ABSTRACT
Coastal pollution is complex, often consisting of multiple contaminants, and it is increasingly recognised that exposure to combinations of pollutants can produce different outcomes than each does separately. This study investigates the toxicity of three major pollutants in coastal environments (copper, lead and zinc) individually and in binary mixtures on larvae of Evechinus chloroticus, a sea urchin endemic to New Zealand. Larval development assays were conducted for 72 h. Median effective concentrations (EC50) for normal larval development for individual metals were 5.4 μg/L Cu, 52.2 μg/L Pb and 27.7 μg/L Zn. Evechinus chloroticus was more sensitive to copper and zinc than most echinoid species tested to date. Effects of metal mixtures were analysed using a toxic unit (TU) approach. Cu + Zn and Cu + Pb had a less-than-additive effect on E. chloroticus larval development. By contrast, Zn + Pb was strictly additive. None of the interactions were strong, with sums of TU ranging from 1.00 to 1.85. These results support the use of criteria based on strictly-additive models to determine whether these three metals exceed water quality thresholds when occurring in a simple mixture.
Introduction
Pollution is one of the major threats to coastal systems. Because of increased awareness of the risks pollution causes to marine organisms and human health, many countries have developed routine monitoring programmes to keep track of pollutants present in coastal waters (Milne Citation2006; Osman Citation2010; Roose et al. Citation2011; Engle Citation2012), and metals are among the contaminants most commonly found in concentrations toxic to marine life (US EPA Citation2014). Metal contamination in coastal waters is highly variable and can be found in concentrations two orders of magnitude higher than occurs naturally (Govindasamy et al. Citation1998; Fatoki & Mathabatha Citation2001; Gorski & Nugegoda Citation2006; Gadd & Cameron Citation2012; SFEI Citation2015).
Copper and lead are among the most common metal contaminants, found in high concentration in many coastal areas, especially those affected by urban and industrial discharges or mining activities (Fatoki & Mathabatha Citation2001; Kobayashi & Okamura Citation2004; SFEI Citation2015). They are considered as priority pollutants by environmental authorities due to their high toxicity to aquatic life (ANZECC Citation2000; US EPA Citation2014). Zinc is generally not considered a priority pollutant (US EPA Citation2014), but its prevalence is increasing in many countries including New Zealand (Eisler Citation2007; Milne & Watts Citation2008; Neale Citation2009; SFEI Citation2015). For example, a study on complex urban effluents identified copper, lead and zinc as responsible for most of the toxicity in surface sediments from Sydney Harbour (McCready et al. Citation2006).
The regulation concerning the acceptable levels of pollutants in New Zealand waters is based on the Australian and New Zealand Environment and Conservation Council (ANZECC) water quality criteria (WQC) (ANZECC Citation2000). These criteria, however, have been developed mainly using studies on Australian species; few studies on local species are available. Nevertheless, these three metals, copper, lead and zinc, are commonly found in urban effluents at levels that exceed WQC for 90% species protection (ANZECC Citation2000; Milne & Watts Citation2008; Neale Citation2009).
New Zealand is a highly isolated system with a high endemism rate, especially among invertebrates and coastal fish with short range dispersal (MacDiarmid Citation2007). Land-use impacts including pollution and sedimentation have been identified as major threats to coastal invertebrates (Morrison et al. Citation2009; Freeman et al. Citation2010). Given New Zealand’s extensive coastline of more than 15,000 km, and valuable coastal fisheries, it is particularly important to verify whether the WQC are appropriate for local species, although very few studies have done so. Fukunaga et al. (Citation2010, Citation2011) found adverse effects of copper and zinc in sediments on infaunal abundance and species richness in Auckland; however, studies on impacts of waterborne pollutants are rare.
Exposure to more than one toxicant, as will commonly be the case for organisms in polluted coastal environments, often produces a different outcome than exposure to each does separately (King et al. Citation2006; Mann et al. Citation2009; Manzo et al. Citation2010; Xu et al. Citation2011). Effects are complex and variable, even for the same metal pair on different organisms. In a review of more than 60 studies on aquatic organisms, 44% of the studies reported a less-than-additive effect of metal mixtures while 27% reported a strictly-additive effect and 29% reported a more-than-additive effect (Norwood et al. Citation2003). This illustrates the difficulty of predicting the outcome of toxicants in mixture.
Concentration addition models predict the toxicity of a mixture using the relative potency of each component, and their concentration in the mixture, assuming a strictly-additive effect. Deviation from the model indicates an interaction between components of the mixture (Norwood et al. Citation2003; Newman Citation2012; Beyer et al. Citation2014). These models are appropriate to analyse mixtures of toxicants with a similar mode of action (e.g. disruption of the endocrinal system; Faust et al. Citation2001). Copper, lead and zinc all appear to elicit similar physiological responses in marine organisms (Grosell et al. Citation2002; Franco et al. Citation2006; Eisler Citation2007; Zizza et al. Citation2013), especially in their disruption of ion transport and calcium absorption, supporting the use of concentration addition models to evaluate how they act in combination. In this study, copper, lead and zinc binary mixtures were evaluated using a commonly used concentration addition model: the toxic unit (TU) method first described by Sprague (Citation1970).
Although many pollutants interact with each other, water quality guidelines rarely take into account the presence of more than one pollutant. Most countries, including European nations, US and South Africa, provide guidelines only for individual pollutants (Warne Citation2003; Beyer et al. Citation2014). These WQC are based on a range of studies on various species using mostly a single pollutant and make no provision for the presence of pollutants in mixture. ANZECC, on the other hand, has implemented a formal threshold based on the concentration addition approach to predict whether a simple metal mixture (less than six toxicants) exceeds WQC:where TTM is the predicted total toxicity of the mixture, Ci is the concentration of the compound and WQCi is the guideline for that compound. The mixture is considered to exceed the WQC if TTM is greater than 1 (ANZECC Citation2000). This criterion assumes simple additivity of the toxic effect of the mixture and does not account for potential synergism.
Decades of ecotoxicity studies have revealed that sensitivity to particular pollutants is strongly species-specific (Grosell et al. Citation2007; US EPA Citation2007). Toxic effects can span several orders of magnitude and vary with the life stage tested, size of organisms and physiology (Grosell et al. Citation2007). For many aquatic species, early life stages are the most vulnerable to environmental stressors, and therefore embryos and larvae are commonly used to determine the sensitivity of organisms to pollutants (Kobayashi Citation1980; Anderson et al. Citation1994; Fitzpatrick et al. Citation2008). Despite being short term (hours to days), these tests are presumed to be ecologically relevant because mortality at the larval stage impacts recruitment to adult populations and even sublethal impacts can increase the probability of later mortality, and persist after exposure, causing mortality or imparing reproduction later in life (Conroy et al. Citation1996; Raimondo & McKenney Citation2005; Tellis et al. Citation2014).
Sea urchins, echinoderms in the class Echinoidea, are commonly used in ecotoxicity assays around the world due to the ease of handling and spawning in the laboratory, and their high sensitivity to pollutants, which makes them good indicator species for marine systems (US EPA Citation2002; ASTM Citation2012). Importantly, urchins are also common and ecologically important members of subtidal reef communities that are likely to be impacted by coastal runoff. However, most studies have been limited to species from the Strongylocentrotus or Paracentrotus genera (Fernández & Beiras Citation2001; Novelli et al. Citation2003; Phillips et al. Citation2003; Nadella et al. Citation2013), and the vulnerability of other genera may be quite different. For example, the Antarctic sea urchin Sterechinus neumayeri was substantially more vulnerable to copper than any other previously tested sea urchin species (King & Riddle Citation2001). Further, few studies have evaluated metal mixtures on larval development of sea urchins (but see Fernández & Beiras Citation2001; Phillips et al. Citation2003; Kobayashi & Okamura Citation2005; Xu et al. Citation2011).
