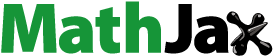
Abstract
We demonstrate the efficient transfer of population from the upper to the lower inversion level within the rotational ground state of para-ND. A packet of ND
molecules is velocity controlled and state selected using a Stark decelerator, and subsequently transferred with
efficiency using the principles of adiabatic rapid passage. Transitions are induced using microwave chirps both under zero-field conditions as well as in the presence of an electric field to probe
-resolved transition efficiencies. Simulations of the transfer efficiencies based on the von Neumann equation, taking all hyperfine transitions into account, showed excellent agreement with experimental observations.
GRAPHICAL ABSTRACT
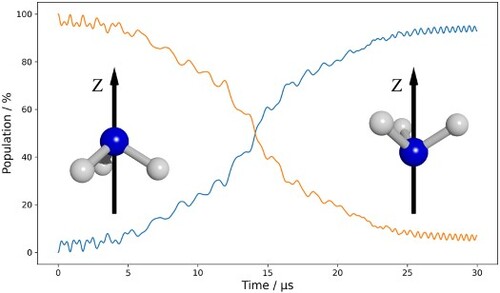
1. Introduction
Ammonia is a molecule with a rich history in the physical sciences. In the chemical community the role of the Haber–Bosch process to convert nitrogen into ammonia for the production of fertiliser is well recognised. In the physics community ammonia is most renowned for its use in the original molecular beam maser (Microwave Amplification by Stimulated Emission of Radiation) setup. Proposed by Townes's group in 1951, it was first supposed to work with ND but was realised in 1954 with NH
instead [Citation1,Citation2]. The development of the maser is of particular historic importance because it laid the foundation of all laser (Light Amplification by Stimulated Emission of Radiation) technology, with the first laser realised in 1960 by Theodore Maiman [Citation3]. In astrophysics, ammonia is of pivotal importance because it was not only the first molecule to be observed with microwave spectroscopy in the laboratory in 1934 [Citation4], but also was the first polyatomic molecule observed in space in 1968 [Citation5], whereas ND
was the first triply deuterated species identified in the interstellar medium in 2002 [Citation6]. Observed emissions from rotational transitions are frequently used to probe the temperature of molecular clouds [Citation7]. More recently, ammonia has become a molecule of primary importance in cold molecule research, as its pronounced first-order Stark effect allows for easy control in electric fields [Citation8]. Decelerators [Citation9], bunchers [Citation10], traps [Citation11–16], guides [Citation17–19], storage rings [Citation20] and synchrotrons [Citation21] have been demonstrated using ND
, and controlled samples of ammonia have been used in novel collision experiments [Citation22–26] and for high resolution spectroscopy [Citation13] alike.
Owing to its general importance, ammonia and its isotopologues are well studied spectroscopically. In ammonia, each rotational level is split into two inversion components of opposite parity. In the present study we will investigate completely deuterated ammonia, which is easier to manipulate in electric fields compared to its non-deuterated isotopologue, related to the different magnitude of the inversion splittings [Citation8]. For the rotational ground state of para-ND
, the splitting between the upper component (with negative parity referred to as
) and the lower component (
) amounts to ≈1.6 GHz. For
ND
and
ND
, especially in their electronic ground state, one finds a wealth of spectroscopic studies and the molecular constants derived from these studies can be used to accurately describe the energy levels of the system [Citation13,Citation27–34]. In addition, the spectroscopic constants of ammonia can be found in the cologne database for molecular spectroscopy (CDSM) [Citation35–37], together with many other molecules.
Like in the original beam maser, experiments that manipulate beams of ammonia with electric fields typically select only molecules that reside in a specific quantum state, as the apparatus is designed to transmit only those molecules that experience the appropriate Stark shift. In an electric field, the and
components of the rotational ground state split according to the projection quantum number
. The
components within the
and
levels are low and high-field seeking, respectively, i.e. molecules in the
(
) state gain (lose) Stark energy when entering an electric field. In contrast, the
components in first order do not experience any Stark shift. Therefore, ND
molecules in the
state are typically selected, and prepared as a starting point for further experimental studies.
Yet, for these subsequent experiments, it might be desirable for the experimentalist to change the quantum state to any other state of choice. For instance, transfer of molecules to the high-field seeking state might be of interest to produce a sample of molecules in their absolute ground state that can be loaded in AC traps [Citation12,Citation14–16]. For collision studies, transfer to
gives access to an initial state with different parity, which generally has a large influence on collision cross sections [Citation38].
Population transfer between two components of an inversion doublet in ND is straightforward by applying microwave radiation at a frequency near 1.6 GHz for
ND
or 1.4 GHz for
ND
, as has been routinely done in a number of previous experiments studying the trapping of high-field seeking states [Citation12–16]. These studies achieved transfer efficiencies limited to around
, which the authors state is due to the width of the applied microwave pulse and the extent of the hyperfine structure. Generally, the maximum transfer efficiencies are dictated by the specific implementation of the microwave fields and the initial beam populations. E.g. in the case of incoherent isotropic radiation, starting with a single state populated in a two-level system, the limit should be
, as stimulated emission and stimulated absorption have the same probability. The remaining population in the
state represents a pure loss in the desired
density, and can cause severe background levels from competing collision pathways in collision experiments.
Here we demonstrate transfer of inversion doublet populations in the rotational ground state of para-
ND
with
transfer efficiency using coherent linearly polarised microwave radiation and the principles of adiabatic rapid passage (ARP, see, e.g. Ref. [Citation39–41]). A beam of ND
is passed through a Stark decelerator to produce samples of
state-selected ND
molecules with near perfect state purity. Transitions are induced both under field-free conditions and in the presence of an external electric field to obtain
-resolved efficiencies for population transfer into the
state. We use single frequency microwave pulses as well as microwave chirps to address all possible transitions (574 hyperfine transitions in the zero-field case). A theoretical description of population transfer for microwave excitation that takes all hyperfine transitions into account is presented and we find good agreement with experimentally obtained transfer efficiencies.
