ABSTRACT
Microcystin, a common class of cyanobacterial toxin, was first detected in New Zealand in 1997. Subsequent research has demonstrated that microcystins are widely distributed around New Zealand and are commonly detected in planktonic samples from lakes and reservoirs. Microcystis is the predominant microcystin-producing cyanobacteria reported in New Zealand and two different microcystin congener profiles have been detected in the majority of samples tested. Benthic species of Planktothrix and Nostoc have also been confirmed as microcystin producers. Whilst the Planktothrix is infrequently observed, Nostoc is common in lakes, rivers and terrestrial environments. However, there is relatively little information on microcystin congener diversity or the distribution of microcystin-producing Nostoc in New Zealand. To-date, genome sequencing and analysis has only been conducted on one New Zealand cyanobacterium (Planktothrix CAWBG59). Further genome sequencing of New Zealand microcystin-producing cyanobacteria could provide valuable insights into the genetic controls for microcystin production. There was a high level of inter-strain variability in microcystin cell quotas from Microcystis, highlighting the need for regional analyses to be conducted for risk assessments. Research on the ecological impact of microcystins is limited but shows the toxin can accumulate in New Zealand aquatic organisms, and, when microcystin concentrations are sufficiently high, have negative effects on survival and behaviour.
Introduction
Cyanobacteria are photosynthetic prokaryotic organisms that are an integral part of many terrestrial and aquatic ecosystems. Under favourable conditions, some cyanobacteria multiply rapidly and form blooms in lakes and rivers. An increasing number of cyanobacteria are known to have toxin-producing strains (Buratti et al. Citation2017). These natural toxins, known as cyanotoxins, are a threat to humans and animals when consumed in drinking water or from contact during recreational activities. The most commonly reported cyanotoxins are the microcystins (MCs; Harke et al. Citation2016). They were first isolated as a crude extract from Microcystis aeruginosa strain NRC-1 by Bishop et al. (Citation1959) and have subsequently been isolated from a variety of other cyanobacteria (Chorus and Bartram Citation1999; Buratti et al. Citation2017).
Microcystins (and the structurally similar nodularins) contain the unique amino acid Adda (3-amino-9-methoxy-2,6,8-trimethyl-10-phenyldeca-4,6-dienoic acid) in Position 5, d-amino acids in Positions 1 and 3 and frequently contain Mdha (N-methyldehydroalanine) in Position 7 (). Because an assortment of amino acids can be incorporated into Positions 2 and 4 and a range of structural modifications are observed at all positions in the structure, greater than 250 structural congeners have been reported to-date (Meriluoto and Spoof Citation2008).
Microcystins are commonly measured in water samples and cyanobacteria extracts using liquid chromatography-mass spectrometry (LC-MS) and Adda-specific enzyme-linked immunosorbent assay (ELISA). LC-MS is a highly-specific analytical technique where complex mixtures of compounds are separated by LC (generally reversed-phase C18) and analysed according to their mass-to-charge ratio (m/z) by MS. Modern LC-MS methods for microcystins will also use tandem-MS (MS/MS) and multiple-reaction monitoring to further increase specificity by fragmenting the precursor ion and measure the intensity of fragment ions diagnostic for microcystins (e.g. the m/z 135 fragment ion from Adda; Hummert et al. Citation2001; Diehnelt et al. Citation2006). Because these methods are highly specific, unusual microcystin congeners with different masses or different fragmentation patterns may not be measured. Furthermore, because the LC-MS response can vary between different microcystin congeners, accurate quantification relies on calibration standards being available for the microcystin congeners being analysed. Semi-quantitation is possible using structurally-similar microcystins for calibration but is prone to errors.
Adda-ELISA uses antibodies raised against Adda, the unique amino acid found in microcystins and nodularins. The technique it is not able to distinguish between microcystin and nodularin or between the different microcystin congeners. The non-specific nature of the method makes it very amenable to determining the ‘total’ concentration of microcystins in a sample and for detecting the presence of previously unidentified microcystin congeners. Because of differences in cross-reactivities for the different microcystin congeners, the accuracy of Adda-ELISA measurements may be affected by the microcystin profile in the sample. Adda-ELISA can also be susceptible to matrix effects yielding ‘false positives’ when dense cyanobacteria extracts are analysed.
Microcystins are hepatotoxins which primarily accumulate in the livers of animals (or the hepatopancreas of crustaceans) and block protein phosphatases 1 and 2a (MacKintosh et al. Citation1990). Most microcystins are highly toxic, with intraperitoneal mouse toxicities ranging between 50–300 µg/kg body weight and only a few non-toxic variants have been identified (Carmichael Citation2001). Numerous incidents of animal and human poisonings have been attributed to microcystins (Ressom et al. Citation1994). One of the most severe cases occurred in Caruaru, Brazil, in 1996 when processes at a water treatment plant failed and manual addition of chlorine to tanker loads of water supplying a dialysis clinic was insufficient to remove the microcystins. This resulted in 56 fatalities (Azevedo et al. Citation2002). In a further case from Brazil, 88 people died after drinking water that came from a newly constructed reservoir where a Microcystis bloom occurred (Teixeira et al. Citation1993). Microcystins are also considered to be chronic carcinogens and can cause long-term liver damage (Falconer et al. Citation1983). Epidemiological evidence from China (Ueno et al. Citation1996; Li et al. Citation2011), Serbia (Svirčev et al. Citation2009, Citation2013) and USA (Zhang et al. Citation2015) has linked the continual consumption of low doses of microcystins in drinking water to an increased incidence of primary liver cancer. Numerous studies have also shown that microcystins can accumulate in a variety of freshwater and marine organisms (Pham and Utsumi Citation2018) and have impacts on reproduction, development and behaviour (Ferrão-Filho and Kozlowsky-Suzuki Citation2011).
The aim of this review is to collate the published data available on the occurrence of microcystins and microcystin research undertaken in New Zealand. In particular, we focus on detailing the diversity of microcystin congeners observed in New Zealand cyanobacteria, collating microcystin cell quotas (the amount of toxin produced per cell) and highlighting current knowledge gaps and future research needs that will assist in refining national risk assessments and increasing understanding on toxic cyanobacteria in New Zealand.
The history of microcystin research in New Zealand
Low levels of microcystins were first detected in New Zealand in a sample collected from a cyanobacterial bloom in Lake Rotorua (North Island) in 1997 (Wilding Citation2000). The toxicity of this sample was confirmed using a mouse bioassay and microcystin content was quantified using a protein phosphatase inhibition assay (PPIA). The low levels of microcystins in the sample did not adequately explain the positive mouse bioassay result suggesting that there could have been other toxins present, although Wilding (Citation2000) also noted that this could have been due to sample degradation decreasing the levels of microcystin present in the samples. The dominant cyanobacteria in these samples were Dolichospermum (previously Anabaena) circinalie and Dolichospermum flos-aquae. All other samples analysed for toxicity from the Rotorua lakes between 12 December 1996 and 17 May 1999 showed no toxicity.