Evechinus chloroticus is an endemic species of sea urchin in New Zealand, of ecological, cultural and economic importance (Andrew Citation1988; MacDiarmid Citation2007; Barker Citation2013). It is a shallow subtidal species primarily found on hard substrates and is distributed around the New Zealand mainland and Snares and Chatham Islands (Dix Citation1969; Shears & Babcock Citation2007). As a dominant grazer, E. chloroticus is a major determinant of community structure of subtidal rocky reef by mediating the abundance of macroalgae (Andrew Citation1988), including the formation of urchin barrens (Schiel Citation1990; Villouta et al. Citation2001; Shears & Babcock Citation2003). Evechinus chloroticus entered the commercial fishery Quota Management System in 2002, with the catch in 2009 worth approximately NZ$5 million in the primarily domestic market (Miller & Abraham Citation2011). Because E. chloroticus are highly significant for Māori, there are also important recreational and customary fisheries, although data on landings are scarce (Miller & Abraham Citation2011).
Evechinus chloroticus reproduces via broadcast spawning from austral late spring to early fall, with variation among populations (Dix Citation1970; Walker Citation1984). Feeding, planktonic larvae are produced that take several weeks to develop, with variability in development time dependent on food availability and temperature (Dix Citation1969; Lamare & Barker Citation1999, Citation2001). It is the only species of its genus and is phylogenetically distant from other member of the Echinometridae family, which consists of nine genera (Gillard et al. Citation2014). Responses of E. chloroticus to pollutants have never been investigated, and only two species of the closest related genus Heliocidaris have been tested for metal sensitivity (Kobayashi Citation1980; Kobayashi & Okamura Citation2005). Given the species-specific nature of the response to pollutants, it is important to verify whether WQC are appropriate to protect this locally valuable species.
The aims of this study were to: 1. evaluate the toxicity of common metal pollutants in mixtures; 2. compare the vulnerability of a species of a locally important sea urchin that has never before been tested with that of related species; and 3. confirm the suitability of concentration addition models underlying WQC for mixtures. This was done using standard biotoxicity assays on E. chloroticus larval stages.
Methods
Metal solutions
Metal stock solutions were made from reagent grade metal salts, namely copper sulphate (CuSO4 · 5H2O), zinc sulphate (ZnSO4 · 7H2O) and lead nitrate (Pb[NO3]2). Stock solutions were analysed by Eurofins Environmental Laboratory Services Ltd, Lower Hutt, New Zealand, by inductively coupled mass spectrometry and were within 96% recovery for copper, 105% for lead and 110% for zinc.
The four levels of metal concentrations used in the experimental assays were: 1, 5, 10 and 20 μg/L for copper; 10, 20, 50 and 100 μg/L for lead; and 5, 10, 30 and 60 μg/L for zinc. These same levels were used in combined metal experiments (Cu + Zn, Cu + Pb and Zn + Pb). In the combined metal experiments, each metal pair (Cu + Zn, Cu + Pb and Zn + Pb) was tested at four levels for a total of 12 binary mixtures (). These values were based on a range-finding pilot trial, and the current experiments were designed to have one concentration producing no toxic effect, one producing close to 100% abnormal larvae and two concentrations producing intermediate effects as required for accurate estimation of EC50s (US EPA Citation2002). There were three replicate jars for each metal level and control, plus an additional jar in control conditions (filtered seawater [FSW]) and one in the highest metal level for each metal, in which to monitor pH and temperature.
Table 1. Copper (Cu), lead (Pb) and zinc (Zn) concentrations (μg/L) used in each level of the combined metals assays.
All reported concentrations in this study are nominal as dissolved metal concentrations were not measured. Metal loss may occur due to low solubility in seawater, leading to an actual metal concentration potentially lower than the one reported. However, previous studies have shown a good agreement between nominal and dissolved concentrations for copper and zinc in seawater at the low concentration range used during this study (Fernández & Beiras Citation2001; Phillips et al. Citation2003; Bielmyer et al. Citation2005). Metal loss is a more important issue with lead, especially at high concentrations. However, recovery after 48 h remained above 88%, up to 512 μg/L Pb (Fernández & Bieras Citation2001). In the present study, the maximum concentration used was 100 μg/L Pb. The difference between nominal and actual concentration for these metals are almost certainly minimal and the resulting difference in EC values would be well within the natural variation of response to contaminants. Indeed, EC50s in echinoderms commonly vary by 20%–40% between cohorts (King & Riddle Citation2001; Manzo Citation2004; Manzo et al. Citation2008).
Bioassays procedure
Adult E. chloroticus (8–20 cm test diameter) were collected from sites around Wellington, New Zealand, in October 2011 and October 2013. A total of 25 adults were kept at Victoria University Coastal Ecology Laboratory (VUCEL) in a 200 L tank with flow-through raw seawater. Raw seawater receives no treatment while FSW is pre-filtered (15 μm) and then filtered through a 0.2 μm paper cartridge. Adults were fed twice a week with native kelp (Macrocystis pyrifera) or commercial feed (ABMAX, E.N. Hutchinson Ltd.). Single metal tests were run in February 2012 and again in January 2014; combined metal tests were done in January 2012. For each spawning, three to four individuals each of males and females were haphazardly selected. Adults were used only once to produce embryos. Spawning was induced by injecting 3 mL of 0.5 M potassium chloride into the abdominal cavity through the perioral membrane (ASTM Citation2012). Eggs were collected by inverting females over a beaker of FSW, and sperm was collected ‘dry’ and placed on ice until fertilisation. Gametes of each spawning adult were collected separately then pooled in similar quantity to produce a composite sperm and egg stock.
Fertilisation occurred less than 1 h after spawning in 500 mL FSW in the presence of a high concentration of sperm (estimated sperm concentration 106–108 sperm/mL, approximate egg:sperm ratio 1:1000). The culture was then rinsed twice with FSW to remove excess sperm. Evechinus chloroticus embryos were placed in glass jars with 300 mL FSW before first cell division, no more than 1 h after fertilisation. The fertilisation rate was above 90%. An initial density of 30 embryos/mL ensured no overcrowding occurred (ASTM Citation2012) and yielded excellent survival rates (≥ 80%) in pilot studies. All glassware was acid-washed and rinsed in distilled water before use.
After 72 h incubation at 16 ± 1 °C, E. chloroticus larvae had reached the pluteus stage. Larvae in all jars, except the temperature and pH control jars, were collected and preserved in 40% ethanol. Endpoints were proportion of normal larvae (n = 100) and larval size (n = 10). For developmental abnormalities, larvae and embryos were rated into one of seven developmental categories: two normal categories (four-armed pluteus and two-armed pluteus); and six abnormal categories (pluteus with skeletal abnomalies [e.g. asymmetric, missing or forked skeletal rod], pluteus with stunted postoral arms, arrested development at the prism stage, arrested development at the gastrula stage, egg or unhatched embryo, and severe abnormalities [e.g. individuals with more than one abnormality or where the developmental stage was not recognisable]). Two-armed pluteus were considered normal as 57% of the larvae were still at this stage after 72 h in controls. Larval size was measured at 100× magnification (body length [BL] and postoral arm length [PO]; ).
Figure 1. Four-armed pluteus 72 h post-fertilisation. AL, anterolateral arms; BL, total body length; PO, postoral arms.
