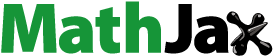
Abstract
Production and subsequent reactions of singlet oxygen are a major problem for the safe storage and transportation of foodstuffs. The reactions of singlet oxygen with cholesterol, a molecule commonly found in cells in vivo and also widely found in foodstuffs, are well known experimentally, with the products of these reactions commonly observed. However, a detailed understanding of the reaction mechanisms and transition states and intermediates involved are less well understood. In the current study, we have employed density functional theory to investigate the nature of the reaction pathways of singlet oxygenand cholesterol, determining the transition states and free energies of reaction. We have identified epoxide-like intermediates in the formation of 5-hydroperoxycholesterol and S-6-hydroperoxycholesterol which may be involved in the formation of 5,6-epoxide derivatives of cholesterol, which are an indicator of liver function. These results have given more detailed insight into the reaction of singlet oxygen with cholesterol.
Keywords:
1. Introduction
Photooxidation of natural unsaturated molecules, especially those found in foodstuffs, is a major issue for global food security. In the type II mechanism, a photosensitiser absorbs UV or visible light, and the excited singlet state undergoes intersystem crossing to a triplet electronic state, after which energy transfer occurs from the photosensitiser to O2, leading to singlet oxygen (1Δg) generation. Singlet oxygen then reacts with unsaturated C = C bonds, often forming hydroperoxyl moieties. Various vitamins act as photosensitisers, leading to generation of singlet oxygen, including vitamin A, riboflavin (vitamin B2) and vitamin E, among many others [Citation1–5]. Many vitamins which are commonly cited as anti-oxidants, such as B3, D2, D3 and E, were found to generate singlet oxygen when pre-irradiated with UVB, followed by UVA [Citation2]. Riboflavin-sensitised reactions have been investigated in different foodstuffs, including model fatty acids, bulk oil and milk [Citation3–5]. Where singlet oxygen is generated in vivo, damage to the cell leading to long-term health effects can occur. A recent study by Yeo and Shahidi [Citation6] investigated the possible mechanism of development of cardiovascular diseases from photooxidation of low-density lipoprotein particles, with riboflavin acting as the sensitiser [Citation6]. Oxidation of cholesterol has been also been linked to cardiovascular disease. The central nervous system is particularly rich in cholesterol [Citation7], and oxysterol derivatives have been linked to neurodegenerative diseases, including Alzheimer’s disease [Citation8], amyotrophic lateral sclerosis (also known as motor neurone disease) [Citation9,Citation10] and Lewy body dementia [Citation11] via modification of important proteins. The presence of 5,6-epoxide derivatives of cholesterol has been detected at ppm levels in human blood and appears to be related liver function [Citation12]. Singlet oxygen also reacts with surrounding solvent, to produce hydrogen peroxide [Citation13].
There have been several inhibitors suggested to prevent singlet oxygen damage, including β-carotene [Citation6,Citation14] (and carotenes more generally [Citation15–21]). β-carotene was shown to quench singlet oxygen when present in human low-density lipoprotein particles at concentrations larger than 10 µM, although at lower concentrations β-carotene acted as a photosensitiser, generating singlet oxygen [Citation6]. The main mechanism of singlet oxygen quenching by carotenoids is via energy transfer [Citation22,Citation23], although chemical quenching leading to carotene endoperoxides is also possible [Citation24–26]. Acetazolamide, previously thought to generate singlet oxygen leading to type II photooxidation reactions [Citation27–30], has been shown to act as a singlet oxygen quencher [Citation31]. Sabat et al. demonstrated the utility of tauroursodeoxycholic acid as a singlet oxygen quenching species in a model POPC membrane, using both experimental techniques and molecular dynamics simulations [Citation32].
Type II photooxidation of cholesterol and related molecules (e.g. vitamin D3) have been widely studied experimentally [Citation33,Citation34] and reaction mechanisms proposed on the basis of the observed products (Figure ), but a detailed mechanistic investigation using theoretical methods has yet to be performed. In the current study, we investigate the reactions of singlet oxygen with cholesterol, commonly found in the central nervous system, more generally in cells in vivo and also in foodstuffs, detailing the reaction mechanisms using density functional theory (DFT).
