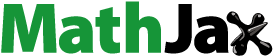
ABSTRACT
High-entropy alloys (HEAs) have attracted a great deal of interest over the last 14 years. One reason for this level of interest is related to these alloys breaking the alloying principles that have been applied for many centuries. Thus, HEAs usually possess a single phase (contrary to expectations according to the composition of the alloy) and exhibit a high level of performance in different properties related to many developing areas in industry. Despite this significant interest, most HEAs have been developed via ingot metallurgy. More recently, powder metallurgy (PM) has appeared as an interesting alternative for further developing this family of alloys to possibly widen the field of nanostructures in HEAs and improve some capabilities of these alloys. In this paper, PM methods applied to HEAs are reviewed, and some possible ways to develop the use of powders as raw materials are introduced.
Nomenclature | ||
HEAs | = | high-entropy alloys |
PM | = | powder metallurgy |
PMHEA | = | HEAs manufactured by PM |
FCC | = | face-centred cubic |
BCC | = | body-centred cubic |
HCA | = | hierarchical cluster analysis |
VEC | = | valence electron concentration |
MA | = | mechanical alloying |
HEAMA | = | HEA powders made by mechanical alloying |
PCA | = | process control agent |
SPS | = | spark plasma sintering |
HP | = | hot pressing |
CIP | = | cold isostatic pressing |
HIP | = | hot isostatic pressing |
FAHP | = | field-assisted hot pressing |
LMD | = | laser melting deposition |
DMD | = | direct melting deposition |
DLF | = | direct laser fabrication |
SLM | = | selective laser melting |
SEBM | = | selective electron beam melting |
LPBF | = | laser powder bed fusion |
SAED | = | selected-area electron diffraction |
EDS | = | energy dispersive X-ray spectroscopy |
TEM | = | transmission electron microscopy |
SFE | = | stacking fault energy |
ODS | = | oxide-dispersion-strengthened (alloys) |
HFIHS | = | high-frequency induction-heated sintering |
PPS | = | pulse plasma sintering |
XRD | = | X-ray diffraction |
HRB | = | Brinell hardness |
DSC | = | differential scanning calorimetry |
DTA | = | differential thermal analysis |
CCT | = | continuous cooling temperature diagram |
EBSD | = | electron back-scattered diffraction |
HEAMC | = | HEA matrix composite |
TWIP | = | twinning-induced plasticity steel |
HEAMCs | = | metal matrix composites based on HEAs |
MMCs | = | metal matrix composites |
ID Layer | = | inter-diffusion layer |
HEAODS | = | oxide dispersion strengthened high-entropy alloys |
1. Introduction
For over 7000 years, since the first copper-based alloy was cast [Citation1], metallurgy has been based on the development of alloys where one metal, the base alloy, was used as the host for other metals (alloying elements) to improve possible weaknesses of the base metal. Most metals are ductile materials, so the purpose of alloying elements is to improve, primarily, hardness and strength. As society became more sophisticated, it began to demand more of metallic materials, and other requirements were introduced such as resistance to corrosion, wear, high temperature, fatigue, impact, etc. These requirements forced physical metallurgists to develop new classes of materials and new heat treatments. The paradigm of a ‘base metal’ to construct an alloy started to change in the 1970s, when a new class of materials was developed industrially: the intermetallics. Intermetallics play an important role as secondary components after heat treatments, due to their defined stoichiometry providing high hardness. Thus, the search for materials with improved strength at high temperatures and low densities opened an opportunity for some intermetallics such as γ-TiAl, with a stable face-centred cubic (FCC) structure at room temperature [Citation2]. However, these new families of alloys with no defined base element were restricted to a few families of intermetallics, where usually two different metals together formed the main component of the alloy. Except for the intermetallic approach and ‘superalloys’, wherein the amount of alloying elements could reach half of the composition, the use of alloying elements has always been restricted. This restriction is linked to the risk of forming uncontrolled intermediate compounds, which usually introduce brittleness in the material.
However, this method of working in physical metallurgy started to change in 2004 when Cantor et al. [Citation3] published a paper in which a multicomponent alloy, starting from five elements in equiatomic proportions (Fe20Cr20Mn20Ni20Co20), was produced, reaching an FCC monophasic structure. At the same time, Yeh et al. [Citation4] developed the same concept, defining ‘high-entropy alloys’ (HEAs) as those composed of five or more principal elements in equimolar ratios. According to Yeh et al., to extend the scope of the alloy design, HEAs may contain principal elements within an atomic concentration range between 35 and 5% for each element. In this paper, Yeh et al. explained that the reason why we can attain a single-phase solid solution with such a high amount of alloying elements is fully linked to controlling the configurational entropy of the system. In conventional metallurgy, there is a main element and the alloying elements are added in a small percentage. In HEA the alloy is composed by five or more alloying elements in similar atomic percentage. This is what we want to symbolise in , where the size of the circles represents the contribution (in at.-%) of the different alloying elements in the final alloy.
Fourteen years have passed since these foundational papers. Today, HEAs have become a promising research field, with more than 5000 scientific papers published, according to the most extensive databases. Among those papers, we want to highlight some interesting reviews. Perhaps the most comprehensive review is the one by Miracle and Senkov [Citation5] who established a full state of art regarding this topic. Their paper covers principles, fundamentals, families of alloys, microstructural discussions, properties (including a comparison with those of possible competing alloys), etc. This paper is critical for researchers who want an introduction to this field. Other general and critical reviews about the topic can be found in [Citation6–10]. In addition, HEAs have been proven to be capable of demonstrating a wide variety of properties, so many works have collected arguments focusing on their value in different fields. References [Citation11,Citation12] highlight the importance of HEAs in applications related to physical properties such as magnetic, electrical or thermal properties. HEAs can also be used under extremely corrosive conditions [Citation13–15]. Moreover, HEAs are clearly a promising material for their introduction in the market due to their good mechanical performance [Citation16–18] including at high [Citation5] or cryogenic temperatures [Citation19]. Other reviews on the mechanical behaviour of HEAs deal with their fundamental deformation behaviour [Citation20] or fracture resistance [Citation21]. Two books concerning this topic have also been written [Citation22,Citation23].