Christoffersen and Burns (Citation2001) used an Adda-ELISA assay to analyse samples from seven South Island lakes for the presence of microcystins. The ELISA used in their study did not distinguish between microcystins and nodularins, thus it is possible that some of the detections could have been due to nodularin (e.g. Lake Ellesmere/Waihora and Lake Forsyth/Wairewa). The levels of microcystins/nodularins that were reported in the seven lakes were low; Lake Johnson (Otago; 0.0003–0.0013 µg/L), Butchers Dam (Otago; 0.0021 µg/L), Lake Rotorua (Kaikoura; 1.2 µg/L), Lake Ellesmere/Te Waihora (Canterbury; 5.8 µg/L), Lake Forsyth/Te Wairewa (Canterbury; 0.0052 µg/L) and Lake Roundabout (Canterbury; 0.001 µg/L). The dominant cyanobacterial genera in these bloom samples were Dolichospermum and Microcystis. Hamill (Citation2001) investigated a series of dog deaths at the Mataura River in 1998/1999, and while anatoxins were most likely responsible for these mortalities, microcystins/nodularins were also detected in benthic Oscillatoria mats using ELISA.
The first LC-MS/MS analysis of New Zealand samples for microcystins was undertaken by Stirling and Quilliam (Citation2001). The authors detected several different microcystin congeners in a cyanobacterial bloom sample from Lake Waitawa (Kapiti coast); MC-LR (8.1 µg/L), MC-RR (5.6 µg/L), MC-FR, MC-WR and MC-LA. Mountfort et al. (Citation2005) investigated the differences in microcystin levels obtained using Adda-ELISA, PPIA and LC-MS/MS in samples from Lake Horowhenua (Levin) and the outlet of this lake, Hokio Stream. The maximum levels recorded at these sites (using the Adda-ELISA) were 260 and 130 µg/L respectively (rounded to two significant figures). As the LC-MS/MS method used in this study only measured three microcystin congeners and nodularin-R, the LC-MS/MS results were consistently lower than those achieved using ELISA and PPIA.
The first large-scale survey of New Zealand waterbodies for microcystins was undertaken by Wood et al. (Citation2006b). They analysed 266 water and cyanobacterial mat samples collected from 227 different water bodies between 2001–2004. Using Adda-ELISA, microcystins/nodularins were detected in 102 samples from 54 different water bodies (27 planktonic lake samples, 1 benthic mat sample from a lake, 12 planktonic samples from ponds or dams, 7 planktonic samples from oxidation ponds, 5 benthic mats samples from streams). The highest microcystin concentration was detected in Lake Horowhenua (Levin; 36,500 µg/L), followed closely by Lake Waitawa (Kapiti coast; 32,000 µg/L). Whilst microcystin concentrations this high have not been reported in New Zealand since, 13,000 µg/L of microcystins was detected in Lake Horowhenua cyanobacteria using cryogenic samplers (Puddick et al. Citation2016b). Five of the samples from Wood et al. (Citation2006b; Lake Horowhenua, Lake Ngaroto, Lake Waitawa, Nuemans Pond and a benthic mat sample from Lake Taupo) were analysed by LC-MS/MS to explore the microcystin congener diversity. The Lake Taupo Nostoc mat sample had a simple microcystin congener profile with four congeners detected (MC-RR, dmMC-RR, MC-LR and dmMC-LR), whilst the other four samples contained a diverse range of microcsytin congeners (MC-RR, MC-YR, MC-LR, MC-FR, MC-WR, MC-RA, MC-LA, MC-LY).
A dog death at the Waitaki River in November 2008 led to an investigation of the toxin content of a benthic ‘algal’ mat the dog had been observed eating. Cyanobacteria strains were isolated from the sample and molecular analysis indicated it was Planktothrix (Wood et al. Citation2010). Analysis by LC-MS identified a number of microcystin congeners including several that had not been previously identified in New Zealand. The genome for one of the strains isolated by Wood et al. (Citation2010) was sequenced as part of a global study on the diversity and lifeforms of this genus (Pancrace et al. Citation2017). This study led to a number of novel findings such as the presence of genes involved in gas vesicle production in all benthic strains studied. It also showed that the biosynthetic gene clusters dedicated to natural product production in the New Zealand benthic strain was more similar to the planktonic strains than other benthic strains.
The identification of the genes responsible for microcystin production (Nishizawa et al. Citation2000; Tillett et al. Citation2000) started a new era of studies using assays based on polymerase chain reaction (PCR) to screen for the presence of microcystin production genes. The first published study to incorporate these techniques in New Zealand was Weller (Citation2011) who analysed 18 samples collected over a 6-week period from Lake Rotorua, Lake Rotoiti and Lake Rotoehu (Rotorua region). The mcyE gene specific to Microcystis and Dolichospermum was detected, although no culturing studies were conducted to confirm the toxin-producing strains. Using matrix-assisted laser desorption/ionisation time-of-flight MS, MC-LR was detected in the samples.
Wood et al. (Citation2017b) undertook a survey of cyanobacteria in 143 New Zealand lakes in 2012/2013 using a PCR-based approach to screen samples. The mcyE gene was detected in 66 samples and microcystins were confirmed in 21 samples using LC-MS/MS. The highest microcystin concentration detected was from Lake Horowhenua (Levin; 55 µg/L) which was substantially lower than the microcystin levels detected at the same lake during the 2001–2004 survey (Wood et al. Citation2006b). Gene sequencing indicated that the toxin-producing organisms were likely to be Dolichospermum lemmermannii and Microcystis. Culturing of these cyanobacteria confirmed microcystin production by Microcystis, but no microcystin was detected in D. lemmermannii despite culturing and testing numerous strains.
The most common means of ingesting microcystins is through drinking contaminated water or eating aquatic organisms where toxins have accumulated. A lesser-researched exposure route is inhalation. Wood and Dietrich (Citation2011) investigated microcystin levels in aerosols using high- and low-volume air samplers for 4, 12 and 24 h periods at Lake Rotorua (Kaikoura) during a Microcystis bloom. Using the high-volume samplers, up to 1.8 pg of microcystin/m3 of air was measured. Based on average human air intakes and assuming inhalation toxicities are comparable to the toxicological data obtained following intraperitoneal applications in mice, the authors indicated that the aerosolised microcystins measured did not represent an acute or chronic hazard to humans.
