ABSTRACT
Boron (B) chemistry during transportation at temperatures above 400 K was experimentally investigated to evaluate the transport behavior of control rod material B during a severe accident (SA) of a boiling water reactor while considering effects of B on cesium (Cs) and iodine (I) behavior. The heating test on B2O3 specimens was conducted at 1150 K in a steam atmosphere using the dedicated experimental apparatus named the TeRRa reproducing release and transportation of B in an SA. New findings on B chemistry in the high-temperature region emerged. A large amount of released B2O3 vapor was deposited above 1000 K by condensation on stainless steel (SS). The B deposits and/or vapors significantly reacted with SS above 1000 K, forming 2FeO · FeBO3 The experimental data indicate that a large amount of released B from a degraded BWR control blade in an SA remains in the high-temperature region like a Reactor Pressure Vessel (RPV). These results and thermodynamic considerations suggest the presence of B deposits in the high-temperature region decrease the amount of Cs vapors transported from an RPV. This is attributed to the possible formation of low volatile compounds like CsBO2 from the reaction of B deposits and Cs vapors.
1. Introduction
The severe accident (SA) of the Fukushima Dai-ichi (1F) nuclear power station (NPS) and the enhancement of light water reactor (LWR) safety that followed reiterate the importance of improving the source term estimations [Citation1–3]. This requires a deeper understanding of and better evaluation technology for release behavior from a nuclear fuel and transport behavior in a reactor (release and transport behaviors) of fission products (FPs) for the improvement of SA analysis codes like THALES-2 [Citation4], SAMPSON [Citation5], and MELCOR [Citation6], employed for source term assessments. Several uncertainties remain in the release and transport behaviors of FPs [Citation1–3]. The major reason for this is the inability of SA analysis codes to adequately handle FP chemistry [Citation1–3,Citation7].
We are, therefore, conducting a fundamental research on the FP chemistry [Citation7–9], because of its significant impact on the release and transport behaviors of FPs. This research primarily experimentally evaluates FP chemistry, and creates and/or improves FP chemistry models for a database named ECUME (Effective Chemistry database of fission products Under Multiphase rEaction) [Citation10,Citation11]. The ECUME database comprises three datasets including chemical reaction kinetics, elemental model, and thermodynamic properties, which are implementable in the SA analysis codes. Regarding the chemical system in ECUME, iodine (I) and cesium (Cs) are the most important FPs because of their significance in LWR safety enhancement and for decommissioning and dismantling of 1F NPS. In this context, molybdenum (Mo) also requires consideration because of its strong chemical impact on Cs and I [Citation12]. Additionally, the SA of 1F NPS highlights the needs for chemistry related to boron (B) in control rod material in BWR [Citation7,Citation8,Citation13]. This necessity is predicated on the paucity of knowledge on the effects on Cs and I relative to the silver-indium-cadmium (Ag-In-Cd) control rod materials in PWR.
In a BWR SA, melting of the BWR control rod and blade by the eutectic reaction of B4C and stainless steel (SS) involves downward relocation of the reactor core prior to the degradation of the fuel assembly [Citation14–18]. This phenomenon favors the co-existence of B4C and B4C-SS mixed melts because intact B4C remains and relocates downward with the melt [Citation17,Citation18]. The latter melt sometimes includes Zircaloy (Zry) from liquefaction of the Zry channel box, producing a B4C-SS-Zry mixed melt. Therefore, the intact B4C and the mixed melt constitute sources of B release. Experiments on the release behavior of B for B4C, and Zr-B and Fe-B compounds, possibly representative of products in the B4C-SS-Zry mixed melt, revealed B oxidation to B2O3 and then vaporization as B2O3 and boric acids like HBO2 and H3BO3 [Citation19–21].
Information on B transportation originates mostly from integral experiments like the representative FP release and transport experiment Phebus-FPT3 test [Citation22], the B4C control rod degradation experiments Quench 07 [Citation23], and BECARRE [Citation17]. The BECARRE experiment provided data suggesting transportation of released B as boric acid vapors (HBO2 and H3BO3) and condensation during transportation, explaining the high deposition in the lower temperature region (around 423 K). Most of the deposits are mainly composed of boric acid [Citation17].