Our motivation behind driving population from the state into the
state after state separation is twofold. Ammonia, with its relatively large dipole moment, has interesting properties for collision studies at collision energies below ≈1 cm
. Of particular interest are experiments to elucidate the role of the anisotropic dipole-dipole interaction in these collisions and/or experiments that apply external electric fields to control and manipulate collision pathways. As the molecule's dipole moment is either oriented along (↑) or against (↓) the direction of the electric field depending on the quantum state, ND
(
) + ND
(
) collisions probe repulsive (
) dipole-dipole interactions, whereas ND
(
) + ND
(
) collisions are governed by attractive (
) dipole-dipole interactions in the presence of an electric field. A second motivation lies in the prospects of merging two state-selected packets of ND
to obtain the lowest collision energies possible. Merging can be achieved by using a curved electrostatic hexapole that bends the trajectory of one ND
beam into another ND
beam's path. Clearly, this is not possible for two ND
beams with molecules that are all in the
state, as manipulation of one beam's path will necessarily also affect the other, setting a fundamental limit to the phase-space overlap of both beams according to Liouville's theorem [Citation42]. As molecules in the
state experience a Stark shift that is much less than for molecules in the
levels, transfer of ND
in one of the beams into the
state may allow for the merging of both packets, circumventing this fundamental obstacle.
To date, the principle of ARP is successfully employed in a variety of research areas. ARP is of current interest in the microwave spectroscopic community and employed for example in the studies of 2-hexanone [Citation43], benzonitrile [Citation44], epifluorohydrin [Citation45] and in general in experiments investigating a phenomenon called strong field coherence breaking [Citation46], a tool used to ease up the assignment if multiple species are contributing to a recorded spectrum. ARP has been used before in experiments where efficient switching between states is desired. Recent examples include the investigation of population transfer between rotational states of CO molecules on a chip [Citation47] using THz radiation, and the manipulation of population in vibrational states in incident beams of methane for molecule-surface scattering experiments using infrared lasers [Citation48].
2. Experimental
The experiments were performed in an apparatus schematically shown in Figure . Details on Stark decelerator and detector are described elsewhere [Citation38,Citation49]. A gas mixture of ND
in Xenon was expanded through a Nijmegen Pulsed Valve (NPV) [Citation50] at typical backing pressures of
, creating a rotationally cooled molecular beam. A skimmer was placed 13 cm from the NPV exit to select the central part of the beam. The beam was subsequently loaded into a
long Stark decelerator, operating at ±15 kV in the s = 3 guiding mode for
[Citation8]. A pair of cylindrical rod electrodes of opposite charge was placed about
downstream of the decelerator exit, directed along the Stark decelerator axis but offset by
on the vertical axis. These so-called deflector electrodes with
length and
diameter were charged with opposite voltages of ±10 kV to create an electric field gradient perpendicular to the decelerator axis. The deflector electrodes were used to create an (inhomogeneous) electric field to study
-resolved microwave transitions, and to have molecules in
states deflected by about
from the beam axis at the detection region as illustrated in Figure .
Figure 1. Schematic overview of the experimental set-up, consisting of: (1) the last stage of the 2.6 m long Stark decelerator, (2) a microwave antenna pointing along the Stark axis, (3) a pair of deflector electrodes, (4) an ionisation laser and an electrode stack with MCP to detect the formed ions. The distance between various components are given in mm.
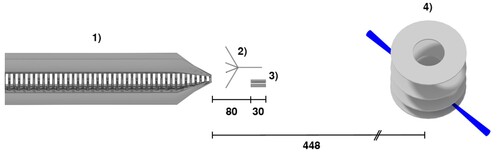
Figure 2. Illustration of the functional principle of the deflector electrodes. Depicted are trajectories of ND molecules in the
(blue),
(red), and
(green) states. If
the molecules get deflected from the beam axis.
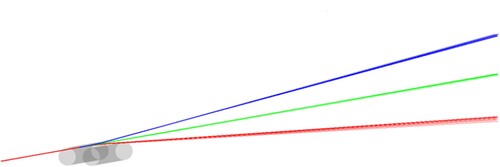
Microwaves were provided using Rohde&Schwarz SMA103B signal generators with chirp functionality, and pulsed operation. The signal generators were connected to custom made monopole antennae -- a simple piece of wire with four radials (four additional wires of similar length that are connected to the coaxial ground). For most of the experiments an antenna inside of the vacuum chamber was used, which was placed close to the exit of the Stark decelerator. For experiments investigating the preparation of a pure
beam, a second antenna outside of the chamber was also used. This second antenna was placed in front of a glass view-port localised vertically above the exit of the Stark decelerator.
The ND molecules are state-selectively detected
from the exit of the Stark decelerator using a (2+1) Resonance Enhanced Multi-Photon Ionization (REMPI) scheme employing a tunable pulsed dye laser, following well-known REMPI-transitions in deuterated ammonia [Citation51]. Molecules in the
(
) state are probed via 2-photon resonant transitions to the v = 5 (v = 4) level of the B state using a wavelength of
(
). The ions are subjected to electric fields that accelerate them towards a micro-channel plate (MCP) detector. The MCP response was recorded using a PicoScope 5444D oscilloscope. The repetition rate of the experiment is
.
3. Computational
Coherent phenomena can be described by the time-dependent Schrödinger equation. Related to this equation but able to track the population of multiple states at once is the von Neumann equation in the interaction picture:
(1)
(1)
The density matrix
contains the populations of the levels as diagonal elements. The time is denoted as t, with t = 0 representing the start of the excitation pulse. The rovibrational Hamiltonian
contains the energies of the levels as diagonal elements. The matrix
is used to transform into the interaction frame. It contains a reference energy level
on all diagonal elements for the lower energy levels. Typically the lowest energy level of the system is chosen for this purpose. For all upper energy levels the diagonal elements are
with ω being the circle-frequency of the radiation. The transition dipole matrix
contains all transition dipole moments, and ϵ represents the amplitude of the raditation field. The notation
is the commutator. The dimension of the matrices in this equation is equal to the number of levels involved in the transition scheme, i.e. neglecting non-involved states.
For our computations we retrieve as well as
using Pickett's calpgm suite of programs [Citation52] (also referred to as SPFIT/SPCAT). These programs, among many other useful codes, are available on the PROSPE website (www.ifpan.edu.pl/ kisiel/prospe.htm) [Citation53]. The initial spectroscopic parameters required as input for SPFIT/SPCAT are taken from the CDSM database and we add the hyperfine and fine structure terms derived from Ref. [Citation34] to get a complete Hamiltonian of the system. The transition dipole matrix has to be converted according to the interaction picture:
(2)
(2)
We make the assumption that all molecules experience the same field. The electric field function is defined as a cosine with the phase set to zero for convenience. For ARP calculations
(3)
(3)
will depend on time with the start frequency
and the sweep rate
. During the chirp, the electric field of the radiation is given by
(4)
(4)
where an integration is required since ω varies with time. For chirps the formulation of the von Neumann equation is
(5)
(5)
However, each time step the frame changes -- since
changes, too. Thus, each time step
(6)
(6)
must be also transformed from the old frame (old) to the new frame (new).