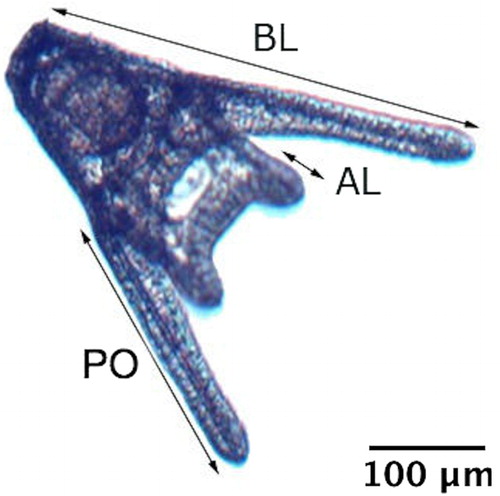
In the single metal tests, a total of three samples were accidentally lost: one sample of 20 μg/L Pb; one of 30 μg/L Zn; and one of 60 μg/L Zn.
Statistical analyses
Single metals
All data analyses were based on US Environmental Protection Agency (EPA) methods (US EPA Citation2002). Data from the two separate runs for single metal assays were pooled as both EC50s and pluteus size were not significantly different between the two runs. All abnormal categories were pooled to determine the overall toxic effect. Both point estimation techniques and hypothesis testing were used.
A probit regression was used to estimate EC50s (median effective concentration), EC10s (effective concentration affecting more than 10% of the population) and their respective 95% confidence intervals (CI). These probit regressions were also used to estimate the proportion of abnormal larvae at metal concentrations of special interest, such as WQC level.
The overall effect of metal on normal development was analysed using one-way ANOVAs. Lowest observable effect concentration (LOEC; i.e. lowest tested concentration at which toxic effects were observed) was determined for both the proportion of normal development (arcsine transformed) and pluteus size (BL and PO/BL ratio) using Dunnett’s pairwise comparison. Minimum significant differences (MSD) were calculated following US EPA (Citation2002) methods and expressed as the percentage of the control’s mean to give a measure of Dunnett’s test sensitivity. Larval size was averaged per replicate for all size data. The number of larvae measured per jar (n = 10) provided a power of 90% in the detection of differences of 25 μm in larval length. The occurrence of two-armed plutei among normal larvae was analysed using one-way ANOVAs.
Probit regressions were calculated in SPSS (v.22); all other tests were performed in R v3.1, package multcomp (Hothorn et al. Citation2008).
Metal mixtures
Metal interactions were evaluated using the TU approach described in Sprague (Citation1970). Each compound is given a TU determined from its concentration in the mixture and its toxic effect as a single toxicant. The chosen toxic effect was EC50 based on a probit regression as described for single metals.
The sum of TUs was calculated using the following equation:where n is the number of components in the mixture, ci is the individual concentration of the ith substance in the mixture at toxic effect level, and EC50i is the individual toxic effect of the ith substance.
A mixture was classified as strictly-additive if the sum of TUs = 1, and less-than-additive and more-than additive, respectively, if the sum of TUs > 1 and <1 (Faust et al. Citation2001; Norwood et al. Citation2003). In this study, the terms ‘less-than-additive’, ‘strictly-additive’ and ‘more-than-additive’ were used to describe the full spectrum of interaction as recommended in Van Genderen et al. (Citation2015). To give a measure of experimental variation, a range for the sum of TUs was determined using both extreme values of the EC50s’ 95% CI, obtained from the probit regression as described above.
Results
In all tests, the proportion of normal larvae in controls was a minimum of 92%. Salinity was 34 ppt and pH was 7.99 ± 0.2 in all tests, with no differences across treatments and controls.
Single metals
All of the metals tested significantly affected E. chloroticus larval development (one-way ANOVA; Cu: F(4, 25) = 159, P < 0.001; Pb: F(4, 24) = 63.4, P < 0.001; Zn: F(4, 23) = 61.5, P < 0.001).
Copper
The resulting 72 h EC50 was 5.4 μg/L Cu with 95% CI 3.9–7.1 μg/L Cu (A). LOEC based on normal development was 5 μg/L Cu (MSD: 3.9% of the control mean). The main abnormalities observed were skeletal abnormalities in the lowest concentration (1 μg/L), stunted larvae in the intermediate concentrations (5 μg/L and 10 μg/L), and arrested development at the gastrula stage in the highest concentration (20 μg/L). Furthermore, among normal larvae, the proportion of early stages (two-armed pluteus larvae) increased with metal concentration (ANOVA: F(1, 19) = 16.2, P < 0.001; B). In the control treatment, there were on average 57% two-armed plutei and 43% four-armed plutei, whereas at 10 µg/L of copper 100% of normal larvae were at the earlier stage.
Figure 2. Copper toxicity on larval development of the sea urchin Evechinus chloroticus after a 72 h exposure. A, Proportion of normal larvae out of 100. Solid line represents the probit model. Dashed line represents the median effective concentration (EC50, probit regression analysis). Shaded grey area represents the EC50s’ 95% CI. Dotted line represents current New Zealand water quality trigger values for 95% species protection (ANZECC Citation2000); B, proportion of two-armed plutei among normal larvae. No data points are shown when the total number of normal larvae was less than five. Error bars represent the standard deviation of the mean (n = 6).
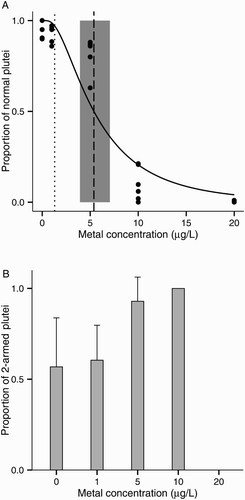
Copper significantly affected the size of normal pluteus larvae, both BL and PO/BL ratios had a LOEC of 5 μg/L Cu (). Larvae exposed to 5 μg/L Cu were 8% smaller (BL) than control larvae (A). The PO/BL ratio was also lower in larvae exposed to 5 μg/L Cu (mean PO/BL ratio: 0.58 vs. 0.60 in controls; B).
Figure 3. Copper toxicity on larval development of the sea urchin Evechinus chloroticus after a 72 h exposure. A, Body length; B, postoral arm/body length ratio (PO/BL). Asterisks mark treatments significantly different from control (Dunnett’s pairwise comparison, P < 0.05). Error bars represent the standard error of the mean (n = 6). No data points are shown when the total number of normal larvae was less than five.
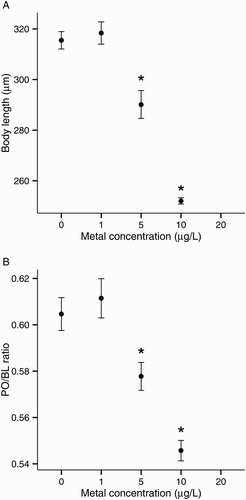
Lead
EC50 was 52.2 μg/L Pb (CI 39.6–73.0 μg/L Pb; A). LOEC based on normal development was 100 μg/L Pb (MSD: 5.2% of the control mean). The main abnormalities observed were skeletal abnormalities in the lower range of concentrations (10 μg/L and 20 μg/L) and stunted and arrested development at the prism stage from 50 μg/L. The proportion of two-armed plutei among normal larvae increased with metal concentration from 57% in controls to 100% at 100 µg/L (ANOVA: F(1, 22) = 14.3, P < 0.001; B).
Figure 4. Lead toxicity on larval development of the sea urchin Evechinus chloroticus after a 72 h exposure. A, Proportion of normal larvae out of 100. Solid line represents the probit model. Dashed line represents the median effective concentration (EC50, probit regression analysis). Shaded grey area represents the EC50s’ 95% CI. Dotted line represents current New Zealand water quality trigger values for 95% species protection (ANZECC Citation2000); B, proportion of two-armed plutei among normal larvae. No data points are shown when the total number of normal larvae was less than five. Error bars represent the standard deviation (n = 6).