In addition to the integral experiments, several basic studies on B and its effects on Cs and I during transportation exist. Thermodynamic studies demonstrate that the presence of the B vapors significantly impacts Cs chemistry through the formation of CsBO2, enhancing gaseous iodine generation [Citation13,Citation24]. Previous experimental studies also revealed the enhancement of gaseous iodine generation from the reaction of condensed CsI and B2O3 in the high-temperature region [Citation25]. This was predicted from the analysis results of the trapped sample in the lower temperature region. In the Phebus-FPT3 test incorporating a B4C control rod in the experimental system [Citation22], some enigmatic results like B-containing blockage formation with Cs compounds at around 423 K and the consequent increased gaseous iodine fraction in the simulated primary containment vessel (PCV) were noted. The Cs deposition and gaseous iodine formation can be attributed to the presence of B compounds.
Although B likely effects Cs and I during transportation, most of the experimental data were available on the transport behavior of B in the low-temperature region around 400 K reported in the integral experiments. This study, therefore, is an experimental investigation of B chemistry during transportation in the high-temperature region above 400 K. The dedicated experimental apparatus named TeRRa (Test bench for FP Release and tRansport) was used [Citation9]. Insight into B chemistry during transportation involved characterization of deposits and airborne materials. The likely effects of B on Cs and I chemistry in an SA were then discussed based on the experimental results and thermodynamic considerations.
2. Experimental method
2.1. Conditions of heating test reproducing boron transportation
Heating test reproducing B transportation in an SA condition was conducted using TeRRa. Although details of the facility are described in [Citation9], a brief introduction is still provided in this study. shows the basic specifications and components of TeRRa. The temperature in the induction furnace can attain about 2500 K, which is representative of the temperature around the molten core during an SA. The Thermal Gradient Tube (TGT) comprising 13 furnaces with a linear temperature gradient from 1000 K to 400 K is connected to the high-temperature furnaces. This temperature range represents that from the reactor coolant system (RCS) to PCV conditions during an SA. The deposits, airborne aerosol particles, and gas transported downstream to below 400 K are collected by the sampling tube, the aerosol filter, and the liquid trapping system, respectively. The airborne aerosol particles extracted at 100 K intervals of the downstream in TGT were subjected to an on-line measurement of particle size distribution, and an off-line analysis for the collected aerosol particles in the cascade impactor.
Figure 1. Basic specifications and components of the dedicated experimental apparatus named TeRRa reproducing FP release and transport
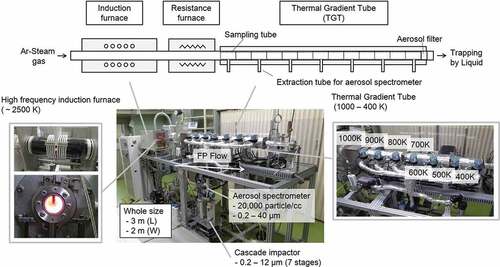
The conditions employed during heating test reproducing B transportation are presented in . The powder specimen of B2O3 (99.995%, Kojundo chemical laboratory Co., Ltd) was loaded into the resistance furnace. shows the temperature profile along the furnace and TGT. shows the preset temperature history of the resistance furnace and TGT. The duration of steam atmosphere is also shown in . First, the sampling tubes (SS type 304 L) were loaded into the resistance furnace and TGT as received. Then, the B2O3 specimen and the sampling tubes were heated at the heating rate of about 10 K/s and 25 K/s, respectively, in an Ar gas flow of 2 NL/min. The flow gas was changed to an Ar gas with a steam of 60°C of dew point (about 20% H2O) at about 920 K of resistance furnace where the vaporization of B2O3 specimen was quite limited. It means that the sampling tubes in the resistance furnace and TGT were oxidized depending on their temperature before the interaction between B vapors and sampling tubes. Thus, the representative conditions of SS in RCS and PCV during an SA could be reproduced. The B2O3 specimen was vaporized at about 1150 K which corresponded to about 1270 K in the center of resistance furnace. After the heating of the B2O3 specimen for 1 h at about 1150 K, the temperature of the resistance furnace was lowered at about 10 K/s.
Table 1. Fundamental conditions of heating test reproducing B transportation
2.2. Characterization of deposits and airborne materials
The trapped and deposited samples were characterized by Inductively Coupled Plasma–Mass Spectrometry (ICP–MS), X-ray diffraction (XRD), and Scanning Electron Microscopy. (SEM). The airborne materials during the heating test were extracted for every 100 K of the TGT from 1000 K to 400 K and their size distributions measured.