In recent years, research has moved away from purely characterising the microcystins present in New Zealand and the cyanobacteria that produce them and has begun advancing international knowledge on the factors that regulate microcystin production. Using field-based studies at Lake Rotorua (Kaikoura), Wood et al. (Citation2011) showed for the first time that Microcystis can ‘switch’ toxin-production on and off. They detected ca. 20-fold changes in microcystin quotas and measured greater than 400-fold changes in mcyE gene expression. In 2012, the same research team used mesocosms to experimentally induce similar upregulation in microcystin quotas (Wood et al. Citation2012). In both studies, these dramatic changes were associated with increased Microcystis densities and were not caused by a shift in the relative abundance of toxic/non-toxic genotypes. A study by Puddick et al. (Citation2016b) conducted a similar mesocosm experiment but sampled the Microcystis scums that developed on the surface using cryogenic samplers which produced fine-scale profiles of the top 40 mm of the water column. This demonstrated that the observed upregulation in microcystin quotas was highly localised at the very top of the water column near the air–water interface.
Because of the congener-specific toxicity differences observed with microcystins (Buratti et al. Citation2017), knowledge on which environmental conditions promote the production of different microcystin congeners is important. A laboratory culturing study using Microcystis CAWBG11 showed that nitrogen depletion led to a reduction in arginine-containing microcystin congeners (Puddick et al. Citation2016a). As arginine is a nitrogen-rich amino acid, this shift in microcystin congener abundance is most likely a mechanism for conserving nitrogen in times of shortage. The study also highlighted the difficulty of investigating the effects of low nutrient concentrations on microcystin production because cyanobacteria are very effective at storing reserves of nitrogen and phosphate.
A study conducted at Lake Waitawa (Kapiti Coast) assessed cyanobacterial scums (comprised of Microcystis and Dolichospermum) as they decomposed, but did not find strong links between microcystin content and the other parameters assessed (Steiner et al. Citation2017). The authors also used 16S rRNA metabarcoding to explore the bacterial community associated with the cyanobacterial samples. Proteobacteria was the most abundant phylum, among which Aeromonas, Caulobacter and Brevundimonas dominated. No relationships were observed between microcystin quotas and the bacterial communities. Bacteria are known to degrade microcystins, and Somdee et al. (Citation2013) isolated microcystin-degrading bacteria from Lake Rotoiti (Rotorua region). Its biodegradation efficiency was tested using [Dha7] MC-LR and MC-LR purified from Lake Horowhenua (Levin) bloom material.
Wood et al. (Citation2017a) collected weekly or fortnightly samples from Lake Rotorua Kaikoura between January 2014 and May 2015 to better understand how microcystin concentrations changed in the lake. Using quantitative-PCR (qPCR; targeting the mcyE gene) and LC-MS/MS, the authors explored how the relative abundance of toxic and non-toxic Microcystis genotypes, and toxin quotas varied between two contrasting summers (dry and wet) and the possible drivers of these differences. Microcystis genotype composition was highly variable, particularly in the wet summer and no environmental parameters measured were correlated with the observed shifts in toxin-producing genotypes. However, a positive relationship (p < 0.001) was identified between microcystin quotas and surface water temperature, although the authors cautioned against over interpreting the result.
Confirmed microcystin producers in New Zealand
Our criteria for a cyanobacterium to be a confirmed microcystin producer is that it must be isolated, cultured and microcystin production determined using LC-MS. To-date, only three cyanobacteria genera have been confirmed as microcystin producers in New Zealand; Microcystis sp. (e.g. Puddick et al. Citation2014), Planktothrix sp. (South Island; Wood et al. Citation2010), and Nostoc sp. (isolated from a benthic mat collected from the Hakataramea River in Canterbury; Wood and Puddick unpublished data).
Microcystin congeners identified in New Zealand
A range of microcystin congeners have been detected in New Zealand freshwater environments; from relatively common congeners (e.g. MC-LR, MC-RR, MC-YR) to more unusual structural variants (e.g. [Asp3, ADMAdda5] MC-LHar, [Dha7] MC-HilR, MC-(H4)YR; ). The most frequently encountered microcystin congener profiles in New Zealand cyanobacteria appear to be from a Microcystis species which produces predominantly [Dha7] MC-LR, with lower levels of [Asp3, Dha7] MC-LR and MC-LR (see CAWBG617 in ; Rogers Citation2014), and a Microcystis species that produces a wide array of toxin congeners (> 27 congeners including MC-RR, MC-YR, MC-LR, MC-FR, MC-WR, MC-RA, MC-RAba, MC-LA, MC-FA, MC-WA, MC-LAba, MC-FAba, MC-WAba, and their desmethylated variants; see CAWBG11 in ; Puddick et al. Citation2014). The isolation of cyanobacterial strains and observations in the field suggest that Microcystis strains similar to CAWBG617 exist as single-cells or small colonies under isolation in the laboratory, but in the natural environment can form large colonies that easily disaggregate into smaller colonies. Strains similar to CAWBG11 form large intact colonies in the natural environment and remain as large colonies upon isolation in the laboratory (cultures isolated in 2005 still maintain the same morphology).
Table 1. Microcystin congeners reported in studies on New Zealand environmental samples and cyanobacteria strains.
Once the wide array of microcystin congeners produced by Microcystis CAWBG11 was established in 2013, it was identified that similar profiles of microcystin congeners had been previously observed in New Zealand (Wood et al. Citation2006a, Citation2006b; Crush et al. Citation2008; Puddick and Prinsep Citation2008; ). In the earlier studies, many of the hydrophobic microcystin congeners (e.g. MC-FA, MC-WA, MC-FAba, MC-WAba) produced by Microcystis strains similar to CAWBG11 were not measured as they had not yet been identified. Other microcystin congeners (e.g. MC-LAba and MC-RAba) had been identified overseas but were not thought to be prevalent in New Zealand cyanobacteria. These observations reinforce the need for exploratory research on the toxin congeners present in different waterbodies and the assessment of data compiled from different regions. This would enable LC-MS/MS methods to be tailored for the microcystin congeners most likely to be observed in a specific region and reduce the frequency of microcystin congeners being ‘missed’ during analysis.
An additional consideration when analysing microcystins by LC-MS is that oxidation artefacts can occur in tryptophan- and methionine-containing microcystins (Puddick et al. Citation2013a; Miles et al. Citation2014). Because oxidation of these residues alters the mass of the microcystin, they will not be detected by LC-MS unless the method is specifically-tailored for their analysis. Whilst tryptophan-containing microcystins have been observed in New Zealand (as well as their oxidation artefacts; Puddick and Prinsep Citation2008; Puddick et al. Citation2013a), methionine-containing microcystins have not been reported to-date. The relative proportion of the oxidised congener in relation to the parent congener was not assessed in the natural environment and no toxicity work on the oxidised microcystin congeners has been undertaken.