Most of the works related to HEAs have developed processing methods based on ingot metallurgy. Arc melting has been confirmed to be a highly efficient technique when more than five metals, some of them with high melting points, must be melted and solidified with a good level of solubility while avoiding segregation. However, in cases where a complex composition must be achieved, ingot metallurgy presents some difficulties that can diminish its potential for fabricating an emerging, promising family of alloys. In this context, powder metallurgy (PM) has shown high potential as a way to manufacture HEAs. PM, a forming technology that allows significant compositional accuracy can completely avoid segregation, achieve superior microstructural control (including the formation of nanocrystalline materials) and easily produce metal matrix composites.
Although the first works related to HEAs started to be published in 2004, some papers using PM started to appear a few years later [Citation24–26]. Since the early days of this new family of structural alloys, the benefits of nanostructures and their potential use for many applications have been highlighted [Citation4]. In this competitive environment, PM, similar to other forming techniques such as high-pressure torsion has many advantages [Citation27]. PM also has two other advantages over other forming techniques: (1) it can be applied when metals with dissimilar densities must be used, which is the case when lightweight HEAs are developed [Citation28]; and (2) it can be applied when many metals with extremely high melting points are involved in the development of the HEA, i.e. so-called refractory HEAs [Citation29].
For this review, a selective search was performed in four databases (ISI Web of Science, Scopus, Science Direct and Google Scholar). Different combinations of keywords (high-entropy alloys, powder metallurgy, sintering, mechanical alloying (MA), gas atomising, spark plasma sintering, among others) were used, and 166 papers that fulfil the requirements were found. Some papers were not included in the database of the review for some of the following reasons: a reliable citation system could not be reproduced, some steps in the experimental procedure were not clear, or PM was used as an alternative process to other production methods. As an example of this latter issue, many papers on ‘laser cladding’ were found, where powders were used to produce coatings or surface modifications on substrates using a laser power source. In these cases, papers were included only when the powder development had a special role. Most of the papers aimed to consolidate a bulk material (after a sintering process), and only a few were devoted to the development of the powder technology and thus were included for special importance.
The relevance of the topic can be seen in the increasing number of papers published in the last 10 years (see ). The interest in PMHEAs has produce a geometrical increase in the papers published during this period.
2. Powder metallurgy HEAs: chemical compositions
HEAs were first developed by ingot metallurgy using at least five core elements alloyed in equimolar concentrations. The literature offers hundreds of different compositions, based mainly on the 3d transition metals. Such complex compositions (five or more elements, all of them with an important role in the alloy) are needed to conform to a basic set of requirements established by Yeh et al. [Citation6] to be considered a HEA. These requirements are (1) thermodynamics: high-entropy effects; (2) kinetics: sluggish diffusion; (3) structures: severe lattice distortion; and (4) properties: cocktail effects. These four core effects in HEAs have been widely discussed in many papers but are highlighted in [Citation5,Citation18] and [Citation30].
Reviewing the technical literature regarding ingot casting HEAs, these alloys can be divided in four families: 3d transition metals, lightweight materials, lanthanide and refractory HEAs ().
In the present review, nearly two hundred papers considering 166 PM alloys are included. The number of papers is higher than the number of alloys because many authors use the same alloys. If the frequency with which the different alloying elements have been used is analyse the frequency with which the different alloying elements were used (), three main groups of elements arise, which are also represented in .
Figure 4. Distribution (frequency) of alloying elements in the studied PM HEAs (i.e. Fe is in 152 different alloys; Al in 98 of the studied alloys).
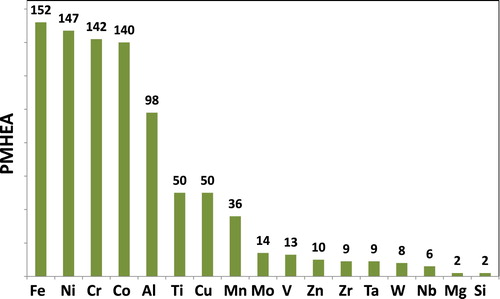
The most commonly used elements are Fe, Ni, Cr and Co, all of which can be considered in the core group of transition metals. A second group of alloying elements is used less frequently, also belonging to the transition metals (except Al): Al, Ti, Cu and Mn. If we take into account the definition of HEA introduced by Yeh et al. [Citation4] we would need at least five alloying elements. Considering the distribution shown in , most of the alloys studied are composed of the 4 elements of the first group, and one or two elements of the second group. We can also find a third interesting group of elements used with less frequency: Mo, V, Zn, Zr, Ta, W, and Nb. Except for Zn, these elements are all refractory metals and play the leading role in the so-called refractory HEAs [Citation29]. After the first booming of the HEAs mainly based on the 3d transition metals, later refractory HEAs were developed, possessing very specific and different properties that could cover another range of applications. Finally, there are some examples in which the use of elements such as Mg and Si is reported, so a fourth group can be seen in .
To determine whether some combinations of elements tend to be used consistently, we performed a hierarchical cluster analysis (HCA) by means of the statistical Jaccard distance [Citation31]. The clustering followed the complete linkage method based on dissimilarities between the different alloys’ compositions. We used 166 PM HEAs from different papers in the study.
The HCA identified nine different clusters of alloys based on their composition (). Some of the clusters had a few members, and others were composed of a considerably higher number of members (). As could be expected, the clusters with more members are related to the HEAs based on the 3d transition metals.