The ICP–MS involved B deposits collected in two steps of leaching from each sampling tube and aerosol filter to distinguish soluble and insoluble sample. Initially, the sampling tubes and aerosol filters were completely immersed in an adequate volume of 0.04 mol/L NaOH solution at room temperature for about 18 h to obtain soluble samples. The leaching in acid followed directly after the leaching by the alkali solution, to obtain insoluble samples. The sampling tubes and aerosol filters were introduced in an appropriate volume of 3 mol/L HNO3 solution at room temperature for 3 h. Prior to the ICP–MS analyses, sample solutions were diluted to appropriate concentrations using the same solution with leaching solvent. The diluted samples from the NaOH leaching (soluble samples) and HNO3 leaching (insoluble samples) were measured by ICP–MS (Agilent 8800, Agilent technology Inc.). The XRD (MiniFlex600SC, Rigaku Inc.) analysis was conducted to identify the chemical forms of deposits at room temperature using a Cu-Kα spectrum with 2θ ranging from 10 to 80 deg. in continuous mode, with a step size of 0.1 deg. and scanning speed of 1 deg./min. The applied voltage and current were 40 kV and 15 mA, respectively. The microstructure of deposits and trapped airborne materials were observed using an SEM-EDX (JSM-7610F, JEOL), with the electron beam voltage set to 15 keV. The size distributions of airborne materials were measured by an on-line optical particle counter (Welas, PALAS Inc.) for 5 min. The effective measurement range of particle size is 0.2–10 µm and is calibrated using polystyrene latex (PSL).
2.3. Chemical equilibrium calculation
The chemical forms and state of B compounds during transportation in TeRRa were evaluated following thermodynamic considerations. The chemical equilibrium calculation was achieved through the Thermo-Calc software [Citation26] with the SGTE substance database [Citation27]. The maximum temperature and total pressure were set to 1100 K and 1 atm, respectively. Considering that the partial pressure of B vapor species, temperature, and their variation during transport influence the chemical forms and state of B compounds, the B2O3/H2O ratio and temperature were set as parameters.
3. Results and discussion
Firstly, the temperature conditions at which B chemistry changed drastically in the experiments are identified from the distribution of B in the TGT and aerosol filter measured by ICP–MS. The B chemistry is discussed in detail for each temperature region based on results from the characterization of deposits and airborne aerosol particles, and results of chemical equilibrium calculations. Finally, the effects of B on Cs and I chemistry are discussed based on the result in this study, with thermodynamic consideration.
3.1. Distribution of boron amount
shows the distribution of the amounts of B in deposits in the TGT and aerosol filters from ICP–MS analyses of soluble and insoluble samples. The highest amount of B was deposited above 1000 K, with 60–70% of the deposits comprising insoluble compounds. Since compounds in the H-B-O system like B2O3 and HBO2 are soluble [Citation28], the formation of insoluble compounds potentially results from the reaction of B with SS. Contrarily, the B deposits in the TGT (from 1000 K to 400 K) were limited, with a small peak of the B amount in deposits at about 700 K. The airborne materials had the highest amount of B deposited on the aerosol filter at about 400 K. Since most deposits in the TGT and aerosol particles transported downstream of the TGT were dissolved, the behaviors are primarily attributed to the condensation of vapors in the H-B-O system (boric acid vapors).
3.2. Deposits in the high-temperature region (above 1000 K)
shows the XRD patterns for the SS surface at various temperatures in the TGT. The reference peaks for SS, Fe3O4, and 2FeO · FeBO3 are also shown in . Only the SS displays peaks below about 970 K, whereas peaks from Fe3O4 and the product of B and SS 2FeO · FeBO3 appear above 1010 K. The formation of Fe3O4 indicates that the condition of this experiment was similar to the representative one during an SA because Fe3O4 was formed also in the core of Three Mile Island Unit 2 [Citation29]. Regarding the formation of 2FeO · FeBO3, the peak intensity at about 17.2 deg. and 34.8 deg. derived from 2FeO · FeBO3 decreased as temperature increased. This result is consistent with results of B distribution above 1000 K in : the B amounts in the insoluble deposits containing 2FeO · FeBO3 decreased as temperature increased above 1000 K. exhibits the microstructure of the SS surface from SEM for about 1040 K and about 970 K. The microstructures of specimens at 1040 K significantly differ from those at 970 K, with a unique acicular structure. This difference is attributed to the formation of 2FeO · FeBO3 on the surface of the SS as demonstrated by the XRD results, since this acicular structure is missing in the microstructure of Fe3O4 [Citation30]. These results indicate that B reacted with the SS during transportation, forming 2FeO · FeBO3 on the surface of the SS above about 1000 K.