The majority of information on microcystins in New Zealand is based on Microcystis, with several exceptions; a Planktothrix species that produces [Asp3] MC-LR, [Asp3, Dha7] MC-LR, MC-LR, [Asp3, ADMAdda5] MC-LHar (Wood et al. Citation2010) and Nostoc species that produce dmMC-RR, MC-RR, dmMC-LR and MC-LR (Wood et al. Citation2006b; Wood and Puddick unpublished data). Whilst the Planktothrix species isolated in Wood et al. (Citation2010) is not commonly observed, Nostoc is frequently found in terrestrial, river and lake environments around New Zealand. Despite its frequent presence, there is very little information on microcystins in New Zealand Nostoc. International studies on microcystins from Nostoc have shown that they commonly contain structural modifications not frequently detected in New Zealand; e.g. variants containing acetyl-desmethyl-Adda (ADMAdda), dehydrobutyrine (Dhb) and homoarginine (Har; Beattie et al. Citation1998; Fewer et al. Citation2007; Puddick et al. Citation2015). Further investigation of microcystin congeners produced by New Zealand Nostoc species using exploratory techniques such as those described in Kleinteich et al. (Citation2018), as opposed to highly-specific LC-MS/MS detection techniques, may identify a wider array of microcystin congeners in New Zealand.
Microcystin cell quota values in New Zealand Microcystis
Planktonic cyanobacterial monitoring in New Zealand waterbodies used for recreational activities generally follow the protocols and thresholds set out in the ‘Interim New Zealand Guidelines for Cyanobacteria in Recreational Fresh Waters’ (Ministry for the Environment and Ministry of Health Citation2009). These biovolume thresholds were established using toxicity calculations based on the negative health effects of microcystins, the likelihood of exposure to the toxins, and the expected microcystin cell quota. In this review we collated data from the 2009 interim cyanobacteria guidelines and six new studies ().
Table 2. Collated microcystin cell quota values from studies on New Zealand Microcystis.
The compiled studies use two different methods to quantify microcystins which provide slightly different information. The ELISA used in two of the compiled studies uses antibodies raised against Adda (an amino acid unique to microcystins/nodularin) and should detect the majority of known microcystin congeners and nodularin (Fischer et al. Citation2001). ‘Free’ Adda may also be detected in some instances, potentially overestimating total microcystin load in a sample (e.g. Wood et al. Citation2008a). In contrast, LC-MS/MS detects the specific mass of individual toxins in a sample and thus provides information on which congeners are present. This is particularly relevant for microcystins, where over 250 congeners exist (Meriluoto et al. Citation2016) and structural differences can affect their toxicity. The LC-MS/MS methods used in the collated studies detected the 18 most commonly observed microcystin congeners found in New Zealand Microcystis (see previous section), and the sum of the concentration of the individual microcystin congeners was used.
The studies also use two different methods for quantifying the concentration of Microcystis cells present in the samples; microscopy enumeration or a qPCR assay targeting the mcyE gene (a gene involved in the production of microcystins). Because microscopy cannot distinguish between toxic and non-toxic cells, environmental studies utilising this methodology may over-estimate the concentration of toxin-producing cells, leading to an under-estimation of the microcystin cell quota.
A total of 187 microcystin quota values were collated (). The highest microcystin quota detected among the seven studies was 13 pg/cell in Puddick et al. (Citation2016b; ). The median microcystin quota in this study was also higher than all other studies (6.0 pg/cell). A limitation with the sampling technique employed in this study was that the extracellular microcystins could not be distinguished from the intracellular microcystins. An ANOVA with Tukey’s post-hoc test which showed the microcystin quotas reported in the Puddick et al. (Citation2016b) study were significantly higher than those of all of the other studies (p < 0.05; ). For these reasons, this dataset was excluded from further assessment.
Figure 2. Microcystin cell quotas from studies on New Zealand Microcystis sp., sourced from four different lakes (denoted by colour). All studies used environmental samples except for those denoted with *, which used cultured stains of Microcystis. Data are displayed on: (A) a linear, and (B) a logarithmic y-axis. Identical letters above the box indicate no statistically significant difference between studies (p > 0.05; One-way ANOVA, Tukey HSD).
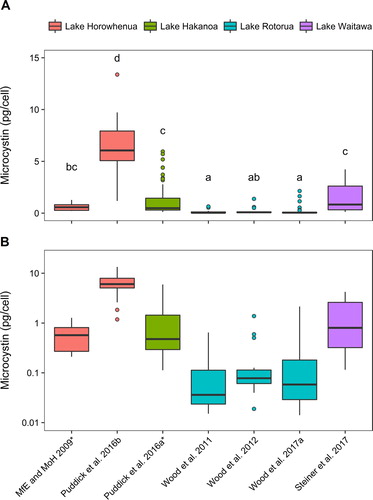
When the data were assessed, excluding the Puddick et al. (Citation2016b) study, the median microcystin cell quota from the combined datasets was 0.28 pg/cell, the lowest quota value was 0.006 pg/cell (Wood et al. Citation2017a), and the highest was 5.9 pg/cell (Puddick et al. Citation2016a; ). The median values from the individual studies ranged from 0.036 pg/cell (Wood et al. Citation2011) to 0.86 pg/cell (Steiner et al. Citation2017). There were statistically significant differences between the studies (ANOVA F5,149 = 27.09, p < 0.001) which could be due to the different methodologies used or differences in the Microcystis strains present in the different lakes. A number of international studies have shown differences in microcystin production among strains of the same species (e.g. Vezie et al. Citation1998; Saker et al. Citation2005) and this was noted for Microcystis strains CAWBG13–17 isolated from Lake Horowhenua (Levin) where a 6-fold difference in microcystin cell quota was reported (Ministry for the Environment and Ministry of Health Citation2009). Within each study set assessed there were also marked differences in microcystin cell quotas (6- to 350-times difference between the minimum and the maximum values). Because of the variability in microcystin cell quotas observed, risk assessments should take a conservative approach and use the highest reported microcystin quota from the region in order to protect human health.
When the microcystin cell quota values from New Zealand were compared with data reported from Microcystis in other countries, New Zealand microcystin quotas were generally higher (). For example, the lowest median value from the New Zealand studies (0.036 pg/cell; Wood et al. Citation2011) was higher than the microcystin cell quotas in five of the seven studies assessed. Two of the studies Sabart et al. (Citation2013) and Yu et al. (Citation2014) reported microcystin quotas closer to the New Zealand data (0.03–4 pg/cell and 0.012–1.9 pg/cell respectively). As several of the New Zealand studies assessed dense ‘scums’ of Microcystis, which has been shown to stimulate microcystin production in Microcystis (Wood et al. Citation2011, Citation2012), this may have led to higher microcystin quotas.
Table 3. Microcystin (MC) cell quota values from international studies on Microcystis.