2. Computational details
DFT was used, with the ωB97X-D functional [Citation35] and 6-311G(d) basis set [Citation36] to identify stationary points on the reaction pathways of singlet oxygen with cholesterol in the gas phase and in a solution comprised of 12 molar% water and 88 molar% acetone, giving a dielectric constant of 27.624, to match typical experimental conditions for singlet oxygen reactions [Citation37]. We chose the ωB97X-D functional as it excels for a range of different chemical phenomena, including barrier heights [Citation38], while the basis set was chosen as the ωB97X-D functional was developed with a Pople triple-zeta plus polarisation basis set (with additional diffuse and polarisation functions); we used 6-311G(d) as a good compromise between accuracy as a triple-zeta plus polarisation basis set and computational efficiency. Solution phase calculations were performed with the conductor-like polarisable continuum model (C-PCM) [Citation39,Citation40], as implemented in the Q-Chem 5.4 package. The PCM solvent approach has previously been successfully employed for investigations of solution phase singlet oxygen reactivity [Citation41–43]. Stationary points were confirmed as minima or transition states using vibrational frequency calculations. Intrinsic reaction pathway [Citation44–46] calculations were used to confirm that a transition state connected the ‘reactant’ and ‘product’ of a given reaction. Spin-restricted Kohn–Sham calculations were performed in all cases. All calculations were performed with the Q-Chem software [Citation47]. Free energy differences are reported herein, where translational, rotational and vibrational enthalpy and entropy terms are taken from the vibrational frequency calculations at 298.15 K. Sample input files are given in the Supporting Information.
The 1Δg electronic state of O2 is formally doubly-degenerate, with spin-paired electrons residing in one (or other) of the two degenerate π* orbitals. Due to the SCF process, these orbitals are no longer degenerate, as one is doubly occupied and the other remains unoccupied; however, this is still a good approximation to the 1Δg electronic state.
Rates of reaction were calculated with the Eyring equation:
3. Results and discussion
3.1 Cholesterol reactions
The reaction profile for addition of singlet oxygen to carbon position 5 of cholesterol is shown in Figure (with the reaction mechanism in Scheme 1), and to position 6 in Figures and (R- and S-enantiomers, respectively; reaction mechanisms given in Schemes 2 and , respectively). The molecular structures are given in Figure (formation of 5-hydroperoxycholesterol), Figure (formation of R-6-hydroperoxycholesterol) and Figure (formation of R-6-hydroperoxycholesterol) and in Figures S1–S3 in the Supporting Information for the corresponding gas phase results. For both the formation of the 5-hydroperoxycholesterol and S-6-hydroperoxycholesterol, the ‘ene’ reaction proceeds via two transition states, with the C–O bond forming first, followed by removal of a hydrogen atom from a C–H bond to form the O-H bond and leading to migration of the C = C double bond. Formation of the first transition state on the 5-hydroperoxycholesterol pathway is 1.8 kJ mol−1 lower than the corresponding transition state along the S−6-hydroperoxycholesterol pathway. The intermediate structures for both pathways are very similar, both exhibiting epoxide-like structures (see Schemes S1 and S3). The intermediate on the 5-hydroperoxylcholesterol pathway has the second oxygen atom pointing towards the hydrogen atom connected to carbon position 7 (Figure (b)), while the second oxygen atom on the S-6-hydroxyperoxylcholesterol pathway intermediate points towards the hydrogen atom connected to carbon position 4 (Figure (b)) and is 7.3 kJ mol−1 more favourable than the 5-hydroxyperoxyl intermediate.
Figure 2. Reaction energy profile for the reaction of cholesterol and 1O2 to produce 5-hydroperoxycholesterol (see Figure ). Molecular structures of transition states and intermediates are given in Scheme 1 and Figure . All values are relative to the previous step.
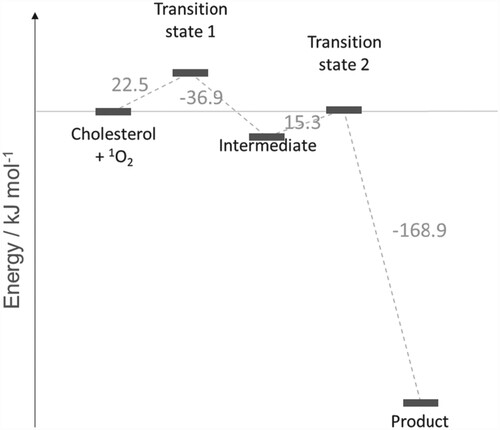
Figure 3. Reaction energy profile for the reaction of cholesterol and 1O2 to produce R-6-hydroperoxycholesterol (see Figure ). Molecular structures of transition states and intermediates are given in Scheme 2 and Figure . All values are relative to the previous step.