Figure 5. XRD patterns for the SS surface at various positions (temperature) in the TGT and reference peaks of SS, Fe3O4, and 2FeO · FeBO3
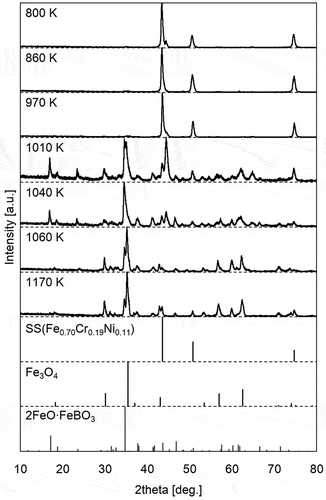
Figure 6. Microstructure of the SS surface from SEM: (a) −50 mm (about 1040 K), (b) 50 mm (about 970 K)
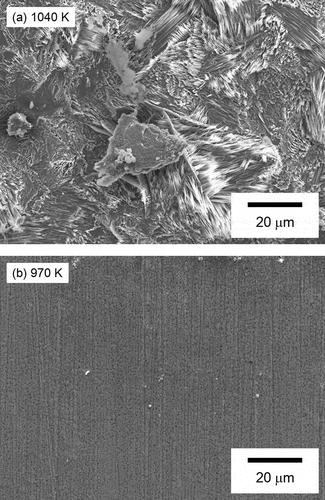
The chemical reaction mode discussed relies on the results from chemical equilibrium calculation for stable compounds at various temperatures and the B2O3/H2O ratio in . At temperatures above 1000 K, the calculations reveal the possibility of B existing as condensed B2O3 or as boric acid vapors like (HBO2)3, depending on the B2O3/H2O ratio. In this experiment, soluble deposits occurred above 1000 K as in , and these possibly are mainly composed of B2O3. It is inferred from these results that the chemical reaction mode for the formation of 2FeO · FeBO3 involves be two pathways: the liquid-solid reaction between liquid B2O3 and solid SS and/or the gas-solid reaction between boric acid vapors and solid SS.
3.3. Airborne materials and deposits in thermal gradient tube (below 1000 K)
3.3.1. Airborne materials
shows the particle size (PSL equivalent diameter) distribution at each temperature as measured by the on-line optical particle counter. The particle size distributions at each temperature are likely to be lognormal. Fine particles displayed a peak around 0.2 μm for a temperature of 1000 K. In addition to fine particles around 0.2 μm, the number of particles larger than 0.3 µm in diameter increased at around 700 K, without further change in the size distribution as the temperature decreased to 400 K. The microstructure of airborne aerosol particles trapped at 400 K is shown in , with the particles displaying cubic or rectangular morphology. In addition to the primary particles below 1 µm, some fine particles agglomerated on the surface of primary particles. This result suggests that particles containing B grow instantly to a primary particle during transportation, causing condensed fine particles to agglomerate in the lower temperature region. These results indicate that the growth of particles containing B was enhanced and saturated at around 700 K under the test conditions.
Figure 8. Distribution of polystyrene latex (PSL) equivalent diameter at each temperature measured by the on-line optical particle counter
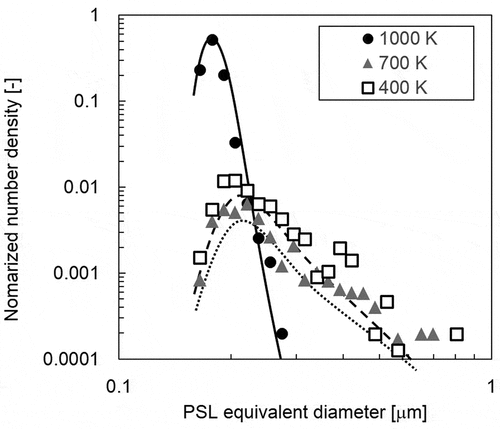
The formation mechanism of particles is discussed based on the results from chemical equilibrium calculations in . The results below 1000 K indicate that B compounds take condensed B2O3 aerosol particles or boric acid vapors like (HBO2)3 depending on the B2O3/H2O ratio in the relatively high-temperature region, whereas condensed HBO2 aerosol particles were most stable in the relatively low-temperature region. The boundary temperature for HBO2 condensation decreased with decreasing B2O3/H2O ratio. These results indicate that boric acid vapors like (HBO2)3 start condensing during transportation to HBO2 aerosol particles below 650 K. This temperature corresponds approximately to that at which the growth of particles containing B was enhanced and saturated (). Thus, the enhancement of particle growth originates from the condensation of boric acid vapors to aerosol particles.