Table 1. Clusters compositions and some members in the cluster.
describes the main alloying elements that characterise each cluster and some comments providing more information about the group.
On the right side of the dendrogram () we can see a bundle of papers among clusters 1–3 and 4, and these latter groups are related to cluster 2. These clusters are the most powerful in terms of membership. Interestingly, the core group (clusters 1 and 3) are composed of four transition metals (Fe, Ni, Cr and Co), the most common in HEAs (), plus a fifth transition metal from the second most used elements (Al, Mn and Ti). Another interesting fact is that the core composition of cluster 3 is the so-called Cantor alloy [Citation3] one of the most studied HEAs in the field. If we analyse the alloys included in these clusters in some detail, most of them exhibit an FCC structure after consolidation or, in some cases, FCC+body-centred cubic (BCC). The main composition of cluster 1 is a clear example of how Al is a critical element that can appreciably modify the crystal structure. Just by increasing the Al content in the HEA, the final crystal structure can be moved from FCC to FCC+BCC and BCC [Citation6]. These four clusters are connected to cluster 2, which only has Ni and Co in common with all the other clusters, and some other elements depending on the cluster, i.e. Al and Ti but also Cu and Zn. Considering Ni, Co, Al and Ti, cluster 2 shares elements with all the other clusters on this side of the dendrogram. These combinations of elements also produce a final structure of FCC+BCC in all the members of the cluster.
On the left side of the dendrogram are three clusters with all the refractory elements, including Ti. All of these elements have a BCC structure, so all the HEAs produced from different combinations of these elements should produce a BCC structure. These are also transition metals, mostly belonging to periods 5 and 6 of the periodic table. These HEAs are called ‘refractory high-entropy alloys’ [Citation29,Citation178], and were developed in the search for high-temperature load-bearing structures and thermal protection materials for the aerospace industry, among other applications.
In the centre of the dendrogram are two clusters composed mainly of alloying elements from the 3d transition metals. Clusters 5 and 9 share Fe, Cr and Al and include Ti, Cu, Zn, Mn and V. These two clusters contain many elements with a preferential BCC structure, so these HEAs will preferably crystallise as BCC.
The formation of one single phase is a desired objective when producing HEAs. In a conventional alloy, where we could have one main component and some minor alloying elements, the prediction of possible solid solutions are governed by the Hume-Rothery rules. In HEAs, where we have a much more complex situation in terms of alloying elements, the enthalpy and entropy of mixing play the most relevant role. There are a couple of deep investigations [Citation179–181] that have studied these key parameters on the phase formation, and they have proposed different empirical rules: the formation of solid solutions or intermetallics depends on the enthalpy of mixing (ΔHmix), the entropy of mixing (ΔSmix) and the atomic size (δ). There are some specific conditions related to the limits of these parameters that promote the formation of simple phases or intermetallics. In the different regions are mapped according to these limits: ΔHmix cannot be too large in value because large positive ΔHmix leads to phase separation and large negative ΔHmix typically generates intermetallic phases. δ has to be small enough since large δ leads to excess strain energy and destabilises simple structures.
Figure 7. Superimposed effect of ΔHmix, δ (atomic size) and ΔSmix on phase stability in equiatomic multi-component alloys and bulk metallic glasses. The symbol ○ represents equiatomic amorphous phase forming alloys; ● represents non-equiatomic amorphous phase forming alloys; □ represents solid solution phases and △ represents intermetallic phases. The region delineated by the dash-dotted lines indicates the requirements for solid solution phases to form, from [Citation180].
![Figure 7. Superimposed effect of ΔHmix, δ (atomic size) and ΔSmix on phase stability in equiatomic multi-component alloys and bulk metallic glasses. The symbol ○ represents equiatomic amorphous phase forming alloys; ● represents non-equiatomic amorphous phase forming alloys; □ represents solid solution phases and △ represents intermetallic phases. The region delineated by the dash-dotted lines indicates the requirements for solid solution phases to form, from [Citation180].](/cms/asset/e96d5873-296b-4203-9c67-b09a933fb6d5/ypom_a_1584454_f0007_oc.jpg)
This equilibrium among solid solution, intermetallics and amorphous phases was explored with more detail also by Guo et al. [Citation182] and can be analysed in .
Figure 8. A δ (atomic size)-ΔHmix plot delineating the phase selection in high entropy alloys. The dash-dotted regions highlight the individual region to form solid solutions, intermetallic compounds and the amorphous phase. From [Citation182].
![Figure 8. A δ (atomic size)-ΔHmix plot delineating the phase selection in high entropy alloys. The dash-dotted regions highlight the individual region to form solid solutions, intermetallic compounds and the amorphous phase. From [Citation182].](/cms/asset/e9747fd4-7312-4397-9cac-bb4c02e67e36/ypom_a_1584454_f0008_oc.jpg)
Once the limits in terms of ΔHmix, ΔSmix and δ, have been stablished, the next interesting point to analyse is the nature of the possible single phase formed. In principle in HEAs it could be BCC, FCC or a mix of BCC and FCC. According to [Citation183] the most critical factor that can predict if the alloy crystallises into BCC or FCC is the valence electron concentration (VEC), which is calculated from the weighted average VEC of the constituent components. Guo et al. [Citation183] summarised the relationship between VEC and structure of many HEAs in .
Figure 9. Relationship between VEC and the FCC, BCC phase stability for various HEA systems.
Notes: Fully closed symbols for sole FCC phases; fully open symbols for sole BCC phase; top-half closed symbols for mixed FCC and BCC phases, from [Citation183].