Studies investigating accumulation or effects of microcystins on aquatic organisms in New Zealand
To the best of our knowledge, the only research undertaken on the effect of microcystins on New Zealand zooplankton was undertaken by Christoffersen and Burns (Citation2001). Using an acute toxicity test with the freshwater daphnid Daphnia carinata, they showed that algal material from Lake Rotorua, Lake Forsyth/Wairewa and Lake Ellesmere/Waihora caused higher mortality than would have been expected with just microcystins/nodularins, thus they concluded the algal material from these lakes must contain toxic substances which were not detected by the Adda-ELISA used.
Crush et al. (Citation2008) investigated the uptake and accumulation of microcystins in lettuce (Lactuca sativa), rape (Brassica napa), perennial ryegrass (Lolium perenne) and white clover (Trifolium repens) by irrigating with microcystin-contaminated lake water. The authors observed differences in microcystin uptake between the different plant species and that higher levels accumulated in the roots of all plants, rather than the shoots. Microcystin did not translocate from roots to shoots in these plants, suggesting that aerial spray irrigation poses a greater human health risk than drip irrigation at ground level. When irrigated with water containing 2,100 µg/L of microcystins, toxin accumulation was observed in all plant species (max. 0.24 µg/kg in ryegrass, max. 1.9 µg/kg in clover, max. 0.18 µg/kg in rape and max. 0.87 µg/kg in lettuce). The authors noted that the levels observed in lettuce would exceed the World Health Organisation tolerable daily intake of 0.04 µg of microcystins/kg of body weight/day for a 60 kg person consuming a typical salad.
Wood et al. (Citation2006a) investigated microcystin accumulation in rainbow trout (Oncorhynchus mykiss) and freshwater mussels (kākahi; Echyridella menziesi) in Lake Rotoiti and Lake Rotoehu (Rotorua region). Hatchery rainbow trout were added to an enclosure in Lake Rotoiti where microcystin concentrations were monitored. Rainbow trout that were free to roam in the entire area of each lake were also included in the study. Kākahi were suspended sub-surface in cages in the enclosure. Adda-ELISA results confirmed the presence of microcystins in rainbow trout liver (max. 79 µg/kg; free roaming, Lake Rotoehu), muscle tissue (max. 35 µg/kg free roaming, Lake Rotoehu) and in kākahi (max. 65 µg/kg). Microcystins were detected in the rainbow trout tissue/liver even when there was no detectable toxin in the water column and there had not been blooms for several months, suggesting accumulation of the toxin through the food web. The microcystin congeners MC-LR, MC-YR, MC-RR, MC-RA (recorded as MC-AR in the publication), MC-FR, MC-LA and MC-WR were detected in caged kākahi by LC-MS/MS, but not in either the rainbow trout muscle or liver tissue. Modelling was carried out to estimate the potential for adverse health risks following consumption of rainbow trout muscle tissue. The authors suggested it was unlikely that eating rainbow trout from these lakes as part of a regular diet (several meals per week) would result in any adverse health effects, assuming the Adda-ELISA was determining compounds of equivalent toxicity to MC-LR.
The effect of microcystins on two native freshwater species was studied by Clearwater et al. (Citation2014). The authors showed that the survival of juvenile kākahi and New Zealand freshwater crayfish (kōura; Paranephrops planifrons) decreased after four days exposure to microcystin-containing cell-free extracts that were at concentrations typically detected in cyanobacterial blooms. Whilst kōura survival was 100% when fed freeze-dried microcystin-containing Microcystis sp., growth rates were significantly lower compared with controls, but not compared with a diet incorporating non-toxic cyanobacteria. Shade- and shelter-seeking behaviour of kōura was also negatively impacted.
International research has highlighted the potential for microcystin from freshwater sources to accumulate in marine species (e.g. Miller et al. Citation2010; Gibble et al. Citation2016). In New Zealand, Lake Omapere (Northland) contributes significant inputs of freshwater to Hokianga Harbour and at times has severe toxin-containing cyanobacterial blooms. During the summer of 2004, ELISA analysis indicated microcystins were present in Pacific oysters (Crassostrea gigas) in Hokianga Harbour, leading to farm closures and health warning advisories against recreational harvesting. However, LC-MS/MS analysis could not detect microcystins (Holland et al. Citation2005). An in vitro feeding trial of C. gigas with microcystin-producing Microcystis was undertaken, but no microcystins were detected in the shellfish using LC-MS/MS (Wall et al. Citation2013).
Future research on microcystins in New Zealand
Research over the past two decades has primarily focused on the detection of microcystins in New Zealand and identifying the toxin-producing cyanobacteria. Although to-date only three microcystin-producing genera of cyanobacteria have been confirmed, we predict that others will be identified in the future. Further research is needed to investigate microcystin producers in benthic mats. Hamill (Citation2001) and Wood et al. (Citation2006b) both reported the presence of microcystins in benthic mat samples. Whilst systematic surveys have been undertaken on planktonic samples, the same approach has not been broadly applied to benthic mats and in many instances only anatoxins are tested for given their high prevalence (McAllister et al. Citation2016). Further exploration of less well characterised environments; e.g. thermal and alpine habitats may also yield new producers. New planktonic cyanobacteria continue to be reported, either as a result of introductions or environmental change (Rueckert et al. Citation2007; Wood et al. Citation2014), thus vigilance is required, and on-going microcystin testing is suggested even in the absence of known producers.
Among the currently known producers there is a clear gap in knowledge on the microcystin congeners produced by Nostoc. Phylogenetic studies and more information on the species distribution and microcystin congener diversity are needed. To-date Planktothrix CAWBG59, is the only New Zealand microcystin producer to have its genome sequenced. Further genetic sequencing of cyanobacteria strains from New Zealand may provide novel data that will assist in understanding toxin production; e.g. D. lemmemnanii from New Zealand contains at least one of the genes involved in microcystin production but does not produce the toxin, and Microcystis CAWBG11 produces a unique profile of microcystin congeners compared to other microcystin-producing cyanobacteria.
Whilst there is toxicology information for many of the microcystin congeners prevalent in New Zealand (e.g. MC-RR, MC-LR, [Dha7] MC-LR, MC-LA), some of the microcystin congeners observed in New Zealand freshwater environments lack toxicity data (e.g. MC-RA, MC-FA, MC-WA, MC-LAba). Additional work on the toxicity of microcystin congeners most relevant to New Zealand would help to ensure that the thresholds set in New Zealand’s recreational freshwater guidelines for cyanobacteria (Ministry for the Environment and Ministry of Health Citation2009) and drinking water standards (Ministry of Health Citation2005) are robust.