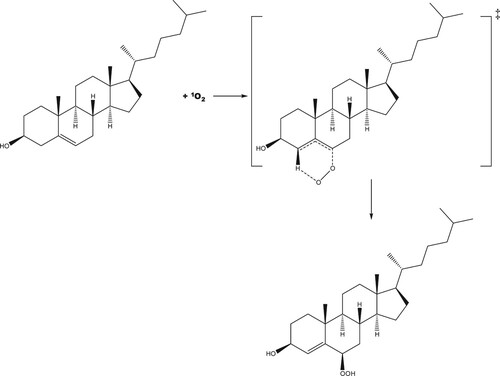
The reaction pathway for production of R-6-hydroperoxycholesterol has a concerted transition state in which the C–O and (O-)O-H bonds form simultaneously (see Figure ), a known facet of ‘ene’ reactions of singlet oxygen [Citation48]. The activation energy for this pathway is 2.0 kJ mol−1 lower in energy than that of the 5-hydroxyperoxycholesterol pathway, leading to a product that is 6.9 kJ mol−1 lower in energy than S-6-hydroxyperoxycholesterol.
3.2 General discussion
For each of the reactions of singlet oxygen with cholesterol considered, double-bond migration is observed, consistent with the ‘ene’ reaction. Production of R-6-hydroperoxycholesterol proceeds through a one-step, concerted mechanism, with oxygen addition and removal of a hydrogen atom from a neighbouring carbon (resulting in the double-bond migration), while production of 5-hydroperoxycholesterol and S-6-hydroperoxycholesterol proceeds via a two-step reaction, with the C–O bond forming first, followed by removal of the hydrogen atom from the C–H bond, leading to the double bond migration. These same results were also seen in the gas phase calculations (see Supporting Information). Both the 5-hydroperoxycholesterol and S-6-hydroperoxycholesterol pathways have an epoxide-like intermediate (Schemes 1 and 3); these could be the starting point for production of the significant (parts-per-million) levels of 5,6-epoxides found in in human blood [Citation12]. This intermediate may therefore react with NO2 in vivo to produce these epoxides [Citation49].
Figure 4. Reaction energy profile for the reaction of cholesterol and 1O2 to produce S-6-hydroperoxycholesterol (see Figure ). Molecular structures of transition states and intermediates are given in Scheme 3 and Figure . All values are relative to the previous step.
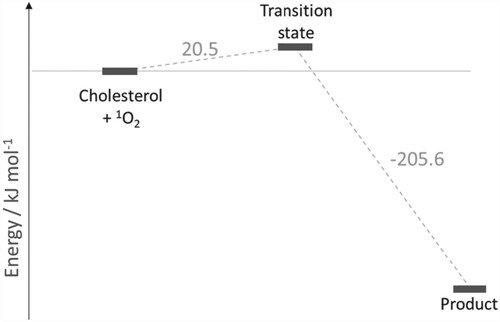
Figure 5. (a) Molecular structure of the first transition state for the addition of singlet oxygen to cholesterol at the 5 position; (b) Epoxide-like intermediate; (c) second transition state, leading to the formation of 5-hydroperoxycholesterol.
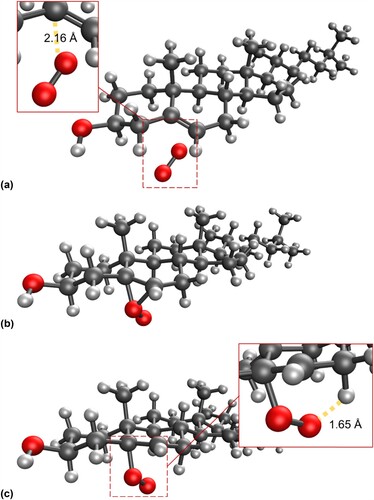
Figure 6. Molecular structure of the concerted transition state for the addition of singlet oxygen to cholesterol at the 6 position to form the R-6-hydroperoxycholesterol.
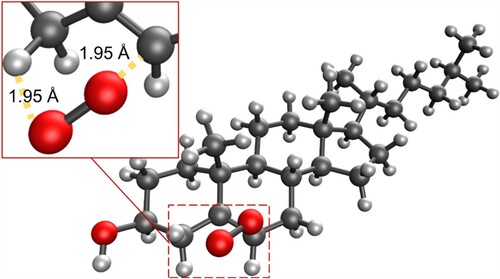
Figure 7. (a) Molecular structure of the first transition state for the addition of singlet oxygen to cholesterol at the 6 position; (b) Epoxide-like intermediate; (c) second transition state, leading to the formation of S-6-hydroperoxycholesterol.