3.3.2. Deposits
shows the microstructures of deposits at 700 K, corresponding to the temperature at which a small peak of the B amount in deposits is observed in . The deposits are characterized by two types of particles with diameters of about 5 μm and below 0.2 μm (arrowed in ). The appearance of the larger one resembles solidified liquid. As described earlier, the condensation of boric acid vapors likely started already in this temperature region. Thus, the deposition in this temperature region is probably caused by the condensation of boric acid vapors onto the SS, where the temperature is slightly lower than in the gas phase due to the thermal gradient of the tube. Although the sizes of smaller deposits (<0.2 μm) correspond to that of major airborne aerosol particles in , the sizes of larger deposits in are much higher than that of airborne aerosol particles. Thus, the large deposits likely originate from the repeated agglomeration of condensed boric acid vapors, thereby minimizing the surface area of the deposits.
3.4. Possible effects of boron on the cesium and iodine chemistry in an a severe accident
Previous studies on the transport behavior of B with integral B4C control rod degradation tests indicate that released B is transported as boric acids like HBO2 and H3BO3, and is deposited in a lower temperature region like RCS outlet around 423K as a form of aerosol particles [Citation15–17]. The results of this study provide an additional explanation for the behaviors noted in the previous studies and new knowledge on the reaction with SS. The new information on B chemistry in this study is presented in . In this section, the possible effects of B on the Cs and I chemistry are discussed based on the results in this study related to detailed B chemistry and thermodynamic considerations.
Table 2. Boron chemistry during transportation
3.4.1 Effects of boron deposits
The results of this study suggest that significant amounts of the released B were deposited above 1000 K, with part of the deposits forming insoluble compounds via reaction with SS. These results indicate that released B vapors from an SA possibly remain in a Reactor Pressure Vessel (RPV) through reaction with SS and deposition as low volatile B2O3 at high temperatures.
These B deposits possibly affect the Cs chemistry, since it is well known that Cs vapors like CsOH react with SS and adhere to the surface of SS (Cs chemisorption behavior) [Citation31–33]. This behavior was vital in the evaluation of Cs distribution in the RPV of 1F NPS, with the separator and steam dryer exhibiting the larger surface areas. The Cs chemisorption was caused by the reaction of Cs vapors with precipitated Si oxide on the SS surface [Citation32–34]. Silicon is one of the minor ingredient elements in SS, and Si oxide is formed by oxidation of SS. Regarding the effects of B on the chemisorption behavior of Cs, the stability of Cs-Si-O compounds in co-existence with B was estimated based on the results of the chemical equilibrium calculations [Citation34]. The results indicate a minor effect of B on the chemisorption behavior of Cs, since the Cs-Si-O compounds are mostly stable compounds. In contrast, the results of this study demonstrate significant enhancement of the reaction between B compounds and SS, e.g., a significant change in surface microstructure to an acicular structure possibly containing B at high temperature. Therefore, the formation of B compounds likely affects the surface oxidation of SS, producing the changes in precipitation of Si oxide on the surface. Therefore, the B reaction with SS possibly affects the chemisorption behavior of Cs. However, even if the chemisorption of Cs on SS is suppressed, a possibility is that Cs vapors react with B deposits on SS and Cs is retained in the high-temperature region as shown below.
The possibility of the deposited B to retain Cs vapors was also assessed based on chemical equilibrium calculations using the Thermo-Calc software [Citation26] with the SGTE substance database [Citation27]. shows the fraction of Cs compounds in the Cs-I-O-H system and Cs-I-O-H with B system at various temperatures. The calculation condition allowed determination of the amount of Cs, I, B, O, and H based on the SA condition of 1F-NPS [Citation13,Citation35]. In the absence of B, Cs compounds exist as CsI and CsOH vapor species above 1200 K but start to condense at 1200 K. Contrarily when B is present, the temperature at which Cs starts to condense is much higher. Thus, the presence of B likely produces low volatile compounds like CsBO2. This result indicates that some Cs is retained by the reaction of CsOH vapor with such B2O3 deposits in the higher temperature region.