We have now laid out the equations required for our predictions. By simply replacing the infinitesimal changes in the von Neumann equation with finite changes Δ we arrived at suitable Python3 scripts. Time steps of typically
were used and simulating
only takes a few minutes (on an i7-1050H processor @
). In the zero-field chirp predictions of
ND
described later the error introduced by using this size of time step is about
in the value of the predicted population transfer.
To investigate the effects of single frequency pulses and chirps on state populations we first discuss a relatively simple 10 level system belonging to the OH radical for demonstrative purposes. This is done in Section 3.1 for its electronic ground state () and hyperfine states with total angular momentum F = 2. After this exploration we will proceed to understand the predictions of the more involved 144 level system of
ND
in the
states in Section 3.2. The influence of external electric fields on the hyperfine levels of ND
and population transfers will be shortly visited in Section 3.3.
3.1. The OH radical
As a consequence of the unbalanced electron angular momentum of the ground state of OH, the energy levels are split into so called lambda doublets of even or uneven symmetry. The microwave lambda doublet transitions of the OH radical in the
states, which we will discuss as a simple system for demonstrative purposes here, were experimentally well investigated in Ref. [Citation54]. For each lambda doublet component of the levels with F = 2 there are five possible states distinguished by their projection quantum number
. This means, the system is composed of 10 levels. Using linear polarised light outside of electric bias fields introduces the selection rule
. Also, for
the transition dipole moment happens to be zero for the pure lambda doublet transitions, such that this state can not be addressed this way. The transition dipole moment
is related to its reduced matrix elements
via Wigner 3j symbols (based on Equation 5.4.1 of [Citation55])
(7)
(7)
with the reduced matrix elements
being provided by SPFIT/SPCAT. The parameters 1 and q in the Wigner 3j symbol give the total angular momentum and projection of angular momentum of the radiation. A photon is a spin 1 particle, which explains the value of 1 in the symbol. The parameter q is the projection quantum number, representing the polarisation, and can have values of
, corresponding to left handed circular polarised light, Z-polarised light, and right handed circular polarised light, respectively. The dipole moment of OH is
. For the states of the OH radical considered here and using q = 0, Equation (Equation7
(7)
(7) ) gives transition dipole moments
of
for
,
for
, and
for
. It is important to note that the transition dipole moment is exactly twice as high for
compared to
. For resonant excitations the frequency with which two level system populations oscillate, the so called Rabi frequency Ω, is proportional to the transition dipole moment,
. This means the Rabi frequency for
is exactly twice as large as for
and the corresponding fractions of population oscillate with
and
, respectively. Starting with equal populations of all
states of the upper doublet component this frequency relation in population oscillations, together with the fact that the
state is not addressed, gives a quantum mechanical limit of how much transfer can be achieved with resonant excitations:
(8)
(8)
To push the population transfer beyond the limit of
, one can make use of an ARP. To make use of this phenomenon the radiation is first tuned far from resonance. Then the frequency is slowly changed over time so that it first becomes resonant and then detunes in the opposite direction to where it started. This is called a chirp pulse or simply chirp. If the detuning rate is slow and powers are not too high, the population transfer becomes independent of Rabi oscillations, and robust population transfer between states is achieved. Results for predictions of the OH radical system with realistic pulse settings are provided in Figure . Since all possible transitions are addressed this way, the limit in population transfer for the OH radical is increased to
only limited by the population of the
states. So clearly, chirps have advantages in comparison to single frequency pulses to achieve an inversion of population.
Figure 3. Prediction for the upper state populations (orange) and lower state populations (blue) of the OH lambda doublet transition as a function of time. Equal population of all five
states of the upper lambda doublet levels is assumed at the beginning of the pulse. Single frequency pulses resonant at
, chirps from
to
. Predictions made for a power density of
.
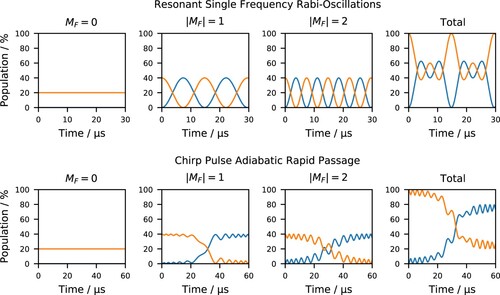
3.2. The ND
system
Having established the general benefits of ARP on the simpler 10 level system of the OH radical, we will now investigate the effects on the more involved 144 level inversion transitions of ND
.
The different angular momenta (or spins) apparent in the molecule of ammonia couple to give a total angular momentum . Each state can be characterised by quantum numbers associated with these angular momenta, and since we will be addressing the states using these quantum numbers, a brief explanation of each of them is given here:
J, total angular momentum excluding nuclear spin
.
K, projection of
on the symmetry axis of ammonia
(z-axis, with x, y, z referring to the molecule fixed frame)
, projection of
on the electric field axis
(Z-axis, with X, Y, Z referring to the laboratory frame)
, nuclear spin of nitrogen
, nuclear spin of deuterium
, combined nuclear spins of the three deuterium atoms
, coupled angular momenta
with
F, total angular momentum
with
, projection of
on the electric field axis
First the deuterium spins are combined to give the total spin of the deuterium atoms [Citation56]. Then
is coupled with
to yield
which is subsequently coupled with
to yield
.
The increased complexity, including all possible hyperfine states, offers benefits as well as downsides. On the one hand, all levels are linked via transitions to states of the other inversion component. So, compared to the OH system discussed above, there is no limit to the total population transfer through states not addressed by the radiation. On the other hand, the system is very complex with a total of 574 transitions addressed in one chirp. Some of these will first move population from to
while others cause a back transfer at later timings during the chirp. Also, some of the transitions share target levels, which will limit the possible transfer. To see how exactly these effects balance and what population transfer is to be expected we carried out numerical simulations based on the von Neumann equation. The results, based on the assumption of starting with equal population in each hyperfine component of
, are displayed in Figure . In the left part of the figure we see that about
transfer can be achieved. To illustrate the temporal evolution of the population in a single hyperfine component, the right part of the figure shows the population of the
state of the
levels as a function of time. It is seen that the population of this state does not approach zero during the microwave pulse. Despite the complexity of the system large transfers are predicted and the calculated time evolution of the population resembles that shown in an early study of NH
[Citation40].
