O vapor, and HNO3 + H2O vapor [Citation9]. The results demonstrated that the RuO4(g) deposited on the migration pathway in N2, whereas almost all Ru passed through the migration pathway and kept gaseous form in the HNO3 + H2O vapor. However, the report lacks the results on material balance, and it discussed only qualitative results. In addition, this experiment employed N2 as coexisting gas; hence their results exclude the effect of oxygen (O2).
Kodama et al. reported the migration behavior of the materials with the boiling experiments of a non-radioactive simulant of HLLW [Citation10]. The results showed that the collection rate of Ru decreased with the temperature rise in the migration pathway. However, these results of mass transport were affected by various phenomena such as composition changes of vapor phase with the evaporation of the HLLW simulant, condensation of H2O and HNO3 vapor, and re-evaporation caused by heat up of migration pathway.
In order to contribute to an assessment of this accident, we conducted experiments to evaluate the interactions between RuO4(g) and coexisting gasses in the EDLCF. The migration behavior of Ru(g) under the EDLCF condition was evaluated with a mass balance analysis by using the Ruthenium Migration Evaluating Apparatus (RMEA), which ensured steady supplies of RuO4(g) and HNO3 + H2O vapor. In this paper, we provide the quantitative experimental results about the migration behavior of gaseous Ru compound (Ru(g)) on the EDLCF conditions.
2. Experimental
2.1. Apparatus
The experiments were performed by using the RMEA, which partially simulates the volatilization and migration of the Ru in the EDLCF (). The RMEA is an experimental apparatus used in reference [Citation9] with a small modification. The Ru(g) and coexisting gas were stably supplied into the reaction pipes, which simulated the migration pathway in the reprocessing plant. The RMEA consists of four major parts: a gaseous RuO4 generator, a vapor generator, reaction pipes, and a ruthenium recovery system. The RuO4 was employed as a typical volatile ruthenium compound in this study. Generation of RuO4(g) was performed by sublimating solid RuO4 (RuO4(s)) in the gaseous RuO4 generator. The RuO4(s) was contained in a glass container (temperature controlled at −10 °C), and sublimated RuO4(g) was supplied to the downstream by the dry air carrier. In order to prevent decomposition of RuO4 in the supply line before the reaction pipes, a part of supply line was cooled to 20 °C with cooled water (). Generated amount of Ru was approximately 0.5 × 10−6 to 1.0 × 10−6 mol/min when the flow rate of carrier gas was set to 0.1 NL/min. Stability of the generation rate of RuO4(g) was confirmed by evaluating the supply rate of RuO4(g) by using gas absorption bottle (B) (). Generation of H2O vapor and HNO3 + H2O vapor were performed by using the vapor generator, which consists of two liquid pumps, an atomizer, and a mantle heater. In order to stabilize the amount of mist generation from the atomizer, the liquid level was stabilized by using two liquid pumps. The whole of reaction pipes (6 cm I.D. 20 cm × 9 parts, inner surface area = 377 cm2, volume = 565 cm3, Pyrex glass) and Inlet/Outlet connectors (inner surface area = approximately 189 cm2, volume = 255 cm3, Pyrex glass) were housed in the thermostatic chamber to prevent temperature difference between inside and outside of the reaction pipes. The Ru(g), which passed through the reaction pipes, was collected by a ruthenium recovery system, which consists of a glass filter (ADVANTEC, 86R, nominal filtration rating = 1 μm, collection efficiency is 99.9% for 0.3 μm DOP, for collecting aerosols), a Liebig condenser (4 °C, for collecting Ru(g), H2O, and HNO3), and two gas washing bottles (filled with 300 ml of 0.1 mol/L NaOH, for collecting Ru(g)).
2.2. Calculation of LPF
The Leak Path Factor (LPF) [Citation11] was applied for the evaluation of migration behavior of Ru. In this study, the LPF was calculated as the ratio between the total amount of collected Ru and the amount of Ru which deposited on the reaction pipes (Equation Equation1(1)
(1) ). The LPF and migration behavior of the Ru were discussed from the comparison among the amounts of the Ru which was trapped in each apparatus part in the RMEA.