Research on the ecological impact of microcystins in New Zealand is limited. There are many knowledge gaps; e.g. the accumulation of microcystins through the food-web, the impact on native New Zealand species, whether protein-bound microcystins are present in our aquatic organisms, and whether microcystins accumulate in New Zealand’s marine organisms. More research is also needed on the downstream impacts of microcystin-containing blooms, in particular at the freshwater/marine interface. In addition to exploring lake outlets, attention should be given to the outlets of oxidation ponds. Wood et al. (Citation2006b) highlighted that these often contain microcystins, and many flow into estuaries and streams in New Zealand.
Conclusions
The research undertaken in New Zealand, has demonstrated that microcystins are relatively widely distributed and, as with the rest of the world, the most commonly detected cyanotoxin in planktonic samples. Novel and world-first findings have been generated in New Zealand; e.g. showing that microcystin are not always constitutively produced in cyanobacterial blooms (Wood et al. Citation2011), the characterisation of new microcystins (Puddick et al. Citation2013a, Citation2013b, Citation2014), and the identification of an extremely high number of congeners from a single Microcystis strain (Puddick et al. Citation2014). Two decades of data on microcystins has established that two microcystin congener profiles are predominantly produced by New Zealand strains of Microcystis. Because many of the microcystin congeners present in one of these Microcystis strains were only identified in 2013 they were likely to have been missed during previous studies and microcystin analysis methods in New Zealand should be adapted to include these congeners. There was a high level of variability in microcystin cell quotas from New Zealand Microcystis and the microcystin quotas were generally higher than those reported in overseas studies. This highlights the need for microcystin risk assessments to be based on regional data in order to be effective in protecting human health. Research on the ecological impact of microcystins is limited but shows the toxin can accumulate in native species, and when concentrations are sufficiently high have a negative effect on survival and behaviour.
Disclosure statement
No potential conflict of interest was reported by the authors.
Additional information
Funding
References
- Azevedo SM, Carmichael WW, Jochimsen EM, Rinehart KL, Lau S, Shaw GR, Eaglesham GK. 2002. Human intoxication by microcystins during renal dialysis treatment in Caruaru - Brazil. Toxicolo. 181–182:441–446.
- Beattie KA, Kaya K, Sano T, Codd GA. 1998. Three dehydrobutyrine-containing microcystins from Nostoc. Phytochem. 47(7):1289–1292.
- Bishop CT, Anet EFLJ, Gorham PR. 1959. Isolation and identification of the fast-death factor in Microcystis aeruginosa NRC-1. Canadian J Biochem Phys. 37(3):453–471.
- Buratti FM, Manganelli M, Vichi S, Stefanelli M, Scardala S, Testai E, Funari E. 2017. Cyanotoxins: producing organisms, occurrence, toxicity, mechanism of action and human health toxicological risk evaluation. Arch Toxicol. 91(3):1049–1130.
- Carmichael WW. 2001. Health effects of toxin-producing cyanobacteria: “The CyanoHABs”. Hum Ecol Risk Assess: Int J. 7(5):1393–1407.
- Chorus I, Bartram J. 1999. Toxic cyanobacteria in water: a guide to their public health consequences, monitoring and management. London: F & E Spon.
- Christoffersen K, Burns CW. 2001. Toxic cyanobacteria in New Zealand lakes and toxicity to indigenous zooplankton. In: Williams WD, editor. International Association of Theoretical and Applied Limnology, Vol 27, Pt 5, Proceedings. p. 3222–3225.
- Clearwater SJ, Wood SA, Phillips NR, Parkyn SM, Van Ginkel R, Thompson KJ. 2014. Toxicity thresholds for juvenile freshwater mussels Echyridella menziesii and crayfish Paranephrops planifrons, after acute or chronic exposure to Microcystis sp. Environ Toxicol. 29(5):487–502.
- Cordeiro-Araújo MK, Bittencourt-Oliveira M. 2013. Active release of microcystins controlled by an endogenous rhythm in the cyanobacterium Microcystis aeruginosa. Phycol Res. 61(1):1–6.
- Crush JR, Briggs LR, Sprosen JM, Nichols SN. 2008. Effect of irrigation with lake water containing microcystins on microcystin content and growth of ryegrass, clover, rape, and lettuce. Environ Toxicol. 23(2):246–252.
- Diehnelt CW, Dugan NR, Peterman SM, Budde WL. 2006. Identification of microcystin toxins from a strain of Microcystis aeruginosa by liquid chromatography introduction into a hybrid linear ion trap-Fourier transform ion cyclotron resonance mass spectrometer. Anal Chem. 78(2):501–512.
- Falconer IR, Beresford AM, Runnegar MT. 1983. Evidence of liver damage by toxin from a bloom of the blue-green alga, Microcystis aeruginosa. Med J Australia. 1(11):511–514.
- Ferrão-Filho A, Kozlowsky-Suzuki B. 2011. Cyanotoxins: bioaccumulation and effects on aquatic animals. Mar Drugs. 9(12):2729–2772.
- Fewer DP, Rouhiainen L, Jokela J, Wahlsten M, Laakso K, Wang H, Sivonen K. 2007. Recurrent adenylation domain replacement in the microcystin synthetase gene cluster. BMC Evol Biol. 7(1):183
- Fischer WJ, Garthwaite I, Miles CO, Ross KM, Aggen JB, Chamberlin AR, Towers NR, Dietrich DR. 2001. Congener-independent immunoassay for microcystins and nodularins. Environ Sci Technol. 35(24):4849–4856.
- Gibble CM, Peacock MB, Kudela RM. 2016. Evidence of freshwater algal toxins in marine shellfish: implications for human and aquatic health. Harmful Algae. 59:59–66.
- Hamill KD. 2001. Toxicity in benthic freshwater cyanobacteria (blue-green algae): first observations in New Zealand. New Zeal J Mar Fresh. 35(5):1057–1059.
- Harke MJ, Gobler CJ. 2013. Global transcriptional responses of the toxic cyanobacterium, Microcystis aeruginosa, to nitrogen stress, phosphorus stress, and growth on organic matter. PLOS ONE. 8(7):e69834.
- Harke MJ, Steffen MM, Gobler CJ, Otten TG, Wilhelm SW, Wood SA, Paerl HW. 2016. A review of the global ecology, genomics, and biogeography of the toxic cyanobacterium, Microcystis spp. Harmful Algae. 54:4–20.
- Holland P, Mountford D, Malarvannan G, Briggs L, Sprosen J. 2005. Progress report on determination of microcystins in Pacific Oyster. Cawthron Report 993.
- Horst GP, Sarnelle O, White JD, Hamilton SK, Kaul RB, Bressie JD. 2014. Nitrogen availability increases the toxin quota of a harmful cyanobacterium, Microcystis aeruginosa. Water Res. 54:188–198.