Figure 4. Predictions of the inversion transition of the
system. Chirp from 1587.050 to
assuming equal population of all 72 levels of the upper (−) inversion component at the beginning of the pulse. A power density of
was used in the simulations. (a) Total population in all hyperfine components within the
(orange) and
(blue) levels as a function of time. (b) Population of the
component of
as a function of time.
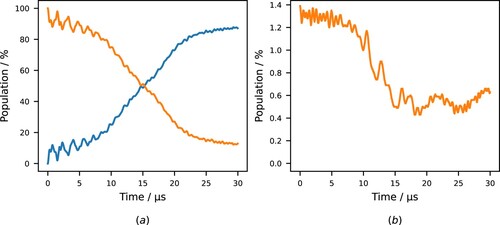
3.3. ND
in external electric fields
The transfer schemes described above did not discriminate between states. Working in external electric fields allows for investigations of
specific population transfer schemes. These external electric fields cause a mixing of zero-field eigenstates and shifts in energy levels, which can be calculated by diagonalisation of the total Hamiltonian
(9)
(9)
Here
is the transition dipole matrix in the basis of the zero-field eigenfunctions of
. This matrix can be calculated from reduced moments (provided by SPFIT/SPCAT) utilising Equation (Equation7
(7)
(7) ) with q = 0. The electric field is denoted as E. The resulting shifts in energy levels in dependence of E were calculated in Ref. [Citation34]. We repeated these calculations, of which the results are given in Figure . It should be mentioned, that our electric field values scale by a factor of
compared to Ref. [Citation34], which is related to a transfer error in the ordinate in Ref. [Citation34].
Figure 5. Stark shifts of hyperfine levels in dependence of electric field on two different scales. At energy levels belonging to the
and
manifold of
show avoided crossings. At high fields the nuclear spins decouple, such that
becomes a good quantum number.
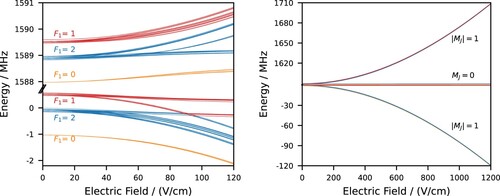
One experiment that we will investigate in Section 4.2 is to transfer as much population as possible from states to
. Predictions of such transfers in electric fields are more involved than their zero-field counterparts. On the one hand, the mixing of zero-field eigenstates has to be taken into account, causing changes in the transition dipole matrix as well as the Hamiltonian. On the other hand the electric field provides a quantisation axis and, dependent on the orientation of the radiation, different values of q in Equation (Equation7
(7)
(7) ) have to be taken into account. In our experiments, the molecules coming from the Stark decelerator should be purely in
states. To illustrate the quantum mechanical limitations in population transfer, two exemplary calculations were carried out at a constant field of
. Both started with a pure
population of
. One chirp was tailored to move population to the
states of
using X-polarised light (mix of q = 1 and q = −1), while the other was designed to move population to the
states of
employing Z-polarised light (q = 0). In both cases a
bandwidth chirp and a power density of
were utilised. The results are shown in Figure . It can be seen that, given the appropriate orientation of the radiation and the correct detuning from zero-field resonance, it is possible to populate only specific
states. The transfers from
to
states (
) is limited to less than
, which is related to the unbalanced number of origin (48 hyperfine state for
of
) and target states (24 for
of
). Transfers with balanced number of origins and targets, from
to
states (
), still work with high efficiency, with 97% for this specific simulation.
Figure 6. Predictions of population transfers inside of a electric field starting with a pure low-field seeking population of
of
with
(
) in orange (blue). From left to right: (a) Energy level diagram with approximate resonant transition frequencies. (b) Population transfer curve for a
chirp running over the
resonance using X-polarised radiation. (c) Population transfer curve for a
chirp running over the
resonance using Z-polarised radiation.
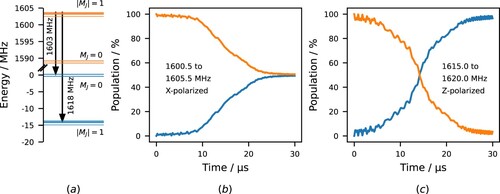
4. Results and discussion
This section describes the results of experiments that were carried out to investigate population transfer between specific quantum states of ND. Three different types of experiments were conducted. In Section 4.1, transitions were performed in zero-field after the ND
packet exits the decelerator. In Section 4.2, transitions to specific
states were induced inside of a fringe electric field by keeping on the voltages applied to the decelerator upon exit of the molecular beam. Finally, in Section 4.3 we investigated the
composition of produced packets of ND
(
) by performing pump-probe experiments employing an additional microwave pulse further downstream.
4.1. Transitions in zero-field
Before investigating population transfer, we first investigated the quantum state composition of ND molecules exiting the decelerator. By virtue of the Stark effect, the decelerator only captures molecules within the
state. In the default operation of a Stark decelerator, the last electrodes are switched off when the packet of molecules exits the decelerator. Since
is not a good quantum number in zero-field, we assume that the ND
molecules are then equally redistributed over all states, i.e. we assume a statistical redistribution over the hyperfine states upon switching off the decelerator. The
microwave spectrum was recorded by applying
pulses shortly after the molecules exit the decelerator, while the resulting
population was probed by the laser. The recorded spectrum is shown by the blue line in Figure (a). It is seen that all nitrogen
hyperfine structure peaks appear in the spectrum, and deuterium hyperfine splittings are partially resolved. The expected spectrum based on the von Neumann equation was calculated (black line) and, except for the first peak corresponding to the
transition, matches the experimental spectrum very well.
Figure 7. Spectra recorded by stepping single frequency pulses, starting at
and ending at
with step size of
. Signal recorded on the
(
) REMPI resonance in orange (blue). Each peak is labelled by the
quantum numbers of the initial and final states involved in the transition. (a) 100 samples per step. Spectrum recorded with Stark decelerator in normal operation together with prediction (black) using the von Neumann equation at a power of −6 dBm for the inside antenna (corresponding to a power density of
). (b) 50 samples per step. Spectrum recorded with extended fringe field of the Stark decelerator and using the outside antenna radiating through a glass view-port into the machine at
(about
). (c) 50 samples per step. Spectrum recorded using a first pump pulse (CP_CROSS in Table ) with the inside antenna at
in the fringe field between the end of the Stark decelerator and the deflector electrodes, followed by a probe pulse at
after the molecules passed the deflector. In (b) and (c) each peak is additionally labelled with
to show the high-field behaviour of the initial state's population (see Figure ).