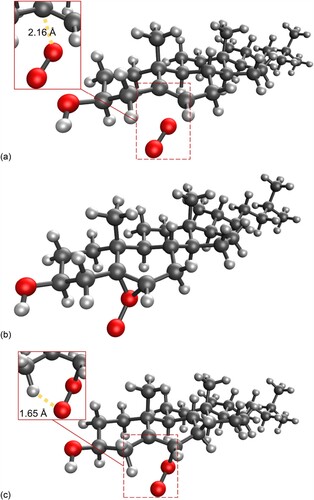
Scheme 1. Proposed reaction scheme for the production of 5-hydroperoxycholesterol via the ‘ene' reaction.
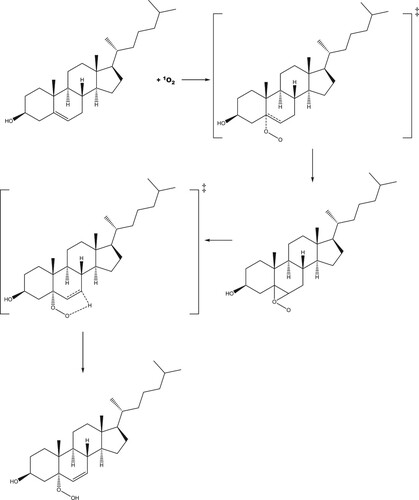
Table 1. Gibbs free energy values for the different reactions (kJ mol−1). All values are relative to the reactants.
The free energies of reaction are summarised in Table and rate constants calculated using the Eyring equation are given in Table . The reaction of singlet oxygen and cholesterol to produce 5-hydroperoxycholesterol is 2.1 times faster than the reaction resulting in production of S-6-hydroperoxycholesterol, in good agreement with experimental data, where the ratio of the rate is 2.4–7.0 times faster in favour of production of 5-hydroperoxycholesterol [Citation50]. In contrast to experiment, the computed results show a slightly lower barrier for production of R-6-hydroperoxycholesterol than the other two derivatives. However, in the gas phase (Table S1 in the Supporting Information), the reaction of singlet oxygen to produce 5-hydroperoxycholesterol is ∼12–14 times faster than the reaction resulting in either of the 6-hydroperoxycholesterol enantiomers, in qualitative agreement with experimental data. In the case of the rate-limiting transition states, the R-6-hydroperoxycholesterol pathway shows the largest stabilisation from gas phase to condensed phase, relative to the reactants. Given the nature of this cyclic transition state (see Scheme 2 and Figure ), this may be expected in solution phase for the continuum model employed. We conjecture that an explicit solvent scheme (e.g. including explicit solvent molecules in addition to the PCM model) may better capture the nature of these transition states; this would confirm if the solvent plays a direct role, in which e.g. a proton shuttle mechanism is in play, rather than just a bulk dielectric effect as demonstrated by the implicit continuum solvent model employed here.
Table 2. Calculated rate constants for the rate limiting steps for each of the products considered in this study. All calculated rate constants were calculated at a temperature of 298.15 K in the condensed phase.
4. Conclusions
We have investigated the reaction of singlet oxygen with cholesterol, an important component in vivo and also in food products. While the final products have long been known, we have elucidated the step-by-step mechanism by which the reactions take place. Reactions proceed via the ‘ene’ reaction for cholesterol, either as a two-step mechanism (5-hydroperoxycholesterol and S-6-hydroperoxycholesterol), or in the case of R-6-hydroperoxycholesterol, as a concerted one step mechanism. In the two step mechanisms, an epoxide-like intermediate is observed, which we speculate may react with NO2 in vivo to form 5 and 6-epoxide derivatives of cholesterol. This detailed level of understanding of the intermediates will aid further study of cholesterol oxidation and formation of products in vivo.
Dedication
I dedicate this work to the memory of Nick Besley, my former supervisor, colleague and friend.
Supplementary Material
Download MS Word (94.5 KB)Acknowledgements
We than NTU for provision of the Hamilton High Performance Computing cluster on which this work was performed.
Disclosure statement
No potential conflict of interest was reported by the author(s).
References
- W. Bäumler, J. Regensburger, A. Knak, A. Felgenträger and T. Maisch, Photochem. Photobiol. Sci. 11, 107–117 (2012).