![Figure 9. Relationship between VEC and the FCC, BCC phase stability for various HEA systems.Notes: Fully closed symbols for sole FCC phases; fully open symbols for sole BCC phase; top-half closed symbols for mixed FCC and BCC phases, from [Citation183].](/cms/asset/19abbb67-8f7d-4c85-a471-a7324c8ae58a/ypom_a_1584454_f0009_oc.jpg)
The VEC can also be used to predict the sigma phase formation in Cr and V containing HEAs [Citation184]. These alloys with so high amount of Cr or V, can easily produce sigma phase that may affect strongly the properties.
Considering the predictions of phase formation that can be achieved from the VEC displayed in and the obtained phases in PMHEAs (), two different facts should be taken into account: in the works used to obtain most of alloys are included in a few of the clusters compositions presents in this work (mainly C1, C5 and C8) and PMHEAs considered to obtain the clusters compositions, were not heat treated to homogenise their final stages, being the measured phases obtained after sintering.
Summarising what we can extract form the statistical analysis of the studied PMHEAs samples, there are five connected clusters, based mainly on Fe, Ni, Cr and Co, to which the majority of the studied alloys belong. These connected clusters preferably produce FCC HEAs or a mix of FCC and BCC. Three clusters contain refractory HEAs, crystallising as BCC and opening new opportunities in the field of high-temperature applications. Finally, two small clusters are composed of transition metals but with a combination of alloying elements that promote BCC structures. Using PM, we can form any HEA that can be produced via ingot metallurgy and open new possibilities for alloying with very competitive performance, as we will see later.
3. Powder development
The first step in any PM process is to obtain suitable powders for the process. In this special family of alloys where at least five different metals contribute to the alloy, any PM route should be easier by using fully prealloyed powders as the starting material. However, we have found many papers in which the starting point is a conventional mix of powders, using pure metals in powder form [Citation33,Citation49,Citation52,Citation69,Citation80,Citation83,Citation157,Citation171,Citation172,Citation185, Citation186]. In those works, the powders were submitted to different shaping methods such as uniaxial pressing, cold isostatic pressing or spark plasma sintering.
In the different works where fully prealloyed powders were used, two different methods were selected to obtain the powders: MA and atomising. The most common method to obtain HEA powders is MA. This method makes any possible composition feasible with enough time and energy to combine the optimal powder with the optimal composition. In Table S1 of the accompanying supplementary data, Appendix A shows the different conditions used to obtain different fully prealloyed powders by MA, as well as the phase obtained when the process is completed. The main records of this table are presented in and . The main milling system used is a planetary ball mill, and the main grinding system is composed of stainless-steel vials and balls, although other combinations are suitable.
Figure 10. Mechanical alloying conditions. The length of the bars represent the number of times a certain procedure was used.
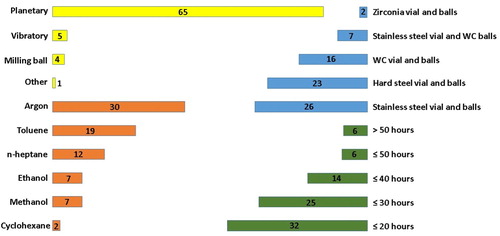
Figure 11. Frequency of the main phases associated to the clusters’ compositions, obtained after the mechanical alloying step: BCC, FCC and BCC with some minor FCC and FCC with some minor BCC.
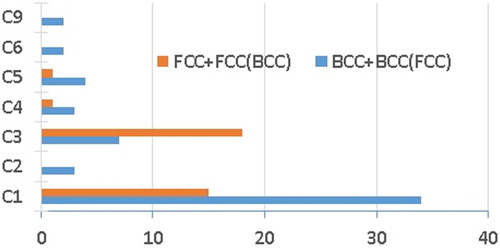
In all cases, a protective milling environment was used. Depending on the goal in terms of composition of the alloy, different authors have selected different ways to reach the optimum powder for the process. One interesting point is that in most works, a carbon-based process control agent (PCA) is used, but as is discussed in the next paragraph, only a few authors consider its possible contamination or influence in the final development of the HEA.
In all the analysed papers, a monophasic HEA (BCC or FCC, depending on the starting metal powder) is obtained (see ) after a prudential time for the process, i.e. on the order of hours (). In clusters C1 to C4, from the powder state and before any heat treatment, the departing phases are FCC and BCC, and in clusters C5 to C9, a BCC structure preferentially forms from the powder.
One weakness of MA to consider is the possible contamination of the powder from two different sources: the PCA and the grinding media. When the grinding media are stainless steel, and the target alloy contains Cr and/or Fe, the possible transfer of these elements may be controlled, in the same way that tungsten carbide is used to produce some refractory HEAs. Nevertheless, the effect of the PCA is not negligible and is not mentioned in most of the analysed papers. Only two papers consider the effect of the PCA [Citation144,Citation170], minimising this effect because of the possible formation of undesirable carbides, which are produced during the formation process by spark plasma sintering (SPS). In contrast, in other works, the presence of extra carbon is desirable because the final goal of the process is to develop a hardmetal from the HEA [Citation146,Citation173].
The second most commonly used method to obtain a full prealloyed HEA powder is atomising. This PM method is especially suitable for most additive manufacturing methods. In this review, different atomising methods are presented, all of them with successful results: gas atomising in Ar [Citation73,Citation107,Citation108,Citation121,Citation140,Citation147,Citation159,Citation187,Citation188], gas atomising in N2 [Citation59] or even water atomising.
An alternative to MA and atomising to produce fully prealloyed HEA powders was followed in [Citation61,Citation70]. In those works, alloys were obtained by melting, and the as-cast bulk part was then powdered via high-energy milling.
To conclude this chapter related to powder development, we must mention the work of Liu et al. [Citation154]. In their work, a hardmetal based on the HEA binder was pursued. Instead of using pure elemental powders as the starting powders for the alloying elements of the binder and then mixing these powders with the reinforcing carbide (this time TiC), the authors mixed WC, Mo2C, TaC, NbC and VC with pure Ti in a high-energy mill. The mixed powders were subsequently sintered at high temperature (1500°C) by SPS and considering the higher affinity of the Ti by the C and looking for the reduction of all the refractory carbides at the sintering temperature. At the end of the process, a matrix of BCC HEA with TiC as the reinforcing agent was obtained.