Figure 11. Mole fraction of Cs compounds in the Cs-I-O-H system (a) and the Cs-I-O-H with B system (b) at different temperatures
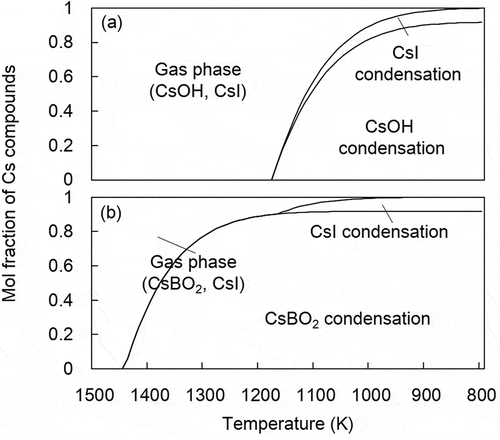
These results further indicate that although the B deposits in the high-temperature region likely influence the chemisorbed amounts of Cs on SS, the Cs amounts in the RPV are probably underestimated in a BWR SA without consideration of the presence of B deposits in the SA analysis.
3.4.2 Effects of boron vapors
The results in this study incorporating thermodynamic considerations show that the chemical forms of B transported below 1000 K are B2O3 vapor species and boric acid vapor species including (HBO2)x and H3BO3 in the high-temperature region, with condensation due to supersaturation (<700 K: (HBO2)x and H3BO3). This behavior probably causes deposition in the lower temperature region, showing consistency with observations from previous integral tests like Phebus-FPT [Citation22] and Quench 07 [Citation23].
Regarding the reaction of Cs, I, and B in the gas phase, the results of previous involving thermodynamic considerations indicate the possibility of enhancement of the formation of gaseous iodine through the gaseous reaction between CsI and B vapors [Citation13,Citation24]. This phenomenon occurs if CsI and B vapors co-exist during transportation to the lower temperature region. A previous heating test of CsI using TeRRa showed that deposition of CsI is characterized by a peak of around 700 K [Citation9]. Although deposition temperatures vary by the concentration of vapor species (partial pressure of vapor species), the deposition temperature of boric acids like HBO2 is close to that of CsI. Thus, from the results of this study, the reaction among Cs, I, and B in the gas phase likely occurred above temperatures where B2O3 and boric acid were supersaturated and deserve consideration in evaluating the source term.
The reaction of B with CsI deposits according to previous single effect tests possibility causes re-vaporization of Cs and I from deposits. It may also produce gaseous iodine, not only through the reaction of B deposits [Citation25] but also from B vapors [Citation36]. As described earlier, since the deposition temperature of boric acids like HBO2 could resemble that of CsI, the re-vaporization of Cs and I by the reaction with B vapors occurs during transportation to the lower temperature region and therefore requires consideration for the evaluation of the source term, namely the generation of gaseous iodine.
These results demonstrate that the formation of gaseous iodine likely occurs in a BWR SA through the gas-solid phase reaction between condensed CsI and B vapors, in addition to the gaseous reaction between CsI and B vapors during transportation.
4. Conclusions
Boron chemistry during transportation was experimentally investigated using the dedicated experimental apparatus named TeRRa in a steam atmosphere to assess B transportation in an SA of the BWR and its effect on Cs and I in the high-temperature region.
In addition to knowledge on B transportation in the low-temperature region around 400 K reported from previous integral experiments, the findings on B chemistry in the high-temperature region that emerged in this study are:
The released B was deposited onto SS above 1000 K by condensation.
Released B notably reacted with SS above 1000 K and formed 2FeO · FeBO3. This is likely caused by the liquid-solid reaction between liquid B2O3 deposits and the solid SS, and/or the gas-solid reaction between B vapors like (HBO2)x and the solid SS.
Boron vapor species transported to the lower temperature region are predicted to include (HBO2)x and H3BO3 based on thermodynamic considerations. These vapors condensed on SS around 700 K under conditions at which their partial pressure attains the saturated vapor pressure.