- Hummert C, Dahlmann J, Reinhardt K, Dang HPH, Dang DK, Luckas B. 2001. Liquid chromatography-mass spectrometry identification of microcystins in Microcystis aeruginosa strain from lake Thanh Cong, Hanoi, Vietnam. Chromatographia. 54(9):569–575.
- Kleinteich J, Puddick J, Wood SA, Hildebrand F, Laughinghouse IV H, Pearce D, Dietrich D, Wilmotte A. 2018. Toxic cyanobacteria in Svalbard: Chemical diversity of microcystins detected using a liquid chromatography mass spectrometry precursor ion screening method. Toxins. 10(4):147.
- Kuniyoshi TM, Sevilla E, Bes MT, Fillat MF, Peleato ML. 2013. Phosphate deficiency (N/P 40:1) induces mcyD transcription and microcystin synthesis in Microcystis aeruginosa PCC7806. Plant Physiol Bioch. 65:120–124.
- Li Y, Chen J-A, Zhao Q, Pu C, Qiu Z, Zhang R, Shu W. 2011. A cross-sectional investigation of chronic exposure to microcystin in relationship to childhood liver damage in the three gorges reservoir region, China. Environ Health Persp. 119(10):1483–1488.
- MacKintosh C, Beattie KA, Klumpp S, Cohen P, Codd GA. 1990. Cyanobacterial microcystin-LR is a potent and specific inhibitor of protein phosphatases 1 and 2A from both mammals and higher plants. FEBS Lett. 264(2):187–192.
- McAllister TG, Wood SA, Hawes I. 2016. The rise of toxic benthic Phormidium proliferations: a review of their taxonomy, distribution, toxin content and factors regulating prevalence and increased severity. Harmful Algae. 55:282–294.
- Meriluoto J, Spoof L, Codd GA. 2016. Handbook of cyanobacterial monitoring and cyanotoxin analysis. Chichester, UK: John Wiley & Sons.
- Meriluoto JA, Spoof LE. 2008. Cyanotoxins: sampling, sample processing and toxin uptake. Cyanobacterial Harmful Algal Blooms: State of the Science and Research Needs. Springer. 619:483–499.
- Miles CO, Melanson JE, Ballot A. 2014. Sulfide oxidations for LC-MS analysis of methionine-containing microcystins in Dolichospermum flos-aquae NIVA-CYA 656. Environ Sci Technol. 48(22):13307–13315.
- Miller MA, Kudela RM, Mekebri A, Crane D, Oates SC, Tinker MT, Staedler M, Miller WA, Toy-Choutka S, Dominik C, et al. 2010. Evidence for a novel marine harmful algal bloom: cyanotoxin (microcystin) transfer from land to sea otters. PLOS ONE. 5(9):e12576.
- Ministry for the Environment and Ministry of Health. 2009. New Zealand guidelines for cyanobacteria in recreational fresh waters – Interim Guidelines. Prepared for the Ministry for the Environment and the Ministry of Health by SA Wood, DP Hamilton, WJ Paul, KA Safi and WM Williamson. Wellington: Ministry for the Environment.
- Ministry of Health. 2005. Drinking-water standards for New Zealand 2005. Wellington: Ministry of Health.
- Mountfort DO, Holland P, Sprosen J. 2005. Method for detecting classes of microcystins by combination of protein phosphatase inhibition assay and ELISA: comparison with LC-MS. Toxicon. 45(2):199–206.
- Nishizawa T, Ueda A, Asayama M, Fujii K, Harada K-i, Ochi K, Shirai M. 2000. Polyketide synthase gene coupled to the peptide synthetase module involved in the biosynthesis of the cyclic heptapeptide microcystin. J Biochem. 127(5):779–789.
- Pancrace C, Barny M-A, Ueoka R, Calteau A, Scalvenzi T, Pédron J, Barbe V, Piel J, Humbert J-F, Gugger M. 2017. Insights into the Planktothrix genus: genomic and metabolic comparison of benthic and planktic strains. Sci Rep. 7:41181.
- Pham T-L, Utsumi M. 2018. An overview of the accumulation of microcystins in aquatic ecosystems. J Env Man. 213:520–529.
- Phelan RR, Downing TG. 2011. A growth advantage for microcystin production by Microcystis PCC7806 under high light. J Phycol. 47(6):1241–1246.
- Puddick J, Prinsep M, Wood S, Kaufononga S, Cary S, Hamilton D. 2014. High levels of structural diversity observed in microcystins from Microcystis CAWBG11 and characterization of six new microcystin congeners. Mar Drugs. 12(11):5372–5395.
- Puddick J, Prinsep M, Wood S, Miles C, Rise F, Cary S, Hamilton D, Wilkins A. 2013a. Structural characterization of new microcystins containing tryptophan and oxidized tryptophan residues. Mar Drugs. 11(8):3025–3045.
- Puddick J, Prinsep M, Wood SA, Cary S, Hamilton DP, Holland PT. 2015. Further characterization of glycine-containing microcystins from the McMurdo Dry Valleys of Antarctica. Toxins. 7(2):493–515.
- Puddick J, Prinsep MR. 2008. MALDI-TOF mass spectrometry of cyanobacteria: a global approach to the discovery of novel secondary metabolites. Chem New Zealand. 72(2):68–71.
- Puddick J, Prinsep MR, Wood SA, Cary SC, Hamilton DP. 2016a. Modulation of microcystin congener abundance following nitrogen depletion of a Microcystis batch culture. Aquat Ecol. 50(2):235–246.
- Puddick J, Prinsep MR, Wood SA, Cary SC, Hamilton DP, Wilkins AL. 2013b. Isolation and structure determination of two new hydrophobic microcystins from Microcystis sp. (CAWBG11). Phytochem Lett. 6(4):575–581.
- Puddick J, Wood SA, Hawes I, Hamilton DP. 2016b. Fine-scale cryogenic sampling of planktonic microbial communities: application to toxic cyanobacterial blooms. Limnol Oceanogr: Meth. 14(9):600–609.
- Ressom R, Soong F, Fitzgerald D, Turczynowicz L, Saadi O, Roder D, Maynard T, Falconer I. 1994. Health effects of toxic cyanobacteria (blue-green algae). Canberra: National Health and Medical Research Council.
- Rogers S. 2014. Investigation of microcystin processing, production and export by Microcystis sp. [MSc Thesis]. Hamilton (NZ): University of Waikato.
- Rueckert A, Wood SA, Cary SC. 2007. Development and field assessment of a quantitative PCR for the detection and enumeration of the noxious bloom-former Anabaena planktonica. Limnol Oceanogr: Meth. 5(12):474–483.
- Sabart M, Misson B, Descroix A, Duffaud E, Combourieu B, Salençon M-J, Latour D. 2013. The importance of small colonies in sustaining Microcystis population exposed to mixing conditions: an exploration through colony size, genotypic composition and toxic potential. Env Microbiol Rep. 5(5):747–756.