- A. Knak, J. Regensburger, T. Maisch and W. Bäumler, Photochem. Photobiol. Sci. 13, 820–829 (2014).
- J. Lee and E.A. Decker, J. Agric. Food Chem. 59, 6271–6276 (2011).
- J.M. Lee, P.S. Chang and J.H. Lee, Food Chem. 119, 88–94 (2010).
- D.O. Ha, J. Yeo, S.T. Kang, M.J. Kim and J. Lee, Eur. J. Lipid Sci. Technol. 114, 780–786 (2012).
- J. Yeo and F. Shahidi, J. Agric. Food Chem. 69, 4204–4209 (2021).
- J.M. Dietschy and S.D. Turley, J. Lipid Res. 45, 1375–1397 (2004).
- Q. Zhang, E.T. Powers, J. Nieva, M.E. Huff, M.A. Dendle, J. Bieschke, C.G. Glabe, A. Eschenmoser, P. Wentworth, R.A. Lerner and J.W. Kelly, Proc. Natl. Acad. Sci. U. S. A. 101, 4752–4757 (2004).
- L.S. Dantas, A.B. Chaves-Filho, F.R. Coelho, T.C. Genaro-Mattos, K.A. Tallman, N.A. Porter, O. Augusto and S. Miyamoto, Redox Biol. 19, 105–115 (2018).
- A.B. Chaves-Filho, I.F.D. Pinto, L.S. Dantas, A.M. Xavier, A. Inague, R.L. Faria, M.H.G. Medeiros, I. Glezer, M.Y. Yoshinaga and S. Miyamoto, Sci. Rep. 9, 11642 (2019).
- D.A. Bosco, D.M. Fowler, Q. Zhang, J. Nieva, E.T. Powers, P. Wentworth, R.A. Lerner and J.W. Kelly, Nat. Chem. Biol. 2, 249–253 (2006).
- L.L. Smith, Free Radic. Biol. Med. 11, 47–61 (1991).
- X. Xu, R.P. Muller and W.A. Goddard, Proc. Natl. Acad. Sci. U. S. A. 99, 3376–3381 (2002).
- C.S. Foote, in Free Radicals Biol. (Elsevier, New York, 1976). pp. 85–133.
- P. Di Mascio, S. Kaiser and H. Sies, Arch. Biochem. Biophys. 274, 532–538 (1989).
- P.F. Conn, W. Schalch, T.G. Truscott and J. Photochem, Photobiol. B Biol. 11, 41–47 (1991).
- O. Hirayama, K. Nakamura, S. Hamada and Y. Kobayasi, Lipids. 29, 149–150 (1994).
- N. Shimidzu, M. Goto and W. Miki, Fish. Sci. 62, 134–137 (1996).
- A. Cantrell, D.J. McGarvey, T.G. Truscott, F. Rancan and F. Böhm, Arch. Biochem. Biophys. 412, 47–54 (2003).
- F. Ramel, S. Birtic, S. Cuiné, C. Triantaphylidès, J.L. Ravanat and M. Havaux, Plant Physiol. 158, 1267–1278 (2012).
- R. Edge and T.G. Truscott., Antioxidants. 7, 5 (2018).
- C.S. Foote and R.W. Denny, J. Am. Chem. Soc. 90, 6233–6235 (1968).
- A. Farmilo and F. Wilkinson, Photochem. Photobiol. 18, 447–450 (1973).
- D.C. Liebler, Ann. N. Y. Acad. Sci. 691, 20–31 (1993).
- J. Fiedor, L. Fiedor, R. Haeßner and H. Scheer, Biochim. Biophys. Acta – Bioenerg. 1709, 1–4 (2005).
- A. Nishino, H. Yasui and T. Maoka, J. Oleo Sci. 66, 77–84 (2017).
- J. Ferguson, Photodermatol. Photoimmunol. Photomed. 18, 262–269 (2002).
- B. Henry, C. Foti and K. Alsante, J. Photochem. Photobiol. B Biol. 96, 57–62 (2009).
- D.E. Moore, Drug Saf. 25, 345–372 (2002).
- E. Selvaag, J. Toxicol. Cutan. Ocul. Toxicol. 16, 77–84 (1997).
- C. Valencia, E. Tobón, and C. Castaño, J. Photochem. Photobiol. A Chem. 251, 113–117 (2013).