4. Conventional consolidation methods
Once the alloy has been prepared by mixing powders in some way to obtain a fully prealloyed powder, we have to follow a PM processing method to obtain the bulk materials. Omitting the additive manufacturing methods, which will be treated in another section, analyses the methods selected in the studied papers, including the percentage of works that use each technique. In this figure, we can see that MA is the preferred way to produce the fully prealloyed HEA powders and SPS is the preferred consolidation method. Therefore, the most common combination is MA+SPS.
In many papers, the route followed to obtain the bulk material is SPS [Citation189]. One of the main advantages of SPS is that the sintering temperature is reached rapidly, and the dwell time is usually short. Those conditions enable fully densifying the sintered material but avoiding excessive grain growth. Other methods that also combine pressure and temperature (such as other hot pressing (HP) methods or hot isostatic pressing (HIP) methods) can also attain full density, but these methods usually need higher temperatures and longer times, so grain growth is not fully avoided, and the mechanical properties can be affected.
Table S2 of the accompanying supplementary data, Appendix A, shows the SPS conditions used in most of the papers analysed and groups the conditions by the cluster composition. The temperature conditions in most of the materials in clusters C1–C4 and C5–C9, where most of the alloying elements are not refractory metals, remain near 1000°C, and that temperature could be considered a low sintering temperature for most of the core elements of these clusters (such as Fe, Ni, Co or Cr). Despite the scattered values regarding time, these values reach up to 60–80 min, but most of the studies maintain the time condition under 10 min, which can be considered a short sintering time. Thus, in most of the studied works, the potential use of SPS to reach full density while maintaining good microstructural features in terms of grain size is fully harnessed. A couple of papers [Citation48,Citation66], using these compositions, where the sintering temperature reaches up to over 1350°C (even 1700°C), have reported higher densities than most of the studied works. The final objective of these two articles was to develop a ‘cermet’ using the HEA as binder and WC as the hard phase. To attain full microstructural integration of the WC in the binder, the sintering conditions were scaled up until the degree of integration was higher than average in these composite clusters.
After SPS, the second most common processing route to obtain PM HEAs is the conventional ‘press and sintering’ route, which is the most extensively used route in mass production for conventional PM. Among the studied works, most use conventional uniaxial pressing, but in a few articles, cold isostatic pressing (CIP) has been used. In [Citation187] is used pressurless sintering. Table S3 of the accompanying supplementary data, Appendix A, shows the pressing and sintering conditions of these PMHEAs. In most of the cases, the compacting pressure remains at values easily handled by the PM industry (under 500 MPa). Other issues are the sintering conditions. Here, the sintering is not enhanced, so the material is fully densified via adequate temperature and time. Compared with the temperatures used in SPS, we find slightly higher average temperatures, especially in some works aiming to develop a cermet [Citation47,Citation68,Citation129,Citation157]. The protective atmosphere used is generally pure Ar and in some cases vacuum.
Two other significant minor processing methods are HP and HIP. These two cases involve enhanced sintering methods (simultaneously applying pressure and temperature), but usually, much longer times are required than those in SPS, despite the temperature remaining at low values. These longer times can produce significant grain growth despite the low temperatures. The HP and HIP conditions are detailed in Table S4 of the accompanying supplementary data, Appendix A. Only in one case [Citation164], where the HP method is a ‘field assisted hot pressing’ (FAHP) method, does the sintering time remain near those of SPS.
In [Citation45] Fu et al. studied and compared the same PM HEA (Al0.6NiFeCrCo, type C1) obtained by SPS (1000°C/30 MPa/8 min) and HP (1000°C/30 MPa/60 min). In both cases, a final FCC structure with some minor BCC is obtained, and the values for compression strength, strain to failure and hardness are quite similar (2150 MPa, 10.6% and 594 Hv vs. 2298 MPa, 10.8% and 570 Hv, respectively). The slightly smaller grain size in the SPS alloy, due to the shorter sintering time, results in a higher yield compression strength (1870 MPa in SPS vs. 1830 MPa in HP).
Beyond the ‘conventional’ PM methods described above, some interesting PM alternatives exist. The first alternative is the development of a FeCoCrNi (C1 type) PM HEA by powder extrusion [Citation108]. Powders are canned and degassed and then hot extruded at 1200°C/60 min. The obtained tensile mechanical properties are very competitive relative to those of the equivalent ‘as-cast’ alloy [Citation190] even if we compare the alloy with a TWIP steel [Citation191] (with much more complex processing and heat treatment). In we can compare these results. The second alternative to conventional PM methods is a method wherein a PM HEA (CoCrFeMnNi, C3 type) is shaped by shock wave compaction at a pressure between 1 and 3 GPa [Citation114]. This pressed alloy is further sintered in vacuum at 1100°C for 2 h. The alloy exhibits a pure FCC structure (with some ZrO2 contamination from the MA process) and full density, leading to promising tensile strength values, much higher than those of the same material processed by static compaction but far less than the same material processed by SPS [Citation139].
Table 2. Comparison of some tensile properties of HEAs made by ingot casting and PM and a TWIP steel.
A few materials under study are subjected to annealing after consolidation [Citation39,Citation58,Citation141], but the main objective of the heat treatment is to ensure the formation of the FCC structure after the consolidation process.