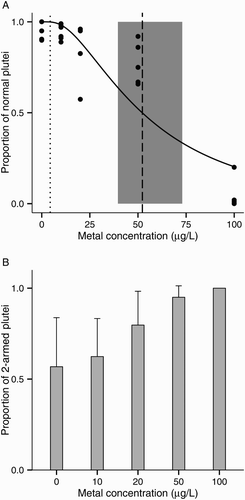
Effects on normal pluteus size were detectable from 50 μg/L Pb (). Plutei exposed to 50 μg/L Pb (LOEC) had a BL 7% smaller than did the control larvae (A) and had smaller PO arms relative to body size (PO/BL ratio: 0.57 vs. 0.60 in controls; B).
Figure 5. Lead toxicity on larval development of the sea urchin Evechinus chloroticus after a 72 h exposure. A, Body length; B, postoral arm/body length ratio (PO/BL). Asterisks mark treatments significantly different from control (Dunnett’s pairwise comparison, P < 0.05). Error bars represent the standard error of the mean (n = 6). No data points are shown when the total number of normal larvae was less than five.
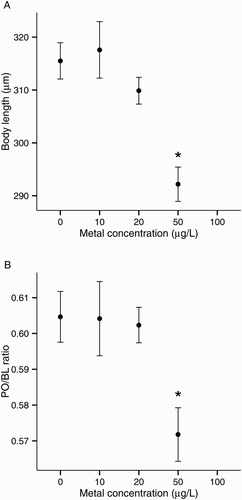
Zinc
EC50 was 27.7 μg/L Zn (CI 20.5–40.1 μg/L Zn; A). LOEC based on normal development was 30 μg/L Zn (MSD: 5.2% of the control mean). The main abnormalities observed were skeletal abnormalities in the lower range of concentrations (5 μg/L and 10 μg/L), stunted and arrested development at the prism stage at 30 μg/L, and severely abnormal larvae in the highest concentration tested (60 μg/L). Contrary to the other two metals tested, there was no difference in the proportion of two-armed plutei across increasing zinc concentrations (ANOVA: F(1, 21) = 2.38, P > 0.05; B).
Figure 6. Zinc toxicity on larval development of the sea urchin Evechinus chloroticus after a 72 h exposure. A, Proportion of normal larvae out of 100. Solid line represents the probit model. Dashed line represents the median effective concentration (EC50, probit regression analysis). Shaded grey area represents the EC50s’ 95% confidence interval. Dotted line represents current New Zealand water quality trigger values for 95% species protection (ANZECC Citation2000); B, proportion of two-armed plutei among normal larvae. No data points are shown when the total number of normal larvae was less than five. Error bars represent the standard deviation (n = 6).
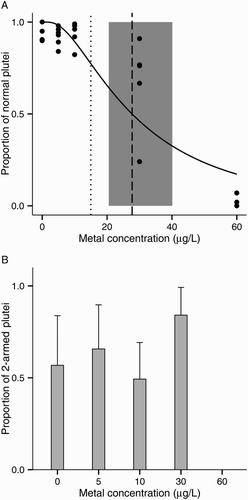
Zinc had a negative effect on larval BL but not on PO/BL ratio (). Plutei exposed to 30 μg/L Zn (LOEC based on size) were 11% smaller in BL than were the control larvae (A).
Figure 7. Zinc toxicity on larval development of the sea urchin Evechinus chloroticus after a 72 h exposure. A, Body length; B, postoral arm/body length ratio (PO/BL). Asterisks mark treatments significantly different from control (Dunnett’s pairwise comparison, P < 0.05). Error bars represent the standard error of the mean (n = 6). No data points are shown when the total number of normal larvae was less than five.
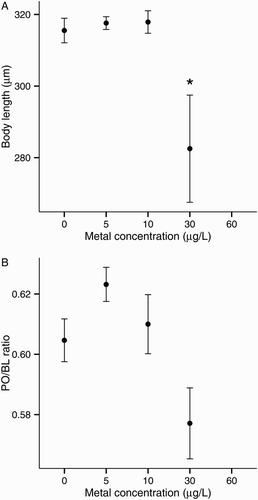
Metal mixtures
Normal developmental rates in mixture controls were 92 ± 4%. The sum of TUs and their range for each metal combination (four combinations for each pair) are shown in . Cu + Zn were less-than-additive with a sum of TUs of 1.85. They also produced a higher EC50 in combination (6.9 μg/L Cu, 95% CI 6.3–7.6 μg/L) than when the most toxic metal (i.e. copper) was present singly (5.4 μg/L Cu, 95% CI 3.9–7.0 μg/L). Cu + Pb had a less-than-additive effect and Zn + Pb mixtures were strictly-additive. The difference between the EC50s of the mixtures and the EC50 of the most toxic metal of the pair was always less than 30%.
Table 2. Toxic effect of metals in mixture based on toxic units (TUs).
Discussion
The results of this study provide the first examination of copper, lead and zinc toxicity on E. chloroticus embryos and larvae. All developmental anomalies recorded were severe and likely to result in larval death. In the case of delayed or arrested development, development might resume when larvae return to uncontaminated water. For example, a recent study showed that sea urchin larvae (Strongylocentrotus nudus from Korea) displaying arrested development after exposure to triclosan recovered if exposure was for less than 30 h (Hwang et al. Citation2014). Recovery from arrested development has not been documented with metal exposure. Nevertheless, even if recovery may occur, prolonging time in the plankton due to increased development time can have a range of adverse effects on larvae, such as increased predation risk, dispersal away from suitable habitat, and lower settlement success and juvenile growth rate (Pechenik Citation1999). In addition, because larval arm rods are necessary for swimming, vertical orientation and feeding (Pennington & Strathmann Citation1990), abnormal development of the skeleton is likely to have dramatic consequences for fitness (Dupont et al. Citation2008). Therefore, although toxic effects observed are sublethal, in the sense that they are categorised as developmental abnormalities, they are likely to result in eventual larval mortality, in many cases quite rapidly.
Single metals
The 72 h EC50s showed a decreasing order of toxicity for each metal as follows: Cu > Zn > Pb. Evechinus chloroticus appeared to be highly sensitive to copper. Overall, EC50s were well above local WQC for all three metals, while EC10s were below WQC for zinc and near WQC for copper (). Based on the probit model, metal concentrations at WQC levels may induce a low frequency of abnormal larvae for copper and lead (< 3%), and a moderate frequency for zinc (25%). Lead was not a concern for this species, with both EC50s and EC10s well above WQC.
Table 3. Summary of 10% effective concentration (EC10) and median effective concentration (EC50) values (μg/L) with 95% CI, and toxicity at water quality criteria (WQC) for 95% species protection levels (ANZECC Citation2000) derived from probit regression for each metal tested in a 72 h bioassay on Evechinus chloroticus.
Evechinus chloroticus appears more vulnerable to metals than other urchin species, with toxicity values towards the low end of the range reported for sea urchin embryos for all three metals (). E. chloroticus is the only species of its genus in the Echinometridae family, with the most closely related taxa thought to be in the Australian genus Heliocidaris. Toxicity values reported for Heliocidaris for the same metals are very similar to the present study and mostly towards the low end of the toxicity range, although the different toxicity endpoints make direct comparison between studies difficult.
Table 4. Comparative metal toxicity for the early life stages (embryos/larvae) of sea urchin species.