These results indicate that released B from a degraded BWR control blade in BWR SA remains in the high-temperature region like the RPV by the formation of products like 2FeO · FeBO3 on SS and through deposition of volatile B2O3.
The possible effects of B on Cs and I chemistry were inferred from the results of this study based on the detailed transport behavior of B with thermodynamic considerations:
The existence of B deposits in the high-temperature region potentially produces low volatile compounds like CsBO2 through the reaction of B deposits and Cs vapors. Therefore, the Cs amount in the RPV is likely underestimated in a BWR SA, when SA analysis fails to incorporate the presence of B deposits.
The formation of gaseous iodine via the gas-solid phase reaction mode of condensed CsI and B vapors likely occurs under BWR SA in addition to the gaseous reaction between CsI and B vapors during transportation.
Thus, the B chemistry in this study suggests that additional consideration is needed for the accurate evaluation of the Cs and I source term.
Acknowledgments
The authors thank Dr. Kunihisa Nakajima for helpful comments during the preparation of this manuscript. They also express gratitude to Mr. Juntaro Takada and Mr. Kunihiro Sumiya for their support on the experiments reported in this study.
Disclosure statement
No potential conflict of interest was reported by the authors.
Correction Statement
This article has been republished with minor changes. These changes do not impact the academic content of the article.
References
- Schwinges B, Journeau C, Haste T, et al. Ranking of severe accident research priorities. Prog Nucl Energy. 2010 January;52(1):11–18.
- Klein-Heßling W, Sonnenkalb M, Jacquemain D, et al. Conclusions on severe accident research priorities. Ann Nucl Energy. 2014 Dec;74:4–11.
- Suehiro K, Sugimoto J, Hidaka A, et al. Development of the source term PIRT based on findings during Fukuhsima Daiichi NPPs accident. Nucl Eng Des. 2015 May;286:163–174.
- Kajimoto M, Muramatsu K, Watanabe N, et al. Development of THALES-2, a computer code for coupled thermal-hydraulics and fission product transport analyses for severe accident at LWRs and its application to analysis of fission product revaporization phenomena. Proc. Int. Topical Mtg. on Safety of Thermal Reactors; 1991 Jul 21-25; Portland (US).
- Ujita H, Satoh N, Naitoh M, et al. Development of severe accident analysis code SAMPSON in IMPACT project. J Nucl Sci Technol. 1999 Nov;36:1076–1088.
- Gauntt RO, Cole RK, Erickson CM, et al. MELCOR computer code manuals: primer and user’s guide version 1.8.5. US: Sandia National Laboratories; 2005. (Report no. NUREG/CR-6119).
- Miwa S, Yamashita S, Ishimi A, et al. Research program for the evaluation of fission product and actinide release behaviour, focusing on their chemical forms. Ener Proc. 2015 May;71:168–181.
- Osaka M, Nakajima K, Miwa S, et al. Results and progress of fundamental research on FP chemistry. Proc. ERMSAR-2017; 2017 May 16-18; Warsaw (Poland).
- Miyahara N, Miwa S, Nakajima K, et al. Development of experimental and analytical technologies for fission product chemistry under LWR severe accident condition. Proc. WRFPM-2017; 2017 Sep 10-14; Jeju Island (Korea).
- Miyahara N, Miwa S, Horiguchi N, et al. Chemical reaction kinetics dataset of Cs-I-B-Mo-O-H system for evaluation of fission product chemistry under LWR severe accident conditions. J Nucl Sci Technol. 2019;56(2)228–240.
- Miwa S, Miyahara N, Nakajima K, et al. Development of fission product chemistry database ECUME for the LWR severe accident, Proc. 27th International Conference on Nuclear Engineering (ICONE27), ICONE27-1993, 2019 May 20-23; Tsukuba, (Japan).
- McFarlane J, Wren JC, Lemire RJ. Chemical speciation of iodine source term to containment. Nucl Technol. 2002 May;138:162–178.
- Miwa S, Yamashita S, Osaka M. Prediction of the effects of boron release kinetics on the vapor species of cesium and iodine fission products. Prog Nucl Energy. 2016 March;92:254–259.
- Gauntt RO, Humphries LL. Final Results of the XR2-1 BWR metallic melt relocation experiment. NUREG/CR-6527. Washington DC, USA: Nuclear Regulatory Commission; 1997.