5. Processing by additive manufacturing
Additive manufacturing is a good opportunity to consolidate HEAs. Once we have powders that can be mixed in an equimolar manner or that are fully prealloyed by MA, and especially by gas atomising, this manufacturing method is a good way to produce parts with a very complex shape and specific properties. In Table S5 of the accompanying supplementary data, Appendix A, we summarise the main works found in the literature regarding manufacturing bulk parts by additive manufacturing (many articles related to ‘laser cladding’ have not been considered in this review). Most of the HEAs developed by this technology belong to clusters 1, 3 and 4; that is, HEAs based on the core group of transition metals that constitute the main group of alloys are usually developed by PM, especially cluster 3, based on the Cantor alloy [Citation3]. Only one work based on refractory HEAs was found [Citation168].
Under the topic ‘additive manufacturing of metals’, we can find many technologies today. Most of these technologies use a laser beam as the power supply, but we can also find other energy sources such as electron beams. The selection of a method based on powder bed or powder feed systems is what truly determine many processing parameters and perhaps includes more restrictions in the alloying design. In [Citation192–195] extended explanations of the different systems and their advantages and disadvantages can be found. Notably, powder bed systems require fully prealloyed powders. These powders are produced by gas atomising to have a spherical shape and a specific size distribution that assures a good packing ratio and enough flowability in the powder to be handled. In powder bed systems, producing changes in the composition is not easy, because we must include all the alloying elements in the prealloyed powder. Preparing a bed of powders from a mix of atomised powders is not appropriate since any small change in the density of the powders belonging to the mix could produce severe segregations in the final composition of the bulk part. Segregations in the mix, when a solidification process is involved, means severe dimensional changes and deformed final parts. The most positive advantage of powder bed systems is that, by ensuring the quality of the prealloyed powder, we could guarantee a good final homogeneity and good dimensional control of the manufactured part.
On the other hand, powder feed methods allow different possibilities while managing the raw material. These methods can use two or more hoppers with different feeders that enable different combinations. For instance, we can place a prealloyed base powder in one hopper and in a second hopper, we can sequentially introduce a fifth or a sixth element with a completely different density. An example of this possibility is illustrated in [Citation84] where in one hopper, an equiatomic mix of CoCrFeNi is introduced with similar density, and in the second hopper, a low-density Al powder is introduced to produce the HEA AlCoCrFeNi.
An interesting issue in additive manufacturing is whether tensile properties are affected by the growth direction of the consolidation. Fujieda et al. [Citation78] developed a study wherein tensile features were compared between tensile samples extracted in the growth direction (tagged 0°) and in the perpendicular direction (tagged 90°). The work was performed for the HEA AlCoCrFeNi (C1 type), and the results were compared with those of the same material produced by casting. compares those results and confirms that, indeed, the best features are obtained in the growth direction of the additive manufactured alloy.
Table 3. Tensile features of AM HEAs in the growth direction and at 90°, and compared with those of an equivalent cast HEA (from [Citation78]).
Another interesting analysis was developed by Joseph et al. [Citation65] who studied the effect of HIP as a posttreatment on an AM HEA, in this case AlxCoCrFeNi (C1 type). In principle, HIP, which is widely used for casting alloys, could be beneficial to collapsing possible shrinkage defects produced during the AM process. This work concludes that this treatment does not significantly improve the AM product, mainly due to two negative effects (that compensate for the densification effect): coarsening in the microstructure, and the possible formation of a σ phase, which is always a risk in these alloys with such a high Cr content.
In addition, materials processed by additive manufacturing can be subjected to annealing, but in this case, the main objective was to release stresses after solidification [Citation77].
6. Mechanical behaviour of PM HEAs
If we classify high-entropy materials into a specific family in the material world, it must be as a ‘structural material’, and as a structural material, the mechanical behaviour is probably the most important feature required in this family of materials. In some of the figures developed for this paper, have been used results from many different sources. Despite in all the referred papers, authors have used standard tests to measure the mechanical properties, it was not possible to assure the same procedures to get all the analysed results (especially in hardness, where the load in the Vickers hardness where different in most of the analysed works, and for this reason is not included in , and ). In this sense, readers are aware of the fact that these data must be treated with care.
6.1. Fine grain and twinning effect
The studied papers about HEAs produced by PM demonstrate that strengthening mechanisms that operate in the alloys are necessarily the same strengthening mechanisms that operate in conventional HEAs. The effect of the solid solutions in alloys, which contain at least five different alloying elements with different structural properties, is very important. Such a high number of alloying elements produces important lattice distortions that can strongly influence the phase transformation, dislocation dynamics and yield of HEAs. Recently, a comprehensive work was published providing a basic understanding of this important issue [Citation196]. However, leaving aside the solid solution effect, the observed twinning after deformation plays an important role in HEAs. Most HEAs possess, at least partially, an FCC structure that produces a twinning effect after deformation, and this twinning effect provides an additional deformation mode that increases the ductility. Twinning also introduces extra interfaces within the grains during deformation, producing a ‘dynamic Hall-Petch’ effect that increases the work hardening rate and, as a consequence, the strength [Citation197]. The strengthening mechanism of nanoscale twins can also be associated with the complicated interaction between dislocations and twin boundaries. Twin boundaries are believed to effectively block dislocation motion, especially when the thickness of the twin/matrix lamellae decreases down to the nanoscale, also reinforcing the Hall–Petch effect [Citation198]. If we combine the high lattice deformation with the twinning effect and consider that twinning is observed in materials with a low stacking fault energy (SFE), the increase in the solute atoms strongly reduces the SFE and increases the nanotwinning tendency [Citation199]. These effects have been widely studied in HEAs produced by ingot metallurgy but have also been confirmed in PMHEAs.
An example of this twinning effect is described in [Citation34,Citation99,Citation149] for the alloys CoNiFeAl0.6Ti0.4, CrCoNiFeAl0.6Ti0.4 and Al0.5CrFeNiCo0.3C0.2, respectively, made by MA and SPS. shows the transmission electron microscopy (TEM) images and corresponding selected-area electron diffraction (SAED) patterns of the FCC phase with nanoscale twins. The nanoscale twins were found only in the FCC phase, which was confirmed by energy dispersive X-ray spectroscopy (EDS)/TEM and the corresponding diffraction patterns.