Many factors affect the toxicity of metal in aquatic organisms in addition to intrinsic variation between species; among them is the duration of the bioassays. Indeed, ecotoxicity studies on sea urchins typically use test durations ranging from 24 h to several days. This is because assays usually end when test animals reach the pluteus stage for echinoderms (ASTM Citation2012) and development time is regulated by temperature (Strathmann Citation1987). Longer assays may allow more time for metal absorption and result in generally lower EC50 values than shorter assays. Indeed, tropical species are often, but not always, less sensitive than their temperate counterparts (); similarly, the only toxicity value reported for an Antarctic sea urchin was the lowest reported so far (King & Riddle Citation2001). Therefore, the relatively higher sensitivity of E. chloroticus to metals observed in this study may in part be due to longer test duration because of slower development time than many, but not all, other species that have been tested to date. In addition, other factors affect the toxicity of metal in aquatic organisms; for example, ultraviolet radiation, salinity, pH, temperature and organic matter may all play an important role in the variation between species (McLusky et al. Citation1986; Pelletier et al. Citation2006; Eisler Citation2007; Green et al. Citation2007; Rozman et al. Citation2010).
All three metals adversely affect larval skeletal development in addition to various enzymatic processes, as illustrated by the predominance of abnormal arm development in E. chloroticus. These abnormalities are typical of metal effects on sea urchins (Bielmyer et al. Citation2005; Ghorani et al. Citation2013). All three metals induced marginally smaller sizes in normal E. chloroticus larvae, and copper and lead induced shorter arms compared to total body length. With lead, this effect was visible at lower concentration than those at which developmental abnormalities became apparent when looking exclusively at LOECs. This suggests that pluteus size is a better indicator of lead toxicity than development in E. chloroticus when tests are conducted with a single concentration (e.g. testing industrial effluents). Previous studies have showed a similar effect of lead on pluteus size (Paracentrotus lividus and Echinometra mathaei; Fernández & Beiras Citation2001; Ghorani et al. Citation2013). Lead competes with calcium for absorption in many organisms (Eisler Citation2007). It has recently been shown to lower whole body calcium accumulation in sea urchin larvae (Tellis et al. Citation2014), in which lead-induced calcium deficiency may impair skeletal rod and thus arm formation (Warnau & Pagano Citation1994; Ghorani et al. Citation2013).
Consequences of reduced arm or body length are harder to predict than abnormal development. Smaller larvae might be at greater risk of predation (Morgan Citation1995; Marshall & Keough Citation2008). Furthermore, plutei use their arms to feed and an increase in arm length leads to an increase in feeding rate (Strathmann Citation1971). Indeed food limitation studies on echinoderms showed that plutei respond to food limitation by elongating their arms to increase feeding rate (Strathmann et al. Citation1992; Fenaux et al. Citation1994; Sewell et al. Citation2004). Early stunting may therefore have a cascading effect, leading to lower growth rate throughout the larval stage.
Metal mixtures
All three metal mixtures tested produced a different interaction. However, none of these interactions were very strong and the EC50s of the mixtures were always similar to the EC50 of the most toxic metal of the pair. Concentration addition models suffer from the lack of a standardised way to account for experimental variability and to define a significant difference (Norwood et al. Citation2003; Manzo et al. Citation2010; Beyer et al. Citation2014). Hayes’ criterion proposes that additive indices differing from 1 by no more than a factor of 2 could not be distinguished from simple additivity (Rozman et al. Citation2010). In this study, the sum of TUs was always within the range 0.5–2; so, by these criteria, all mixtures in this study could be considered as strictly-additive.
Few studies have tested the effects of copper, lead and zinc binary mixtures on sea urchins (but see Fernández & Beiras Citation2001; Phillips et al. Citation2003; Kobayashi & Okamura Citation2005; Xu et al. Citation2011). Overall, metal mixtures seem to be mostly additive or have only a slight interactive effect (more- or less-than additive) on sea urchin larvae. Fernández & Beiras Citation2001 reported a more-than-additive effect of Cu + Pb on Paracentrotus lividus from Spain’s Atlantic coast, but warned that it should not be considered as significantly different from strict additivity based on Hayes’ criterion. Phillips et al. (Citation2003), working in California, found a less-than-additive effect of Cu + Zn on Strongylocentrotus purpuratus, but with a sum of TUs less than 2. Another study on Strongylocentrotus intermedius from the East China Sea concluded that there was a more-than-additive effect of Cu + Zn and Zn + Pb, and a less-than-additive effect of Cu + Pb. However, the authors did not take into account experimental variability and TU standard error for every mixture tested overlapped 1, so the mixtures were likely all strictly-additive (Xu et al. Citation2011). Finally, Kobayashi & Okamura (Citation2005) suggested that copper and lead enhanced zinc toxicity for Anthocidaris crassispina from Japan, but no formal additive indices were provided.
In other aquatic organisms, copper and zinc mixtures had a less-than-additive effect in approximately half of the 21 tests and a more-than additive effect in the other half, in Norwood et al.’s (Citation2003) review. The exact mechanisms of metal interaction in marine organisms are not known, but it has been hypothesised that copper may enhance zinc absorption (Flemming & Trevors Citation1989; Stauber & Florence Citation1990). Effects of the pair Pb + Zn were evenly distributed between the three interactions (less-than-additive, strictly-additive and more-than-additive) but this metal pair was rarely studied with only five assays reviewed by Norwood et al. (Citation2003). Copper and lead mixtures were also rarely studied, but these were strictly additive for larval development of the sea urchin Strongylocentrotus intermedius (Xu et al. Citation2011), and more-than-additive for chironomid larvae (Eisler Citation2007) and Parocentrotus lividus when in tertiary mixture with cadmium (Manzo et al. Citation2010).
Evechinus chloroticus larvae are extremely sensitive to copper and zinc, but relatively robust to exposure to lead. For many marine invertebrates, survival and quality of larvae and new recruits is a critical factor limiting adult population size (Caley et al. Citation1996; Pechenik et al. Citation1998; Crean et al. Citation2011). Concerning metal mixtures, the results are consistent with previous studies on metal toxicity in other sea urchins, even those distantly related. These results support the use of criteria based on strict-additivity for these metals to determine whether their concentrations in coastal waters exceed water quality criteria when in a simple mixture such as is currently recommended in New Zealand and Australia (ANZECC Citation2000).
Acknowledgements
We want to acknowledge Peter Hutchinson from E.N. Hutchinson Ltd. for generously providing us with commercial feed. Thanks to John Van der Sman, Sonja Miller, Paul Mensink and Cesar Cardenas Alacorn for help with specimen collections. Associate Editor: Dr Richard Taylor.
Disclosure statement
No potential conflict of interest was reported by the authors.
Additional information
Funding
References
- Anderson BS, Hunt JW, McNulty HR, Stephenson MD, Palmer FH, Denton DL, Reeve M. 1994. Refinement of effluent toxicity testing protocols for four marine species. 94-2WQ. Sacramento (CA): State Water Resources Control Board, California Environmental Protection Agency.
- Andrew NL. 1988. Ecological aspects of the common sea urchin, Evechinus chloroticus, in northern New Zealand: a review. N Z J Mar Freshw Res. 22:415–426. doi: 10.1080/00288330.1988.9516313
- ANZECC. 2000. Australian and New Zealand guidelines for fresh and marine water quality. Volume 1, The guidelines [Internet]. Auckland, New Zealand: Australian and New Zealand Environment and Conservation Council; [cited 2013 Jan 31]. Available from: http://www.environment.gov.au/water/publications/quality/pubs/nwqms-guidelines-4-vol1.pdf
- ASTM. 2012. Guide for conducting static acute toxicity tests with Echinoid embryos [Internet]. West Conshohocken (PA): ASTM International; [cited 2014 Aug 27]. Available from: http://www.astm.org/Standards/E1563.htm
- Barker MF. 2013. Chapter 24: Evechinus chloroticus. In: Lawrence JM, editor. Edible sea urchins: biology and ecology. Amsterdam: Elsevier; p. 355–368.