(1)
(1)
2.3. Experimental condition
The condition of RMEA experiments is summarized in . The following conditions were set as the experimental conditions. Run 1 was a control experiment for measuring the stability of RuO4(g) in the dry air. Run 2 was a control experiment to evaluate the influence of H2O vapor on the RuO4(g). Run 3 was an experiment to evaluate the influence of H2O vapor containing gaseous HNO3, which is gas phase composition at the EDLCF, on the RuO4(g). The composition of the sample gas (mixed gas of air, RuO4, HNO3, and H2O) was set to the value from the heating experiment of the simulant of HLLW (s-HLLW), which was carried out to simulate the EDLCF [Citation12]. shows the time dependence of the temperature of s-HLLW, the release amount of Ru, and the molar ratio of HNO3:Ru and H2O:Ru, which is calculated from the composition of the condensate in the experiment of reference [Citation12]. We employed the vapor phase composition under which the release amount of Ru took the maximum in (Ru:HNO3:H2O = 1:1.3 × 103:5.0 × 103) for the experiments as the most important condition to simulate the EDLCF. The temperature of the sample gas and the inside of thermostatic chamber were set to 150 °C in this study. This value was set with reference to the temperature range (approximately 140–170 °C), where the release rate of Ru was large in the results of the heating experiment of the s-HLLW [Citation9,Citation12,Citation13,Citation14]. In all experiments, the supply rate of the sample gas was set at 0.7 L/min (4.7 × 10−3 m/sec in the reaction pipes) by adjusting the carrier gas flow rate ().
Table 1. Experimental conditions of RMEA experiment
Figure 2. Time dependence of molar ratio of components, temperature, and released amount of Ru in heating experiment of s-HLLW [Citation12].
![Figure 2. Time dependence of molar ratio of components, temperature, and released amount of Ru in heating experiment of s-HLLW [Citation12].](/cms/asset/d1beb0bd-0865-4103-a825-32af0aa9fe13/tnst_a_1428121_f0002_c.jpg)
2.4. Experimental procedure
A typical procedure of the experiments (Run 3 in ) was as follows: to obtain the supply rate of the RuO4(g) and the amount of total supplied Ru (), the RuO4(g) was firstly supplied with 0.1 NL/min carrier gas to the gas washing bottle (B) () for 10 min and the concentration of Ru in the absorbent of the gas washing bottle (B) was analyzed quantitatively by using ICP-MS (Perkin-Elmer, ELAN DRC-e).
Table 2. Results of material balance in RMEA experiment
After evaluating the supply rate of RuO4(g), the supply rate of the HNO3 aqueous solution was set to a value such that Ru:HNO3:H2O became 1:1300:5000, and the flow rate of carrier gas was set so that the flow rate of the sample gas in the reaction pipes was 0.7 L/min. The temperature of the heated lines and thermostatic chamber were set at a constant value of 150 °C. Next, the internal gas of the reaction pipes was replaced with the sample gas without RuO4 beforehand for 20 min. After that, the RuO4 in the dry air carrier merged and the sample gas was fed into the reaction pipes for 20 min.
After the completion of the sample gas supply, the HNO3 + H2O vapor was supplied for 20 min to purge the remaining Ru(g). Dry air (0.3 NL/min) was subsequently supplied for flushing of the reaction pipes. After the flushing, the reaction pipes, the glass filter, and all other parts were washed with HNO3aq. (1.0 mol/L HNO3, eluent for deposited RuO4), and then immersed into the elution agent for RuO2 [Citation15] (a mixture of 5 g/L potassium persulfate and 0.2 mol/L sodium hydrate) for more than two days to peel and dissolve the Ru which deposited on the glass surface. After the black deposit on the glass surface disappeared, insoluble residues in the elution agent were collected by using a filter paper and then dissolved by alkali fusion (with sodium peroxide, 650 °C, 5 min). The quantitative analysis of Ru was performed by using ICP-MS.
3. Results and discussion
The experimental results are summarized in and , and . The result of the LPF values and distribution of the Ru deposition differed according to the atmospheric composition in the gas phase. The amount of total supplied Ru corresponded with the total collected Ru (95.2%–99.6%) (). These results show that the migration behaviors of Ru were quantitatively obtained with acceptable losses of Ru in this study.