Figure 13. TEM microstructures and SAED pattern of a twinned FCC phase. (a) A twinned FCC grain with the corresponding SAED pattern and (b) a twinned FCC grain surrounded by Cr23C6 carbide, from [Citation34].
![Figure 13. TEM microstructures and SAED pattern of a twinned FCC phase. (a) A twinned FCC grain with the corresponding SAED pattern and (b) a twinned FCC grain surrounded by Cr23C6 carbide, from [Citation34].](/cms/asset/7eba510f-711b-4a14-9dc5-5ca6086477c1/ypom_a_1584454_f0013_oc.jpg)
In addition, [Citation160] focused on the twinning effect in an AlCrCuFeNiZn HEA produced by MA. The stacking faults in this HEA are due to the solubility of Cu and Zn FCC with other solute atoms. With an increase in the Al content in a copper-aluminium alloy, the twin thicknesses decreased [Citation200,Citation201]. Another important factor in this respect is that the twinning effect under stress is produced mainly in the compression mode, because the twinning produced by tensile load is negligible [Citation13].
More possibilities to increase the twinning effect are reported in some studies on PMHEAs. One possibility is the use of reinforcing particles such as TiC [Citation62]. Introducing particles that can act as hard hinges in the formation of deformation twins is associated with a lower SFE. Another possibility is the presence of dual microstructures. PM can more easily promote the formation of dual microstructures than other processing routes, and in the case of PMHEAs, dual microstructures could also be suitable for combining BCC and FCC structures [Citation174]. A bimodal grain size distribution or ultrafine-grained microstructure could promote the accumulation of dislocations and thus achieve a high strain-hardening rate [Citation202].
6.2. High-temperature behaviour and high-strain-rate deformation
Although HEAs are a promising material for use as structural materials at high temperature [Citation203,Citation204], fewer articles deal with the behaviour of PMHEAs at high temperature.
In some of those works, a piece of Gleeble equipment, specially devoted to simulating thermomechanical treatments, evaluates the compression strength at different strain rates and temperatures. In [Citation174] the behaviour of two alloys, TiNbTa0.5Zr and TiNbTa0.5ZrAl0.2, in compression at 800°C and promoting an engineering strain of 50% is analysed. Specifically, the effect on the microstructure of the precipitates of Nb and Ta and the positive effect of adding Al is investigated. In these alloys, belonging to the C8 cluster where the predominant present phase is BCC, the mechanical behaviour at high temperature is enhanced by the presence of precipitates in grain boundaries and the bimodal microstructure. Another example is the analysis of the CrFeCoNiMo0.2 (cluster C3) alloy [Citation109]. In this case, specimens were tested at different temperatures from 700 to 1100°C. Here, the flow behaviour at high temperature is improved by dynamic recovery and grain growth pinning by the precipitation of a Mo-rich σ phase. In addition, some information on the creep behaviour under compression can be found. In [Citation123] the compressive creep behaviour of an oxide-dispersion-strengthened (ODS) CoCrFeMnNi PMHEA produced by SPS was studied. This work found that in the same material without and with dispersed oxides, the latter exhibited improved performance.
Few works provide information about tensile features at high temperature. In [Citation119] a special PMHEA (Fe18Ni23Co25Cr21Mo8WNb3C2) obtained by MA and HP with a 12 Cr ODS steel is compared. shows that the PMHEA exhibits much higher tensile strength but lower ductility than the ODS steel.
Table 4. Comparison of tensile properties from room temperature to 650 °C, from [Citation119].
Some PMHEAs subjected to a high strain deformation rate exhibit so-called ‘serrated yielding’. In [Citation98] visually explains this concept. In [Citation133] different compressive tests (quasistatic at room temperature and different strain rates, quasistatic at different strain rates and different temperatures up to 900°C and dynamic compressive tests) were performed over a PMHEA (CoCrFeMnNi). According to [Citation21] this phenomenon is more accurate in PMHEA than in an as-cast HEA. The serrations observed on the stress-strain curves of the PM CoCrFeMnNi HEA are caused by the periodic pinning and unpinning process of dislocations in the grains due to the number of dispersed Cr-rich phase particles. A high deformation rate at room temperature also increases the serration yielding [Citation107]. In [Citation98], where tests are performed a low strain rate, the serration behaviour is also observed but in this case, it is attributed to the Al2O3 reinforcements in the Al0.4FeCrCo1.5NiTi0.3 PMHEA.
Figure 14. Stress-strain curve of Al0.4FeCrCo1.5NiTi0.3 reinforced by nano-Al2O3 after SPS under compression. From [Citation98].
![Figure 14. Stress-strain curve of Al0.4FeCrCo1.5NiTi0.3 reinforced by nano-Al2O3 after SPS under compression. From [Citation98].](/cms/asset/0e7dbae9-97fe-443b-86c9-78ac5c7d7362/ypom_a_1584454_f0014_ob.jpg)
6.3. Compression behaviour
Most of the papers dealing with mechanical behaviour use compression tests to evaluate the mechanical behaviour. Therefore, extensive information can be used to analyse this topic. Table S6 of the accompanying supplementary data, Appendix A, includes the results concerning yield strength, ultimate strength, deformation at fracture and hardness for many HEAs, including also HEA obtained by ingot casting [Citation53,Citation206–217]. In the table, the alloy composition, the cluster to which the alloy belongs, the results regarding these properties, and the origin of the information are included. In addition, the processing route followed to obtain the alloy is included for comparison (i.e. if it is a PM route another specific route, or casting).