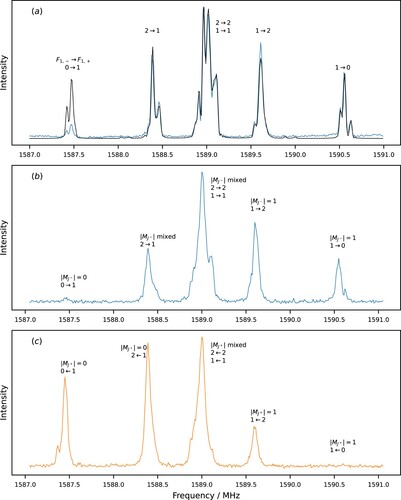
Table 1. Population transfer in electric fringe field of decelerator and deflector.
Referring back to Figure , the manifold exclusively corresponds to
states that are barely influenced by electric fields. Hence, this state is not populated inside the decelerator, but can only gain population when the molecules leave the decelerator and the fields are abruptly switched off. The observed spectroscopic signature of
are evidence that these so-called Majorana transitions [Citation57] occur, but not in a statistical fashion. This implies that our assumption of a complete scrambling of all hyperfine components when the fields are switched off is not entirely valid. To further investigate this effect, we tried to prevent Majorana transitions by leaving the last electrode pair on when the molecules exit the decelerator. The molecules will then not experience the fast switching of high electric fields to zero, and are expected to adiabatically follow the slowly decreasing fringe field of the decelerator while remaining in their quantum state. To record the microwave spectrum under these conditions, we used the second microwave antenna placed outside of the vacuum, radiating through a glass view-port when the molecules are some
downstream from the exit of the decelerator where the fringe field was reduced to negligible (<0.1 V/cm) values.
The recorded spectrum is shown in Figure (b). The first peak has now completely vanished from the spectrum, demonstrating that the
hyperfine component remains unpopulated if ND
molecules adiabatically follow a decreasing electric field. The resolution in the observed spectrum is slightly reduced compared to the spectrum shown in panel (a). This is most likely caused by the non-ideal coupling of the microwave radiation to the molecular beam, with no direct line of sight between the microwave antenna and the beam, and a more confined region of the vacuum chamber resulting in an inhomogeneous field over the
time of interaction.
The efficiency of microwave induced population transfer was then investigated by using the in-vacuum antenna close to the exit of the decelerator, and by operating the Stark decelerator in default mode, i.e. the fields are switched off when the molecules exit. In our experiments, the
population was probed using two different methods. In the first, the
population was simply calculated from the measured
depletion, assuming that all depletion in signal corresponds to population that is moved to
levels and that
was not populated at the beginning of the pulse. Both approximations should be valid. In the second, we measured the
population directly by tuning the REMPI laser to the appropriate transition. We corrected for the difference in REMPI efficiency between the
and
states by use of calibration measurements with a beam of known population. The population of both states should be approximately equal when the Stark decelerator was switched off throughout and the beam propagated through the decelerator in free flight, providing us with a beam to calibrate on. The
signal was generally weaker than
for the same population and a factor of 1.44(9) (the
error is given in parentheses in units of the last significant digit and is 0.09) was determined, to convert
to equivalent
signal. For larger population transfers the absolute signal in
decreases, while it increases for
. Since the statistical fluctuations are directly correlated with the absolute signal, for larger transfers these fluctuations are much smaller for
. All in all, the method to probe population transfer by measuring depletion of the
population was considered the most accurate, and was used where possible. The method of deriving transfer by measurements on
signal will be important again in Section 4.2, where transfers inside of electric fields are investigated and the main source of
depletion is deflection from the molecular beam axis. The
population can then no longer be directly associated with
signal depletion.
The experimental result for a bandwidth
pulse ranging from 1587.050 to
is shown together with predictions in Figure (a). The time development of ND
population in the
state was probed by stopping the chirp at various timings. Excellent agreement between experiment and prediction is obtained with slightly higher population transfers observed experimentally. This can be explained by the assumption of equal population in all hyperfine components of the
state at the start of the pulse, that is made in the theoretical calculations. As discussed above, not all hyperfine components in the
states are equally populated when the decelerator is switched off, causing a preferred population of some states over others. These deviations will generally cause a slight increase in the population transfer.
Figure 8. Population transfer between the two inversion components of ND
for a
chirp from 1587.050 to
. Population of
in orange and of
in blue.
on signal generator corresponds to ≈50 W/m
in power density. Experimental and theoretical results are represented by solid and dashed lines, respectively. (a) As a function of time at
calculating
population from
depletion with a step size of
and 250 samples per step. (b) As a function of power on the signal generator, here the
population was derived measuring and converting the
signal. Each step 1000 samples were used for
as well as
signal. Error bars are
.
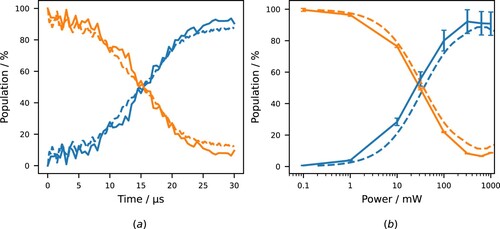
We then varied the power of the chirp to observe the corresponding change in population transfer shown in Figure (b). Again, theory and experiment agree well, while the experimentally observed transfer is slightly higher than predicted. The highest transfer was achieved at the second to last data point with , and it amounts to
. At the highest power that was experimentally accessible, which is
, the transfer was slightly smaller with
. The decrease in transfer with increasing power after a certain threshold is also expected theoretically. In the high power limit, power broadening would become so pronounced that the chirp would, at any frequency, always address the complete hyperfine structure. This would be in violation with the working principles of ARP, and cause decrease in the expected transfer.
The results of these experiments demonstrate that even in the complicated 144 level system of the inversion doublet of deuterated ammonia, population transfer exceeding
is possible using the principle of ARP. Experiments of this kind can also be used to determine the power density of the microwave radiation field in the region of the molecular beam, by matching theory with experiment. In our case dialling up
on the signal generator corresponds to ≈50 W/m
, utilising the antenna inside the chamber.