Table 3. Results of LPF and Ru distribution in RMEA experiment
3.1. Dry air atmosphere (Run 1)
As a control experiment, a RMEA experiment was performed with dry air to ensure that the RuO4 in dry air is metastable at around room temperature while it is unstable at approximately 150 °C (Run 1) [Citation16]. The results showed that almost all of the Ru was collected in the reaction pipes (LPF = 5.1 × 10−3) (, ). shows the deposition amount on each reaction pipe with respect to the total collected amount and the density of Ru per a unit inner surface area. The deposition densities of Ru were decreased along with the migration distance and the main part of Ru was collected in the inlet connector and the pipe #1. The Ru depositions on the glass surface were not dissolved by the HNO3aq. washing, whereas almost all of them were soluble or collectible in the elution agent (). These elution behaviors corresponded to the chemical properties of RuO2 [Citation15,Citation17]. is a photograph of inlet connector to pipe #2 after the completion of Ru supply. More black stains, which appeared to be RuO2 [Citation17], were observed on the inside surface of the inlet connector and the pipe #1, in contrast to the pipe #2 which had less stains. These results indicate that gaseous RuO4 had rapidly decomposed and deposited on the glass surface as RuO2 under the dry air atmosphere. This behavior was similar to the result of the experiment with the N2 atmosphere (LPF = 3 × 10−3) [Citation9]. These results suggested that the O2 does not play a critical role in the prevention of the RuO4 decomposition.
3.2. Water vapor atmosphere (Run 2)
An experiment with the Air + H2O vapor atmosphere (Run 2) was also performed as a control experiment. As in the case of the experiment with the dry air atmosphere, a majority of the Ru (77.8%) deposited on the reaction pipes (LPF = 0.22) (, ). On the other hand, most of the rest of the Ru (21.8%) was collected on the glass filter. The deposition density of Ru decreased along with the migration distance in the experiment with the Air + H2O vapor atmosphere as well as that with the dry air (). The black deposition was observed on the glass filter after the Ru supply (). This deposition was hardly extracted by the HNO3aq. (0.7%), whereas it was dissolved in the elution agent (21.1%) (). These results suggested that the collected Ru on the glass filter was RuO2 aerosol, which formed in the vapor phase. In addition, there is a possibility that H2O vapor was interfering with the deposition of Ru on the glass surface. Mun et al. reported that the decomposition behaviors of RuO4(g) in the gas phase were different between moist air and dry air [Citation15]. The results of our experiment were compatible with their observation.
3.3. Nitric acid and water vapor atmosphere (Run 3)
The experiments, which simulated the atmospheric condition of the EDLCF, were performed by using Air + HNO3 + H2O vapor atmosphere (Run 3). In contrast to the above results, almost no deposition of Ru was observed and detected in both of the reaction pipes and the glass filter (LPF > 0.99) (, ). These results indicate that the supplied RuO4 passed through the reaction pipes and the glass filter as Ru(g) without deposition in the Air + HNO3 + H2O vapor atmosphere. The results also suggested that either the nitric acid stabilized gaseous RuO4, or the RuO4 reacted with the nitric acid or nitric oxides and took another chemical form. The chemical form of Ru(g) in the simulated calcination process was predicted to be nitrosylruthenium [Citation18,Citation19]. However, the structure of Ru(g) in the Air + HNO3 + H2O vapor has not yet been characterized on account of the difficulty of isolation from the condensable gas. In addition, reports on the volatile nitrate complexes of Ru are still not enough. The chemical speciation of the Ru(g) in the Air + HNO3 + H2O vapor is an outstanding issue. It is important to note that almost all of Ru(g) was trapped in the condensate (). This result implies that the removal of Ru(g) from vapor phase would be achieved by the migration to the condensate, which is influenced by changes of vapor phase composition.
4. Conclusion
In this study, we evaluated the migration behaviors of the Ru(g) with some atmospheric conditions including the Air + HNO3 + H2O vapor, which is assumed in the EDLCF. The migration behavior of the Ru(g) has been found to be influenced by the atmospheric condition as follows:
• Almost all of the Ru(g) was deposited on the reaction pipes in the experiment with the dry air atmosphere (LPF = 5.1 × 10−3).
• The majority of the Ru(g) were deposited under the Air + H2O vapor atmosphere (LPF = 0.22), whereas most of the rest Ru migrated as the RuO2 aerosol.
• Almost all of Ru(g) passed through the reaction pipes without deposition when the experiments proceeded under the Air + HNO3 + H2O vapor atmosphere (LPF > 0.99).
These results may contribute towards the improvement of accident management measures and the assessment of their effectiveness of the EDLCF.
Supplemental_Data.pdf
Download PDF (123.8 KB)Acknowledgments
The authors wish to thank Dr Y. Yamane whose comments and suggestions were of inestimable value for this study. Special thanks also go to Mr T. Masaki and the members of Fuel Cycle Research Group of JAEA who provided technical help.
Disclosure statement
No potential conflict of interest was reported by the authors.