- Saker ML, Fastner J, Dittmann E, Christiansen G, Vasconcelos VM. 2005. Variation between strains of the cyanobacterium Microcystis aeruginosa isolated from a Portuguese river. J Appl Microbiol. 99(4):749–757.
- Somdee T, Thunders M, Ruck J, Lys I, Allison M, Page R. 2013. Degradation of [Dha7] MC-LR by a microcystin degrading bacterium isolated from Lake Rotoiti, New Zealand. ISRN Microbiol. Article ID 596429.
- Steiner K, Wood SA, Puddick J, Hawes I, Dietrich DR, Hamilton DP. 2017. A comparison of bacterial community structure, activity and microcystins associated with formation and breakdown of a cyanobacterial scum. Aquat Microb Ecol. 80(3):243–256.
- Stirling DJ, Quilliam MA. 2001. First report of the cyanobacterial toxin cylindrospermopsin in New Zealand. Toxicon. 39(8):1219–1222.
- Svirčev Z, Drobac D, Tokodi N, Vidovič M, Simeunovič J, Miladinov-Mikov M, Baltič V. 2013. Epidemiology of primary liver cancer in Serbia and possible connection with cyanobacterial blooms. J Environ Sci Heal C. 31(3):181–200.
- Svirčev Z, Krstič S, Miladinov-Mikov M, Baltič V, Vidovič M. 2009. Freshwater cyanobacterial blooms and primary liver cancer epidemiological studies in Serbia. J Environ Sci Heal C. 27(1):36–55.
- Teixeira M, Costa M, de Carvalho V, Pereira M, Hage E. 1993. Gastroenteritis epidemic in the area of the Itaparica Dam, Bahia, Brazil. Bulletin of the Pan American Health Organization. 27:244–253.
- Tillett D, Dittmann E, Erhard M, von Döhren H, Börner T, Neilan BA. 2000. Structural organization of microcystin biosynthesis in Microcystis aeruginosa PCC7806: an integrated peptide-polyketide synthetase system. Chem Biol. 7(10):753–764.
- Ueno Y, Nagata S, Tsutsumi T, Hasegawa A, Watanabe MF, Park H-D, Chen G-C, Chen G, Yu S-Z. 1996. Detection of microcystins, a blue-green algal hepatotoxin, in drinking water sampled in Haimen and Fusui, endemic areas of primary liver cancer in China, by highly sensitive immunoassay. Carcinogen. 17(6):1317–1321.
- Vezie C, Brient L, Sivonen K, Bertru G, Lefeuvre J-C, Salkinoja-Salonen M. 1998. Variation of microcystin content of cyanobacterial blooms and isolated strains in Lake Grand-Lieu (France). Microb Ecol. 35(2):126–135.
- Wall JM, Wood SA, Orlovich DA, Rhodes LL, Summerfield TC. 2013. Characterisation of freshwater and marine cyanobacteria in the Hokianga region, Northland, New Zealand. New Zeal J Mar Fresh. 48(2):177–193.
- Weller DI. 2011. Detection, identification and toxigenicity of cyanobacteria in New Zealand lakes using PCR-based methods. New Zeal J Mar Fresh. 45(4):651–664.
- Wilding TK. 2000. Rotorua lakes algae report. Whakatane, NZ: Environment Bay of Plenty. p. 98.
- Wood SA, Borges H, Puddick J, Biessy L, Atalah J, Hawes I, Dietrich DR, Hamilton DP. 2017a. Contrasting cyanobacterial communities and microcystin concentrations in summers with extreme weather events: insights into potential effects of climate change. Hydrobiologia. 785(1):71–89.
- Wood SA, Briggs LR, Sprosen J, Ruck JG, Wear RG, Holland PT, Bloxham M. 2006a. Changes in concentrations of microcystins in rainbow trout, freshwater mussels, and cyanobacteria in lakes Rotoiti and Rotoehu. Environ Toxicol. 21(3):205–222.
- Wood SA, Dietrich DR. 2011. Quantitative assessment of aerosolized cyanobacterial toxins at two New Zealand lakes. J Environ Monitor. 13(6):1617–1624.
- Wood SA, Dietrich DR, Cary SC, Hamilton DP. 2012. Increasing Microcystis cell density enhances microcystin synthesis: A mesocosm study. Inland Waters. 2(1):17–22.
- Wood SA, Heath MW, Holland PT, Munday R, McGregor GB, Ryan KG. 2010. Identification of a benthic microcystin-producing filamentous cyanobacterium (Oscillatoriales) associated with a dog poisoning in New Zealand. Toxicon. 55(4):897–903.
- Wood SA, Holland PT, Stirling DJ, Briggs LR, Sprosen J, Ruck JG, Wear RG. 2006b. Survey of cyanotoxins in New Zealand water bodies between 2001 and 2004. New Zeal J Mar Fresh. 40(4):585–597.
- Wood SA, Maier MY, Puddick J, Pochon X, Zaiko A, Dietrich DR, Hamilton DP. 2017b. Trophic state and geographic gradients influence planktonic cyanobacterial diversity and distribution in New Zealand lakes. FEMS Microbiol Ecol. 93(2): fiw234.
- Wood SA, Mountfort D, Selwood AI, Holland PT, Puddick J, Cary SC. 2008a. Widespread distribution and identification of eight novel microcystins in Antarctic cyanobacterial mats. Appl Environ Microb. 74(23):7243–7251.
- Wood SA, Pochon X, Luttringer-Plu L, Vant BN, Hamilton DP. 2014. Recent invader or indicator of environmental change? A phylogenetic and ecological study of Cylindrospermopsis raciborskii in New Zealand. Harmful Algae. 39:64–74.
- Wood SA, Rhodes LL, Adams SL, Adamson JE, Smith KF, Smith JF, Tervit HR, Cary SC. 2008b. Maintenance of cyanotoxin production by cryopreserved cyanobacteria in the New Zealand culture collection. New Zeal J Mar Fresh. 42(3):277–283.
- Wood SA, Rueckert A, Hamilton DP, Cary SC, Dietrich DR. 2011. Switching toxin production on and off: Intermittent microcystin synthesis in a Microcystis bloom. Env Microbiol Rep. 3(1):118–124.
- Yu G, Jiang Y, Song G, Tan W, Zhu M, Li R. 2014. Variation of Microcystis and microcystins coupling nitrogen and phosphorus nutrients in Lake Erhai, a drinking-water source in Southwest Plateau, China. Environ Sci Pollut R. 21(16):9887–9898.
- Zhang F, Lee J, Liang S, Shum C. 2015. Cyanobacteria blooms and non-alcoholic liver disease: evidence from a county level ecological study in the United States. Envir Heal. 14(1):41.