In many experiments on deuterated ammonia found in the literature the isotopologue ND
is used. To test if the principles described here can also be used on this system, we carried out a few experiments on molecular beams of
ND
, chirping over the respective
transition. Since
N does not undergo quadrupole coupling the hyperfine structure extends over a much smaller region. We found that a chirp of
from 1430.0 to
with a relatively small power density corresponding to
yields a transfer of
. Predictions suggest, however, that if the power is reduced and the chirp duration and bandwidth are increased, larger transfers should be achievable, in agreement with expectations on ideal conditions for the ARP. Such long durations of more than
might be, however, impractical in some experiments. Since the principles are all the same, we will focus on
ND
for the remainder of this article. For more detail on the short explorations into
ND
population transfers, the reader is referred to the supplementary material.
4.2. Transitions in electric fields
The microwave transitions can also be addressed inside of an electric field to populate specific -components. Referring back to Figure , at fields above a few
, the energy levels are grouped according to the quantum number
, i.e.nuclear spin coupling becomes a relatively small perturbation with respect to the Stark energies. Figure shows that the theoretically predicted transfer efficiency for population transfer from the
into the
states is limited to
. As mentioned in Section 3.3, this fundamental limit originates from the unbalanced number of origin (48) and target states (24). By contrast, more than
transfer efficiency should be achievable for the
transition.
Unfortunately, well-defined homogeneous electric fields and a well-defined microwave polarisation are not accessible in our current experimental setup. Yet, we can still probe -resolved population transfer using a combination of the fringe field of the Stark decelerator, and the field introduced by the deflector electrodes. The resulting Stark shifts caused by the (inhomogeneous) field were mapped out using short microwave single frequency pulses at different offset frequencies. The start of the microwave pulse was given a variable delay to probe the Stark shifted transition. The observed Stark shift as a function of delay time is given in Figure (a). The detuning and time axes can be translated into distance and electric field, as shown in Figure (b). It is seen that the electric field is strongly inhomogeneous, and passes through a minimum of
detuning corresponding to
.
Figure 9. (a) Stark shift induced by the fringe fields of the Stark decelerator and deflector electrodes as a function of time of flight from the geometrical end of the Stark decelerator. Black line marks the frequency for resonant transitions. Red line marks the transitions for
. The five different pulses, that were tested for their performance are shown. The performance of these pulses is compared in Table . (b) Time of flight and detuning were translated into electric field in dependence of distance from the Stark decelerator.
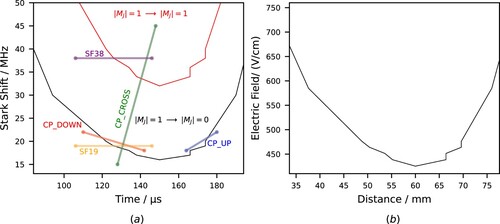
Knowing the Stark shifts, we can devise experiments to transfer ND molecules from the
state into either or both
components of the
state. We used several single frequency pulses (SF) or chirps (CP), and tested their ability to transfer population. In our setup, the deflector electrodes are designed to only transmit molecules with
, and remove molecules that reside in the
or
states. This configuration has the objective to produce a packet of ND
in the
state with as little remaining population in the
state as possible – as is beneficial for a collision experiment for instance –, but different configurations with different objectives can be engineered as well.
The resulting populations in the and the
states, as detected by the laser after the beam passed the deflector electrodes, are given in Table . Populations in both the
and
states were probed by measuring REMPI signals with and without microwave pulses, and the signal intensities were related to the initial population in
without deflector or microwave signal generators in operation. It appears that a cross chirp (CP_CROSS), first running through the
resonance and later through the
resonance, going from 1604.05 to
in the course of
, has the best performance both in
formation as well as in depletion of residual
population. However, a simple single frequency pulse at
detuning (SF19) with a duration of
performs similarly well. The single frequency pulses inside of the electric fields still exploit ARP, since the electric field gradient drags the transition frequencies through resonance over time.
Referring back to Figure , the maximum transfer efficiency with the deflector in operation to deflect all molecules in is limited to less than
. The reported values in Table are clearly below this limit. The deflector will still cause some losses in the
populations by the second order Stark effect, which makes the ND
molecules in these states slightly high-field seeking. To probe whether we can in principle reach the
quantum mechanical transfer limit, an experiment was carried out using the SF19 pulse, which energetically can only address the transition to
. For this purpose the deflector was switched off a few microseconds later than the end of the SF19 pulse. The molecules are then still transferred inside a sizable electric field, but experience no significant deflection. With this configuration, a total transfer of
was achieved, in good agreement with the expected limit of
. The residual population in
, however, is then not reduced.
4.3. Pump-probe experiments to asses beam purity
It is noted that since only states should pass the deflector without being deflected from the beam axis, the
and
population detected by the laser should be almost exclusively in
. To experimentally verify this, we performed a pump-probe experiment using the in-vacuum antenna to transfer population to the
state using the CP_CROSS pulse, and then induced the
transition after the deflector using the second antenna in front of the view-port. The deflector was turned off just before the ND
molecules arrived in the second microwave region to remove residual fringe fields. The resulting
microwave spectrum is shown in Figure (c). It is seen that the first peak, which originates from the
manifold, is now pronounced in the spectrum. As seen in Figure this state has predominant
character, with a region of avoided crossings at around
. Transitions from the
also occur, though these states are adiabatically only connected to
states. We suspect that running over the region of avoided crossings in the decreasing electric fields still introduces Majorana transitions between
and
states. This implies, that a pure
character can not be maintained when propagating through regions of zero-field. By contrast, the outermost peak probing the
is completely missing. This state has pure
character, and its missing spectroscopic signature testifies the formation of a pure beam of ND
(
) inside of the fringe electric fields.
Based on these observations and Figure , some general statements with regards to preservation should be pointed out, similar to the conclusions described in studies involving ND
before [Citation34,Citation58]. These statements are based on the assumption of redistribution within and between states of the
and
manifold of
as well as redistribution within states of the
manifold of
:
When exposing the molecular beam to regions of zero-field,
it is not possible to prepare and maintain
beams of
that keep their character.
it is possible to prepare and maintain
beams of
that keep their character, if exclusively the
states are populated.
it is possible to prepare and maintain
beams of
that keep their character, if exclusively the
states are populated.
it is possible to prepare and maintain
beams of
that keep their character, if exclusively the
states are populated.