- Hofmann P, Markiewicz ME, Spino JL. Reaction behavior of B4C absorber material with stainless steel and zircaloy in severe light water reactor accidents. Nucl Technol. 1989;90(2):226–244.
- Barrachin M, de Luze O, Haste T, et al. Late phase fuel degradation in the Phébus FP tests. Ann Nucl Energy. 2013 May;61:36–53.
- Haste T, Payot F, Dominguez C, et al. Study of boron behaviour in the primary circuit of water reactors under severe accident conditions: A comparison of Phebus FPT3 results with other recent integral and separate-effects data. Nucl Eng Des. 2011 August;246:147–156.
- Shibata H, Tokushima K, Sakamoto K, et al. Control blade degradation test under temperature gradient in steam atmosphere, Proc. Top Fuel 2016; 2016 Sep 11-15; Boise (US).
- Gauntt RO, Cash JE, Cole RK, et al. MELCOR computer code manuals. NUREG/CR-6119, 2005. Rev.3. SAND2005-5713. Vol. 1 & 2. Albuqucrque, New Mexico, USA: Sandia National Laboratories; 2005.
- Krauss W, Schanz G, Steiner H Tg-rig Tests (Thermal Balance) on the Oxidation of B4C, 2003, Forschungszentrum Karlsruhe GmbH, Karlsruhe, Germany. FZKA 6883.
- Imoto J, Miwa S, Osaka M Experimental investigation on boron oxidative vaporization processes from zirconium-boron and iron-boron alloys in a high temperature steam atmosphere, Proceedings of International Topical Workshop on Fukushima Decommissioning Research (FDR2019), FDR2019-1062, 2019 May 24-26; Fukushima, (Japan).
- Haste T, Payot F, Bottomley PDW. Transport and deposition in the Phebus FP circuit. Ann Nucl Energy. 2013 November;61:102–121.
- Homann C, Hering W, Schanz G. Analysis and comparison of experimental data of bundle tests QUENCH-07 to QUENCH-09 about B4C control rod behaviour, 2006, Forschungszentrum Karlsruhe GmbH, Karlsruhe, Germany. FZKA 7101.
- Minato K. Thermodyanmic analysis of cesium and iodine behavior in severe light water reactor accidents. J Nucl Mater. 1991;185:154–158.
- Kalilainen J, Kärkelä T, Zilliacus R, et al. Chemical reactions of fission product deposits and iodine transport in primary circuit conditions. Nucl Eng Des. 2014 February;267:140–147.
- Sundman B, Jansson B, Andersson J-O. The Thermo-Calc databank system. CALPHAD. 1985;9(2):153–190.
- Dinsdale AT. SGTE data for pure elements. CALPHAD. 1991;15:317–425.
- Schweitzer GK, Pesterfield LL. The Aqueous Chemistry of the Elements. New York: Oxford University Press; 2010.
- Olsen C, Jensen S, Carlson E, et al. Materials interaction and temperatures in the Three Mile Island Unit 2 Core. Nucl Technol. 1989;87:57–94.
- Cheng X, Jiang Z, Wei D, et al. High temperature oxidation behaviour of ferritic stainless steel SUS 430 in humid air. Met Mater Int. 2015 March;21(2):251–259.
- Allen GC, Bowsher BR, Dickinson S, et al. Surface studies of the interaction of cesium hydroxide vapor with 304 stainless steel. Oxid Met. 1987 August;28(1–2):33–59.
- Di Lemma FG, Nakajima K, Yamashita S, et al. Surface analyses of cesium hydroxide chemisorbed onto type 304 stainless steel. Nucl Eng Des. 2016 August;305:411–420.
- Di Lemma FG, Nakajima K, Yamashita S, et al. Experimental investigation of the influence of Mo contained in stainless steel on Cs chemisorption behavior. J Nucl Mater. 2017 February;484:174–182.
- Di Lemma FG, Yamashita S, Miwa S, et al. Prediction of chemical effects of Mo and B on the Cs chemisorption onto stainless steel. Energy Procedia. 2017;127:29–34.
- Nishihara K, Iwamoto H, Suyama K, Estimation of fuel compositions in fukushima-daiichi nuclear power plant, JAEA-Data/Code 2012-018, 2012.
- Sato I, Onishi T, Tanaka K, et al. Influence of boron vapor on transport behavior of deposited CsI during heating test simulating a BWR severe accident condition. J Nucl Mater. 2015 June;461:22–28.