- Beyer J, Petersen K, Song Y, Ruus A, Grung M, Bakke T, Tollefsen KE. 2014. Environmental risk assessment of combined effects in aquatic ecotoxicology: a discussion paper. Mar Environ Res. 96:81–91. doi: 10.1016/j.marenvres.2013.10.008
- Bielmyer GK, Brix KV, Capo TR, Grosell M. 2005. The effects of metals on embryo-larval and adult life stages of the sea urchin, Diadema antillarum. Aquat Toxicol. 74:254–263. doi: 10.1016/j.aquatox.2005.05.016
- Caley MJ, Carr MH, Hixon MA, Hughes TP, Jones GP, Menge BA. 1996. Recruitment and the local dynamics of open marine populations. Annu Rev Ecol Syst. 27:477–500. doi: 10.1146/annurev.ecolsys.27.1.477
- Conroy PT, Hunt JW, Anderson BS. 1996. Validation of a short-term toxicity test endpoint by comparison with longer-term effects on larval red abalone Haliotis rufescens. Environ Toxicol Chem. 15:1245–1250. doi: 10.1002/etc.5620150733
- Crean AJ, Monro K, Marshall DJ. 2011. Fitness consequences of larval traits persist across the metamorphic boundary. Evolution. 65:3079–3089. doi: 10.1111/j.1558-5646.2011.01372.x
- Dix TG. 1969. Larval life span of the echinoid Evechinus chloroticus (val.). N Z J Mar Freshw Res. 3:13–16. doi: 10.1080/00288330.1969.9515273
- Dix TG. 1970. Biology of Evechinus chloroticus (Echinoidea: Echinometridae) from different localities. Part III: reproduction. N Z J Mar Freshw Res. 4:385–405. doi: 10.1080/00288330.1970.9515355
- Doyle CJ, Pablo F, Lim RP, Hyne RV. 2003. Assessment of metal toxicity in sediment pore water from lake Macquarie, Australia. Arch Environ Contam Toxicol. 44:0343–0350. doi: 10.1007/s00244-002-2003-8
- Dupont S, Havenhand J, Thorndyke W, Peck L, Thorndyke M. 2008. Near-future level of CO2-driven ocean acidification radically affects larval survival and development in the brittlestar Ophiothrix fragilis. Mar Ecol Prog Ser. 373:285–294. doi: 10.3354/meps07800
- Eisler R. 2007. Eisler’s encyclopedia of environmentally hazardous priority chemicals. Amsterdam: Elsevier.
- Engle V. 2012. National coastal condition reports IV [Internet]. Washington (DC): Office of Research and Development/Office of Water, Environmental Protection Agency; [cited 2015 May 11]. Available from: http://water.epa.gov/type/oceb/assessmonitor/nccr/index.cfm
- Fatoki OS, Mathabatha S. 2001. An assessment of heavy metal pollution in the East London and Port Elizabeth harbours. Water Sa. 27:233–240. doi: 10.4314/wsa.v27i4.4959
- Faust M, Altenburger R, Backhaus T, Blanck H, Boedeker W, Gramatica P, Hamer V, Scholze M, Vighi M, Grimme LH. 2001. Predicting the joint algal toxicity of multi-component s-triazine mixtures at low-effect concentrations of individual toxicants. Aquat Toxicol. 56:13–32. doi: 10.1016/S0166-445X(01)00187-4
- Fenaux L, Strathmann MF, Strathmann RR. 1994. Five tests of food-limited growth of larvae in coastal waters by comparisons of rates of development and form of echinoplutei. Limnol Oceanogr. 39:84–98. doi: 10.4319/lo.1994.39.1.0084
- Fernández N, Beiras R. 2001. Combined toxicity of dissolved mercury with copper, lead and cadmium on embryogenesis and early larval growth of the Paracentrotus lividus sea-urchin. Ecotoxicology. 10:263–271. doi: 10.1023/A:1016703116830
- Fitzpatrick JL, Nadella S, Bucking C, Balshine S, Wood CM. 2008. The relative sensitivity of sperm, eggs and embryos to copper in the blue mussel (Mytilus trossulus). Comp Biochem Physiol Part C Toxicol Pharmacol. 147:441–449. doi: 10.1016/j.cbpc.2008.01.012
- Flemming CA, Trevors JT. 1989. Copper toxicity and chemistry in the environment: a review. Water Air Soil Pollut. 44:143–158. doi: 10.1007/BF00228784
- Franco JL, Trivella DBB, Trevisan R, Dinslaken DF, Marques MRF, Bainy ACD, Dafre AL. 2006. Antioxidant status and stress proteins in the gills of the brown mussel Perna perna exposed to zinc. Chem Biol Interact. 160:232–240. doi: 10.1016/j.cbi.2006.02.002
- Freeman DJ, Marshall BA, Ahyong ST, Wing SR, Hitchmough RA. 2010. Conservation status of New Zealand marine invertebrates, 2009. New Zeal J Mar Fresh 44:129–148. doi: 10.1080/00288330.2010.495373
- Fukunaga A, Anderson MJ, Webster-Brown JG. 2011. Assessing the nature of the combined effects of copper and zinc on estuarine infaunal communities. Environ Pollut. 159:116–124. doi: 10.1016/j.envpol.2010.09.012
- Fukunaga A, Anderson MJ, Webster-Brown JG, Ford RB. 2010. Individual and combined effects of heavy metals on estuarine infaunal communities. Mar Ecol - Prog Ser. 402:123–136. doi: 10.3354/meps08457
- Gadd J, Cameron M. 2012. Antifouling biocides in marinas: measurement of copper concentrations and comparison to model predictions for eight Auckland sites. Auckland Council Technical Report TR2012/033. Auckland, New Zealand: Auckland Council.
- Ghorani V, Shahri NM, Ghassemzadeh F, Mortazavi MS. 2013. The effect of lead toxicity on embryonic development and early larval growth of the Echinometra mathaei sea urchin (Persian Gulf), morphologic and morphometric studies. Indian J Geo-Mar Sci. 42:29–34.
- Gillard GB, Garama DJ, Brown CM. 2014. The transcriptome of the NZ endemic sea urchin kina (Evechinus chloroticus). BMC Genomics. 15:1–27. doi: 10.1186/1471-2164-15-45
- Gorski J, Nugegoda D. 2006. Sublethal toxicity of trace metals to larvae of the blacklip abalone, Haliotis rubra. Environ Toxicol Chem. 25:1360–1367. doi: 10.1897/05-060R.1
- Govindasamy C, Viji Roy AG, Jayapaul A. 1998. Seasonal variation of heavy metals in water and zooplankton of Pondicherry coast, Bay of Bengal. Int J Ecol Environ Sci. 24:141–146.
- Green A, Chapman P, Allen H, Campbell P, Cardwell R, De Schamphelaere K, Delbeke K, Mount D, Stubblefield W. 2007. Aquatic toxicity for hazard identification of metals and inorganic metal substances. In: Adams WJ, Chapman PM, editors. Assessing the hazard of metals and inorganic metal substances in aquatic and terrestrial systems. Pensacola Beach (FL): SETAC Press; p. 89–112.