5. Conclusions
This study demonstrates how to achieve highly efficient population transfers between the upper () and lower (
) inversion components of the
rotational ground state of para-ND
. A beam of ND
is velocity controlled and state selected in the
state by a Stark decelerator, and transferred to the
state by driving the
microwave transition using the principle of adiabatic rapid passage. When the transition is made under zero-field conditions, we produce a sample of ND
of mixed
and
character in the
state, with transfer efficiencies of
. Transfer experiments were also performed inside the inhomogeneous fringe field of the Stark decelerator to produce packets of ND
that exclusively reside in the
levels. These were then further purified using a set of deflector electrodes to remove molecules in unwanted quantum states from the beam. A theoretical description of population transfer was developed based on the von Neumann equation taking all 574 hyperfine transitions into account, which described the experimentally obtained transfer efficiencies well.
Efficient population transfer using adiabatic rapid passage as demonstrated here -- either without or inside of an electric field -- may find interesting applications in spectroscopic investigations, state-resolved collision experiments, merged beam approaches or trapping experiments using high-field seeking molecules. Our investigations were performed in an existing apparatus with limited optical access and space, compromising the ability to apply controlled microwave radiation patterns and electric field geometries. New designs that incorporate dedicated sections for inducing microwave transitions in well controlled and homogeneous electric fields may further enhance our ability to produce samples of -resolved ND
(
) molecules with near-perfect transfer efficiency and quantum state purity.
Supplementary Material
Download PDF (252.5 KB)Acknowledgements
S. H. would like to thank B. Sartakov for the detailed exchange regarding the Stark effect in ammonia and is grateful to H. S. P. Müller for providing initial SPFIT/SPCAT files for ND including nitrogen quadrupole coupling. We thank Niek Janssen and André van Roij for expert technical support.
Disclosure statement
No potential conflict of interest was reported by the author(s).
Additional information
Funding
References
- J.P. Gordon, H.J. Zeiger and C.H. Townes, Phys. Rev. 99 (4), 1264–1274 (1955). doi:10.1103/PhysRev.99.1264
- D. Lainé, in Advances in Electronics and Electron Physics, edited by L. Marton (Academic Press Limited, London, 1976), pp. 183–251.
- T.H. Maiman, Nature 187 (4736), 493–494 (1960). doi:10.1038/187493a0
- C.E. Cleeton and N.H. Williams, Phys. Rev. 45 (4), 234–237 (1934). doi:10.1103/PhysRev.45.234
- A.C. Cheung, D.M. Rank, C.H. Townes, D.D. Thornton and W.J. Welch, Phys. Rev. Lett. 21 (25), 1701–1705 (1968). doi:10.1103/PhysRevLett.21.1701
- F.F.S. van der Tak, P. Schilke, H.S.P. Müller, D.C. Lis, T.G. Phillips, M. Gerin and E. Roueff, Astron. Astrophys. 388 (3), L53–L56 (2002). doi:10.1051/0004-6361:20020647
- C.M. Walmsley and H. Ungerechts, Astron. Astrophys. 122, 164–170 (1983). https://ui.adsabs.harvard.edu/abs/1983A&A...122..164W
- S.Y.T. van de Meerakker, H.L. Bethlem, N. Vanhaecke and G. Meijer, Chem. Rev. 112 (9), 4828–4878 (2012). doi:10.1021/cr200349r
- H.L. Bethlem, F.M.H. Crompvoets, R.T. Jongma, S.Y.T. van de Meerakker and G. Meijer, Phys. Rev. A 65 (5), 053416 (2002). doi:10.1103/PhysRevA.65.053416
- F.M.H. Crompvoets, R.T. Jongma, H.L. Bethlem, A.J.A. van Roij and G. Meijer, Phys. Rev. Lett. 89 (9), 093004 (2002). doi:10.1103/PhysRevLett.89.093004
- H.L. Bethlem, G. Berden, F.M.H. Crompvoets, R.T. Jongma, A.J.A. van Roij and G. Meijer, Nature 406 (6795), 491–494 (2000). doi:10.1038/35020030
- J. van Veldhoven, H.L. Bethlem and G. Meijer, Phys. Rev. Lett. 94 (8), 083001 (2005). doi:10.1103/PhysRevLett.94.083001
- J. Veldhoven, Ph. D. thesis, Radboud Universiteit Nijmegen, 2006.
- H.L. Bethlem, J. van Veldhoven, M. Schnell and G. Meijer, Phys. Rev. A 74 (6), 063403 (2006). doi:10.1103/PhysRevA.74.063403
- M. Schnell, P. Lützow, J. van Veldhoven, H.L. Bethlem, J. Küpper, B. Friedrich, M. Schleier-Smith, H. Haak and G. Meijer, J. Phys. Chem. A 111 (31), 7411–7419 (2007). doi:10.1021/jp070902n
- P. Lützow, M. Schnell and G. Meijer, Phys. Rev. A 77 (6), 063402 (2008). doi:10.1103/PhysRevA.77.063402
- S.A. Rangwala, T. Junglen, T. Rieger, P.W.H. Pinkse and G. Rempe, Phys. Rev. A 67 (4), 043406 (2003). doi:10.1103/PhysRevA.67.043406
- S. Chervenkov, X. Wu, J. Bayerl, A. Rohlfes, T. Gantner, M. Zeppenfeld and G. Rempe, Phys. Rev. Lett. 112 (1), 013001 (2014). doi:10.1103/PhysRevLett.112.013001
- B. Bertsche and A. Osterwalder, Phys. Rev. A 82 (3), 033418 (2010). doi:10.1103/PhysRevA.82.033418
- F.M. Crompvoets, H.L. Bethlem, R.T. Jongma and G. Meijer, Nature 411 (6834), 174–176 (2001). doi:10.1038/35075537
- C.E. Heiner, D. Carty, G. Meijer and H.L. Bethlem, Nat. Phys. 3 (2), 115–118 (2007). doi:10.1038/nphys513
- L.P. Parazzoli, N.J. Fitch, P.S. Żuchowski, J.M. Hutson and H.J. Lewandowski, Phys. Rev. Lett. 106 (19), 193201 (2011). doi:10.1103/PhysRevLett.106.193201
- Z. Gao, J. Loreau, A. van der Avoird and S.Y.T. van de Meerakker, Phys. Chem. Chem. Phys. 21 (26), 14033–14041 (2019). doi:10.1039/C8CP07109H
- X. Wu, T. Gantner, M. Koller, M. Zeppenfeld, S. Chervenkov and G. Rempe, Science 358 (6363), 645–648 (2017). doi:10.1126/science.aan3029
- V. Zhelyazkova and S.D. Hogan, J. Chem. Phys. 147 (24), 244302 (2017). doi:10.1063/1.5011406
- J. Jankunas, B. Bertsche, K. Jachymski, M. Hapka and A. Osterwalder, J. Chem. Phys. 140 (24), 244302 (2014). doi:10.1063/1.4883517
- P. Helminger and W. Gordy, Phys. Rev. 188 (1), 100–108 (1969). doi:10.1103/PhysRev.188.100
- P. Helminger, F.C.D. Lucia and W. Gordy, J. Mol. Spectrosc. 39 (1), 94–97 (1971). doi:10.1016/0022-2852(71)90280-3
- G.D. Lonardo and A. Trombetti, Chem. Phys. Lett. 84 (2), 327–330 (1981). doi:10.1016/0009-2614(81)80356-9
- S.N. Murzin, Opt. Spektrosc. 59, 725 (1985).