- M.J. Sabat, A.M. Wiśniewska-Becker, M. Markiewicz, K.M. Marzec, J. Dybas, J. Furso, P. Pabisz, M. Duda and A.M. Pawlak, Membranes (Basel). 11, 327 (2021).
- S. Miyamoto, R.S. Lima, A. Inague and L.G. Viviani, Free Radic. Res. 1, 1–25 (2021).
- J.M. King and D.B. Min, J. Food Sci. 63, 31–34 (1998).
- J. Da Chai and M. Head-Gordon, Phys. Chem. Chem. Phys. 10, 6615–6620 (2008).
- R. Krishnan, J.S. Binkley, R. Seeger and J.A. Pople, J. Chem. Phys. 72, 650–654 (1980).
- T.L. Li, J.M. King and D.B. Min, J. Food Biochem. 24, 477–492 (2000).
- N. Mardirossian and M. Head-Gordon, Mol. Phys. 115, 2315–2372 (2017).
- V. Barone and M. Cossi, J. Phys. Chem. A. 102, 1995–2001 (1998).
- T.N. Truong and E.V. Stefanovich, Chem. Phys. Lett. 240, 253–260 (1995).
- X. Zou, H. Zhao, Y. Yu and H. Su, J. Am. Chem. Soc. 135, 4509–4515 (2013).
- B.H. Munk, C.J. Burrows and H.B. Schlegel, J. Am. Chem. Soc. 130, 5245–5256 (2008).
- F. Liu, W. Lu, X. Yin and J. Liu, J. Am. Soc. Mass Spectrom. 27, 59–72 (2016).
- K. Fukui, J. Phys. Chem. 74, 4161–4163 (1970).
- K. Ishida, K. Morokuma and A. Komornicki, J. Chem. Phys. 66, 2153–2156 (1976).
- M.W. Schmidt, M.S. Gordon and M. Dupuis, J. Am. Chem. Soc. 107, 2585–2589 (1985).
- Y. Shao, Z. Gan, E. Epifanovsky, A.T.B. Gilbert, M. Wormit, J. Kussmann, A.W. Lange, A. Behn, J. Deng, X. Feng, D. Ghosh, M. Goldey, P.R. Horn, L.D. Jacobson, I. Kaliman, R.Z. Khaliullin, T. Kuś, A. Landau, J. Liu, E.I. Proynov, Y.M. Rhee, R.M. Richard, M.A. Rohrdanz, R.P. Steele, E.J. Sundstrom, H.L. Woodcock, P.M. Zimmerman, D. Zuev, B. Albrecht, E. Alguire, B. Austin, G.J.O. Beran, Y.A. Bernard, E. Berquist, K. Brandhorst, K.B. Bravaya, S.T. Brown, D. Casanova, C.-M. Chang, Y. Chen, S.H. Chien, K.D. Closser, D.L. Crittenden, M. Diedenhofen, R.A. DiStasio, H. Do, A.D. Dutoi, R.G. Edgar, S. Fatehi, L. Fusti-Molnar, A. Ghysels, A. Golubeva-Zadorozhnaya, J. Gomes, M.W.D. Hanson-Heine, P.H.P. Harbach, A.W. Hauser, E.G. Hohenstein, Z.C. Holden, T.-C. Jagau, H. Ji, B. Kaduk, K. Khistyaev, J. Kim, J. Kim, R.A. King, P. Klunzinger, D. Kosenkov, T. Kowalczyk, C.M. Krauter, K.U. Lao, A.D. Laurent, K.V. Lawler, S.V. Levchenko, C.Y. Lin, F. Liu, E. Livshits, R.C. Lochan, A. Luenser, P. Manohar, S.F. Manzer, S.-P. Mao, N. Mardirossian, A.V. Marenich, S.A. Maurer, N.J. Mayhall, E. Neuscamman, C.M. Oana, R. Olivares-Amaya, D.P. O’Neill, J.A. Parkhill, T.M. Perrine, R. Peverati, A. Prociuk, D.R. Rehn, E. Rosta, N.J. Russ, S.M. Sharada, S. Sharma, D.W. Small, and A. Sodt, Mol. Phys. 113, 184–215 (2015).
- H.H. Wasserman and J.L. Ives, Tetrahedron. 37, 1825–1852 (1981).
- A. Sevanian, N. Elsayed and A.D. Hacker, J. Toxicol. Environ. Health. 10, 743–756 (1982).
- W. Korytowski, G.J. Bachowski and A.W. Girotti, Photochem. Photobiol. 56, 1–8 (1992).