- Grosell M, Blanchard J, Brix KV, Gerdes R. 2007. Physiology is pivotal for interactions between salinity and acute copper toxicity to fish and invertebrates. Aquat Toxicol. 84:162–172. doi: 10.1016/j.aquatox.2007.03.026
- Grosell M, Nielsen C, Bianchini A. 2002. Sodium turnover rate determines sensitivity to acute copper and silver exposure in freshwater animals. Comp Biochem Physiol Part C Toxicol Pharmacol. 133:287–303. doi: 10.1016/S1532-0456(02)00085-6
- Hothorn T, Bretz F, Westfall P. 2008. Simultaneous inference in general parametric models. Biometrical J. 50:346–363. doi: 10.1002/bimj.200810425
- Hwang J, Suh S-S, Chang M, Yun Park S, Kwon Ryu T, Lee S, Lee T-K. 2014. Effects of triclosan on reproductive prarmeters and embryonic development of sea urchin, Strongylocentrotus nudus. Ecotoxicol Environ Saf. 100:148–152. doi: 10.1016/j.ecoenv.2013.10.029
- King CK, Gale SA, Hyne RV, Stauber JL, Simpson SL, Hickey CW. 2006. Sensitivities of Australian and New Zealand amphipods to copper and zinc in waters and metal-spiked sediments. Chemosphere. 63:1466–1476. doi: 10.1016/j.chemosphere.2005.09.020
- King CK, Riddle MJ. 2001. Effects of metal contaminants on the development of the common Antarctic sea urchin Sterechinus neumayeri and comparisons of sensitivity with tropical and temperate echinoids. Mar Ecol Prog Ser. 215:143–154. doi: 10.3354/meps215143
- Kobayashi N. 1980. Comparative sensitivity of various developmental stages of sea urchins to some chemicals. Mar Biol. 58:163–171. doi: 10.1007/BF00391872
- Kobayashi N, Okamura H. 2004. Effects of heavy metals on sea urchin embryo development. 1. Tracing the cause by the effects. Chemosphere. 55:1403–1412. doi: 10.1016/j.chemosphere.2003.11.052
- Kobayashi N, Okamura H. 2005. Effects of heavy metals on sea urchin embryo development. Part 2. Interactive toxic effects of heavy metals in synthetic mine effluents. Chemosphere. 61:1198–1203. doi: 10.1016/j.chemosphere.2005.02.071
- Lamare MD, Barker MF. 1999. In situ estimates of larval development and mortality in the New Zealand sea urchin Evechinus chloroticus (Echinodermata: Echinoidea). Mar Ecol Prog Ser. 180:197–211. doi: 10.3354/meps180197
- Lamare MD, Barker MF. 2001. Settlement and recruitment of the New Zealand sea urchin Evechinus chloroticus. Mar Ecol Prog Ser. 218:153–166. doi: 10.3354/meps218153
- Lorenzo JI, Nieto O, Beiras R. 2002. Effect of humic acids on speciation and toxicity of copper to Paracentrotus lividus larvae in seawater. Aquat Toxicol. 58:27–41. doi: 10.1016/S0166-445X(01)00219-3
- MacDiarmid A, editor. 2007. Treasures of the sea: Ngā taonga a tangaroa. A summary of biodiversity in the New Zealand Marine Ecoregion. Wellington: WWF-New Zealand.
- Mann RM, Hyne RV, Spadaro DA, Simpson SL. 2009. Development and application of a rapid amphipod reproduction test for sediment quality assessment. Environ Toxicol Chem. 28:1244–1254. doi: 10.1897/08-346.1
- Manzo S. 2004. Sea urchin embryotoxicity test: proposal for a simplified bioassay. Ecotoxicol Environ Saf. 57:123–128. doi: 10.1016/j.ecoenv.2003.10.007
- Manzo S, Buono S, Cremisini C. 2008. Predictability of copper, irgarol, and diuron combined effects on sea urchin Paracentrotus lividus. Arch Environ Contam Toxicol. 54:57–68. doi: 10.1007/s00244-007-9009-1
- Manzo S, Buono S, Cremisini C. 2010. Cadmium, lead and their mixtures with copper: Paracentrotus lividus embryotoxicity assessment, prediction, and offspring quality evaluation. Ecotoxicology. 19:1209–1223. doi: 10.1007/s10646-010-0506-z
- Marshall DJ, Keough MJ. 2008. The evolutionary ecology of offspring size in marine invertebrates. In: Sims DW, editor. Advances in marine biology. Vol. 53. San Diego (CA): Elsevier Academic Press Inc; p. 1–60.
- McCready S, Birch GF, Long ER, Spyrakis G, Greely CR. 2006. Relationships between toxicity and concentrations of chemical contaminants in sediments from Sydney Harbour, Australia, and vicinity. Environ Monit Assess. 120:187–220. doi: 10.1007/s10661-005-9057-9
- McLusky D, Bryant V, Campbell R. 1986. The effects of temperature and salinity on the toxicity of heavy-metals. Oceanogr Mar Biol. 24:481–520.
- Miller SL, Abraham ER. 2011. Characterisation of New Zealand kina fisheries. Wellington: Ministry of Fisheries.
- Milne JR. 2006. Contaminants in shellfish flesh. An investigation into microbiological and trace metal contaminants in shellfish from selected locations in the Wellington region. Wellington: Environmental Monitoring and Investigations Department, Greater Wellington Regional Council.
- Milne JR, Watts L. 2008. Stormwater contaminants in urban streams in the Wellington region. Wellington: Environmental Monitoring and Investigations Department, Greater Wellington Regional Council.
- Morgan SG. 1995. Life and death in the plankton: larval mortality and adaptation. New York (NY): CRC Press.
- Morrison MA, Lowe ML, Parsons DM, Usmar NR, McLeod IM. 2009. A review of land-based effects on coastal fisheries and supporting biodiversity in New Zealand. Wellington, New Zealand: Ministry of Fisheries.
- Nadella SR, Tellis M, Diamond R, Smith S, Bianchini A, Wood CM. 2013. Toxicity of lead and zinc to developing mussel and sea urchin embryos: critical tissue residues and effects of dissolved organic matter and salinity. Comp Biochem Physiol Part C Toxicol Pharmacol. 158:72–83. doi: 10.1016/j.cbpc.2013.04.004
- Neale MW. 2009. State of the Environment Monitoring: River Water Quality Annual Report 2007. Auckland, New Zealand: Auckland Regional Council.
- Newman M. 2012. Quantitative ecotoxicology, 2nd ed. London: CRC Press.