References
- Nuclear Regulation Authority (Japan). Outline of the draft new regulatory requirements for nuclear fuel facilities, research reactors, and nuclear waste storage/disposal facilities [Internet]. Tokyo, Japan: NRA (Japan); [cited 2018 Jan 18]. Available from: http://www.nsr.go.jp/data/000067274.pdf.
- Philippe M. Mercier JP, Gue JP. Behavior of ruthenium in the case of shutdown of the cooling system of HLLW storage tanks. In: First MW, editor, Proceeding of the 21st DOE/NRC Nuclear Air Cleaning Conference; 1990 Aug. San Diego (CA): The Harvard Air Cleaning Laboratory, 1991. p. 831–843.
- Miyata T, Takebe K, Tamauchi Y, et al. Application of Probabilistic Safety Assessment to Rokkasho Reprocessing Plant, (II) The Occurrence Frequency of Boiling Accident of Highly Active Liquid Waste. Nihon-Genshiryoku-Gakkai Shi (J At Energy Soc Jpn). 2008;7:85–98. Japanese.
- Eichholz GG. Hazards and control of ruthenium in the nuclear fuel cycle. Prog Nucl Energy. 1978;2:29–76.
- Kato T, Usami T, Tsukada T, et al. Study on volatilization mechanism of ruthenium tetroxide from nitrosyl ruthenium nitrate by using mass spectrometer. J Nucl Mater. 2016;479:123–129.
- Sasahira A, Kawamura F. Formation Rate and Gas-Liquid Equilibrium of RuO4. J Nucl Sci Technol. 1988;25:472–478.
- Sasahira A, Hoshikawa T, Kamoshida M, et al. Transfer of Ruthenium from a Simulated Reprocessing Solution to Gas Phase during a Continuous Distillation. J Nucl Sci Technol. 1996;33:753–757.
- Griffith WP. Catalysis by metal complexes 34: ruthenium oxidation complexes. Netherlands: Springer; 2011. Chapter 1, Ru(VIII) Complexes; p. 7–30.
- Japan Atomic Energy Agency. Report of study on release and transport of radioactive materials in reprocessing plant. Project management group of study on release and transport of radioactive materials in reprocessing plant. Japan: Japan Atomic Energy Agency; 2014. Japanese.
- Kodama T, Shibata Y, Takahashi N, et al. Experiments on the leak path factor of ruthenium volatilized from high-level liquid waste tanks in a reprocessing plant in case of the boiling and drying accident. J Nucl Sci Technol. 2015;52:467–471.
- DOE Handbook. Airborne Release Fractions/Rates and Respirable Fractions for Nonreactor Nuclear Facilities Volume I - Analysis of Experimental Data. USA: Department of Energy; 1994. DOE-HDBK-3010-94.
- Tashiro S, Uchiyama G, Amano Y, et al. Release of Radioactive Materials from Simulated High-Level Liquid Waste at Boiling Accident in Reprocessing Plant. Nucl Technol. 2015;190:207–213.
- Yoshida K, Ishikawa J. Thermal-hydraulic analysis of boiling event of reprocessed liquid wastes with MELCOR code. Japan: Japan Atomic Energy Agency; 2012. p. 26. ( JAEA-Research; JAEA-Research 2012-026). Japanese.
- Tashiro S, Amano Y, Yoshida K, et al. Release Characteristics of Ruthenium from Highly Active Liquid Waste in Drying Step. Trans At Energy Soc Japan. 2015;14:227–234. Japanese.
- Mun C, Cantrel L, Madic C. Study of RuO4 decomposition in dry and moist air. Radiochim. Acta. 2007;95:643–656.
- Ortner MH, Anderson CJ, Campbell PF. Research and development studies on waste storage process. Idaho Falls (ID): U.S. Atomic Energy Commission; 1961. IDO-14504.
- Perry LD. Handbook of inorganic compounds. 2nd ed. USA: CRC Press; 2011. Inorganic Compound Data, p. 353.
- Igarashi H, Kato K, Takahashi T. Effect of calcining temperature on volatilization of ruthenium in batch calcination of simulated high-level liquid waste. Radiochim Acta. 1993;60:143–148.
- Klein M, Weyers C, Goossens WRA. Volatilization and trapping of ruthenium in high temperature processes. In: Proceedings of the 17th DOE Nuclear Air Cleaning Conference, 2-5 August 1982, Denver, CO., USA. USA: Department of Energy. 1982. Vol. 1. p. 371–380