- L. Fusina, G. di Lonardo and J.W.C. Johns, J. Mol. Spectrosc. 112 (1), 211–221 (1985). doi:10.1016/0022-2852(85)90205-X
- L. Fusina, M. Carlotti, G.D. Lonardo, S.N. Murzin and O.N. Stepanov, J. Mol. Spectrosc. 147 (1), 71–83 (1991). doi:10.1016/0022-2852(91)90169-B
- L. Fusina and S.N. Murzin, J. Mol. Spectrosc. 167 (2), 464–467 (1994). doi:10.1006/jmsp.1994.1250
- J. van Veldhoven, R.T. Jongma, B. Sartakov, W.A. Bongers and G. Meijer, Phys. Rev. A 66 (3), 032501 (2002). doi:10.1103/PhysRevA.66.032501
- C.P. Endres, S. Schlemmer, P. Schilke, J. Stutzki and H.S.P. Müller, J. Mol. Spectrosc. 327, 95–104 (2016). doi:10.1016/j.jms.2016.03.005
- H.S.P. Müller, F. Schlöder, J. Stutzki and G. Winnewisser, J. Mol. Struct. 742 (1–3), 215–227 (2005). doi:10.1016/j.molstruc.2005.01.027
- H.S.P. Müller, S. Thorwirth, D.A. Roth and G. Winnewisser, Astron. Astrophys. 370 (3), L49–L52 (2001). doi:10.1051/0004-6361:20010367
- G. Tang, M. Besemer, T. de Jongh, Q. Shuai, A. van der Avoird, G.C. Groenenboom and S.Y.T. van de Meerakker, J. Chem. Phys. 153 (6), 064301 (2020). doi:10.1063/5.0019472
- C. Liedenbaum, S. Stolte and J. Reuss, Phys. Rep. 178 (1), 1–24 (1989). doi:10.1016/0370-1573(89)90018-5
- T.L. Weatherly, Q. Williams and F. Tsai, J. Chem. Phys. 61 (7), 2925–2928 (1974). doi:10.1063/1.1682434
- J.U. Grabow, in Handbook of High-resolution Spectroscopy, edited by M. Quack and F. Merkt (John Wiley & Sons, Ltd, Weinheim, 2011), Chap. 1, pp. 723–799.
- J. Liouville, J. Math. Pures Appl., 3, 342–349 (1838).
- S.M. Fritz, P. Mishra and T.S. Zwier, J. Chem. Phys. 151 (4), 041104 (2019). doi:10.1063/1.5109656
- D. Schmitz, V.A. Shubert, T. Betz and M. Schnell, J. Mol. Spectrosc. 280, 77–84 (2012). doi:10.1016/j.jms.2012.08.001
- G.G. Brown, B.C. Dian, K.O. Douglass, S.M. Geyer and B.H. Pate, J. Mol. Spectrosc. 238 (2), 200–212 (2006). doi:10.1016/j.jms.2006.05.003
- A.O. Hernandez-Castillo, C. Abeysekera, B.M. Hays and T.S. Zwier, J. Chem. Phys. 145 (11), 114203 (2016). doi:10.1063/1.4962505
- G. Santambrogio, S.A. Meek, M.J. Abel, L.M. Duffy and G. Meijer, ChemPhysChem 12 (10), 1799–1807 (2011). doi:10.1002/cphc.201001007
- J. Werdecker, M.E. van Reijzen, B.J. Chen and R.D. Beck, Phys. Rev. Lett. 120 (5), 053402 (2018). doi:10.1103/PhysRevLett.120.053402
- J. Onvlee, S.N. Vogels, A. von Zastrow, D.H. Parker and S.Y.T. van de Meerakker, Phys. Chem. Chem. Phys. 16 (30), 15768–15779 (2014). doi:10.1039/C4CP01519C
- B. Yan, P.F.H. Claus, B.G.M. van Oorschot, L. Gerritsen, A.T.J.B. Eppink, S.Y.T. van de Meerakker and D.H. Parker, Rev. Sci. Instrum. 84 (2), 023102 (2013). doi:10.1063/1.4790176
- M. Ashfold, R. Dixon, R. Stickland and C. Western, Chem. Phys. Lett. 138, 201–208 (1987). doi:10.1016/0009-2614(87)80368-8
- H.M. Pickett, J. Mol. Spectrosc. 148 (2), 371–377 (1991). doi:10.1016/0022-2852(91)90393-O
- Z. Kisiel, in Spectroscopy from Space, edited by J. Demaison et al. (Kluwer Academic Publishers, Dordrecht, 2001), pp. 91–106.
- E.R. Hudson, H.J. Lewandowski, B.C. Sawyer and J. Ye, Phys. Rev. Lett. 96 (14), 143004 (2006). doi:10.1103/PhysRevLett.96.143004
- A.R. Edmonds, Angular Momentum in Quantum Mechanics (Princeton University Press, Princeton, 1996).
- H.M. Pickett, J. Mol. Spectrosc. 228 (2), 659–663 (2004). doi:10.1016/j.jms.2004.05.012
- E. Majorana, Il Nuovo Cimento 9 (2), 43–50 (1932). doi:10.1007/BF02960953
- E.W. Steer, L.S. Petralia, C.M. Western, B.R. Heazlewood and T.P. Softley, J. Mol. Spectrosc. 332, 94–102 (2017). doi:10.1016/j.jms.2016.11.003