- Norwood WP, Borgmann U, Dixon DG, Wallace A. 2003. Effects of metal mixtures on aquatic biota: a review of observations and methods. Hum Ecol Risk Assess. 9:795–811. doi: 10.1080/713610010
- Novelli AA, Losso C, Ghetti PF, Ghirardini AV. 2003. Toxicity of heavy metals using sperm cell and embryo toxicity bioassays with Paracentrotus lividus (Echinodermata: Echinoidea): comparisons with exposure concentrations in the Lagoon of Venice, Italy. Environ Toxicol Chem. 22:1295–1301. doi: 10.1002/etc.5620220616
- Osman AGM. 2010. Water quality and heavy metal monitoring in water, sediments, and tissues of the African catfish Clarias gariepinus (Burchell, 1822) from the River Nile, Egypt. J Environ Prot. 01:389–400. doi: 10.4236/jep.2010.14045
- Pechenik JA. 1999. On the advantages and disadvantages of larval stages in benthic marine invertebrate life cycles. Mar Ecol Prog Ser. 177:269–297. doi: 10.3354/meps177269
- Pechenik JA, Wendt DE, Jarrett JN. 1998. Metamorphosis is not a new beginning. Bioscience. 48:901–910. doi: 10.2307/1313294
- Pelletier É, Sargian P, Payet J, Demers S. 2006. Ecotoxicological effects of combined UVB and organic contaminants in coastal waters: a review. Photochem Photobiol. 82:981–993. doi: 10.1562/2005-09-18-RA-688.1
- Pennington JT, Strathmann RR. 1990. Consequences of the calcite skeletons of planktonic echinoderm larvae for orientation, swimming, and shape. Biol Bull. 179:121–133. doi: 10.2307/1541746
- Phillips BM, Anderson BS, Hunt JW. 1998. Spatial and temporal variation in results of purple urchin (Strongylocentrotus purpuratus) toxicity tests with zinc. Environ Toxicol Chem. 17:453–459. doi: 10.1002/etc.5620170316
- Phillips BM, Nicely PA, Hunt JW, Anderson BS, Tjeerdema RS, Palmer SE, Palmer FH, Puckett HM. 2003. Toxicity of cadmium-copper-nickel-zinc mixtures to larval purple sea urchins (Strongylocentrotus purpuratus). Bull Environ Contam Toxicol. 70:0592–0599. doi: 10.1007/s00128-003-0026-0
- Raimondo S, McKenney CL. 2005. Projected population-level effects of thiobencarb exposure on the mysid, Americamysis bahia, and extinction probability in a concentration-decay exposure system. Environ Toxicol Chem. 24:564–572. doi: 10.1897/04-187R.1
- Ramachandran S, Patel TR, Colbo MH. 1997. Effect of copper and cadmium on three Malaysian tropical estuarine invertebrate larvae. Ecotoxicol Environ Saf. 36:183–188. doi: 10.1006/eesa.1996.1508
- Roose P, Albaiges J, Bebianno MJ, Camphuysen C, Cronin M, de Leeuw J, Gabrielsen G, Hutchinson T, Hylland K, Jansson B, et al. 2011. Chemical Pollution in Europe’s Seas: programmes, practices and priorities for research. Marine Board Position Paper 16. Ostend, Belgium: Marine Board-ESF.
- Rozman KK, Doull J, Hayes WJ. 2010. Dose and time determining, and other factors, influencing toxicity. In: Hayes WJ, editor. Handbook of pesticide toxicology. Vol. 1. Amsterdam: Academic Press; p. 3–103.
- Rumbold DG, Snedaker SC. 1997. Evaluation of bioassays to monitor surface microlayer toxicity in tropical marine waters. Arch Environ Contam Toxicol. 32:135–140. doi: 10.1007/s002449900165
- Schiel DR. 1990. Macroalgal assemblages in New Zealand: structure, interactions and demography. Hydrobiologia. 192:59–76. doi: 10.1007/BF00006227
- Sewell MA, Cameron MJ, McArdle BH. 2004. Developmental plasticity in larval development in the echinometrid sea urchin Evechinus chloroticus with varying food ration. J Exp Mar Biol Ecol. 309:219–237. doi: 10.1016/j.jembe.2004.03.016
- SFEI. 2015. Contaminant data display and download. San Francisco Estuary Institute, Regional Monitoring Program. [Internet]. [cited 2015 Apr 15]. Available from: http://sfei.org/programs/rmp-data
- Shears NT, Babcock RC. 2003. Continuing trophic cascade effects after 25 years of no-take marine reserve protection. Mar Ecol Prog Ser. 246:1–16. doi: 10.3354/meps246001
- Shears NT, Babcock RC. 2007. Quantitative description of mainland New Zealand’s shallow subtidal reef communities. Science for Conservation 280. Wellington: Department of Conservation.
- Sprague JB. 1970. Measurement of pollutant toxicity to fish. II. Utilizing and applying bioassay results. Water Res. 4:3–32. doi: 10.1016/0043-1354(70)90018-7
- Stauber JL, Florence TM. 1990. Mechanism of toxicity of zinc to the marine diatom Nitzschia closterium. Mar Biol. 105:519–524. doi: 10.1007/BF01316323
- Strathmann MF. 1987. Reproduction and development of marine invertebrates of the northern Pacific Coast. Seattle (WA): University of Washington Press.
- Strathmann RR. 1971. The feeding behavior of planktotrophic echinoderm larvae: mechanisms, regulation, and rates of suspension feeding. J Exp Mar Biol Ecol. 6:109–160. doi: 10.1016/0022-0981(71)90054-2
- Strathmann RR, Fenaux L, Strathmann MF. 1992. Heterochronic developmental plasticity in larval sea urchins and its implications for evolution of nonfeeding larvae. Evolution. 46:972–986. doi: 10.1111/j.1558-5646.1992.tb00613.x
- Tellis MS, Lauer MM, Nadella S, Bianchini A, Wood CM. 2014. Sublethal mechanisms of Pb and Zn toxicity to the purple sea urchin (Strongylocentrotus purpuratus) during early development. Aquat Toxicol. 146:220–229. doi: 10.1016/j.aquatox.2013.11.004
- US EPA. 2002. Short-term methods for estimating the chronic toxicity of effluents and receiving waters to marine and estuarine organisms. Washington (DC): US Environmental Protection Agency, Office of Water.
- US EPA. 2007. Updated Aquatic Life Copper Criteria [Internet]. Washington (DC): U.S. Environmental Protection Agency, Office of Water; [cited 2014 Aug 27]. Available from: http://water.epa.gov/scitech/swguidance/standards/criteria/aqlife/copper/2003_index.cfm
- US EPA. 2014. National Recommended Water Quality Criteria [Internet]. [cited 2013 Jan 31]. Available from: http://water.epa.gov/scitech/swguidance/standards/criteria/current/index.cfm#cmc
- Van Genderen E, Adams W, Dwyer R, Garman E, Gorsuch J. 2015. Modeling and interpreting biological effects of mixtures in the environment: Introduction to the metal mixture modeling evaluation project. Environ Toxicol Chemi. 34:721–725. doi: 10.1002/etc.2750
- Villouta E, Chadderton WL, Pugsley CW, Hay CH. 2001. Effects of sea urchin (Evechinus Chloroticus) grazing in Dusky Sound, Fiordland, New Zealand. N Z J Mar Freshw Res. 35:1007–1024. doi: 10.1080/00288330.2001.9517060
- Walker M. 1984. Larval life-span, larval settlement, and early growth of Evechinus chloroticus (Valenciennes). N Z J Mar Freshw Res. 18:393–397. doi: 10.1080/00288330.1984.9516060
- Warnau M, Pagano G. 1994. Developmental toxicity of PbCl2 in the echinoid Paracentrotus lividus (Echinodermata). Bull Environ Contam Toxicol. 53:434–441. doi: 10.1007/BF00197237
- Warne MSJ. 2003. A review of the ecotoxicity of mixtures, approach to, and recommendations for, their management. In: Langley A, Gilbey M, Kennedy B, editors. Health environmental assessment of site contamination. Adelaide: National Environment Protection Council; p. 253–277.
- Xu X, Li Y, Wang Y, Wang Y. 2011. Assessment of toxic interactions of heavy metals in multi-component mixtures using sea urchin embryo-larval bioassay. Toxicol In Vitro. 25:294–300. doi: 10.1016/j.tiv.2010.09.007
- Zizza M, Giusi G, Crudo M, Canonaco M, Facciolo RM. 2013. Lead-induced neurodegenerative events and abnormal behaviors occur via ORXRergic/GABAARergic mechanisms in a marine teleost. Aquat Toxicol. 126:231–241. doi: 10.1016/j.aquatox.2012.11.011