displays the results concerning the ultimate compressive strength as a function of the deformation at fracture. When we analyse and compare these two properties in the figure, no tendency can be distinguished that could differentiate the materials obtained by conventional ingot casting from those obtained by PM. Perhaps the unique remarkable difference is that some HEAs obtained by casting exhibit a very low deformation at fracture but also a higher aptitude to attain higher levels of deformation, but the analysed sample of papers in this graph does not allow us to extract clear conclusions. collects the results only taking into account the ones belonging to a specific cluster, that is, according to the composition, without considering if the processing is PM or ingot casting. Materials belonging to clusters C1, C3, and C4, based on NiCoFeCr, are spread throughout the figure, showing all the possibilities of strength and deformation. C3 materials exhibit less strength on average (but can reach higher levels of deformation), and C4 materials exhibit a lower level of deformation on average. Those materials are the most common in the literature regarding HEAs and are based on the core group of transition metals (3d transition metals from the 4th period) commonly used in ingot metallurgy. HEAs were developed, in part, from the Cantor alloy [Citation3], based on these elements plus Mn. Leaving aside clusters C2–C5 (with only one alloy), the materials made with clusters C6-C8, that is, refractory HEAs, mainly composed of refractory metals (W, Ta, Nb, Mo, Zr) exhibit much less deformation at fracture.
Figure 16. Ultimate compression strength vs. deformation at fracture considering the cluster composition.
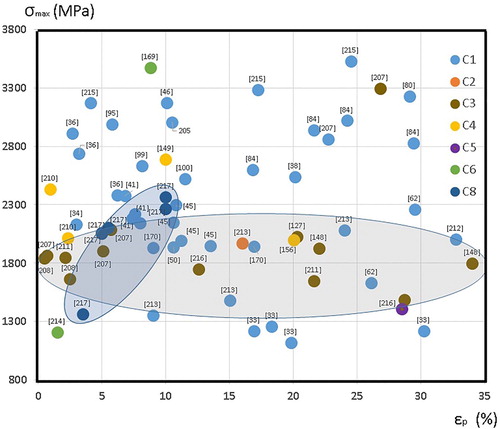
However, if we analyse the hardness against the deformation at fracture (), a slight improvement in the relationship of the hardness/deformation of the cast alloys against the PM alloys becomes more apparent. In any case, these results are based on many different materials (even compositions), manufactured under different processing conditions, and this is just an orientating tendency. Clearly, PM can compete in most fields to provide possibly required properties. Interestingly, the compositions and processing conditions of PMHEAs can be modified for a customised application, in contrast with ingot metallurgy.
6.4. Tensile behaviour
Unfortunately, much less information about the tensile behaviour of PMHEAs is available. Just a few papers regarding tensile features for these materials have been found. Table S7 of the accompanying supplementary data, Appendix A, shows some tensile results (those obtained from papers where reliable tensile data were provided), and results from as-cast HEA [Citation34,Citation77,Citation204,Citation208,Citation218–228], rolled HEAs [Citation26,Citation147,Citation221,Citation229–231], forged HEAs [Citation219], HEAs obtained by high pressure torsion [Citation197]. Other structural alloys belonging to the so-called structural materials are also included, like Ti base alloys [Citation35,Citation232,Citation233], stainless steels [Citation234], Ni base superalloys [Citation235], ODS steels [Citation205,Citation236] or intermetallics [Citation237].
If we plot some of these results for analysis, some clusters of results can be distinguished in . As-cast HEAs can be found in all the spaces of the figure, but could be appreciated in some groupings in the high values of deformation, being as these materials are the conventional structural alloys in this smaller range (and here Ti-based alloys show a little slightly bit better performance). Once again, like as in the compression results, the PMHEAs are distributed in all throughout the space of the graph, but also here this is not relevant at all due to because of the low number of samples. Only in one paper [Citation77] compared the same material (CoCrFeNi HEA) made by additive manufacturing and arc melting, and the results for the PMHEA are clearly positive (σmax/ε in PMHEA of 745 MPa/32% vs. 457 MPa/50%, yield strength of 600 MPa vs. 188 MPa). Furthermore, most of the tensile results for the PMHEAs came from materials produced by additive manufacturing. These are promising results, but this information on this strategy is remarkably lacking, which makes it a good niche to research.
7. Special properties of PM HEAs
Clearly, HEAs have enormous potential as structural materials, especially because of their important mechanical properties, but other important abilities as structural materials beyond the mechanical properties can be found in HEAs. Here, we outline some of these abilities.
7.1. Corrosion and oxidation
The presence in the composition of an alloy of more than 20% (wt.) Cr in combination with Ti and Al powerfully suggests possible good corrosion or oxidation behaviour. Among the works covered in this review, some of them focus on corrosion or oxidation, and among those, most of them belong to compositional clusters C1 to C4, in which Cr, Ti or Al are present.
Considering the HEAs in [Citation32,Citation52,Citation54,Citation104], all of them belong to compositional clusters C1 and C2 made by conventional PM. Electrochemical corrosion is tested through potentiodynamic polarisation curves, and better performance than that of stainless steel 304 was observed in all of these works. In [Citation132], the electrochemical behaviour of a C4 cluster was evaluated by measuring the pitting potential, the results were positive. This later work was devoted to the development of composites (HEA reinforced with SiC), and introducing reinforcements deteriorated the pitting corrosion behaviour.
In [Citation104] the pitting corrosion of three alloys (a CuZr alloy, labelled E2, a CuZrAlTiNi HEA, labelled E5, and stainless steel 304, obtained by MA and SPS) is evaluated by electrochemical polarisation measurements using a potentiostat in an artificial sea water solution at a scan rate of 0.003 V s−1. The artificial sea water mainly consisted of NaCl, MgSO4, MgCl2 and CaCl2. The corrosion resistance of the as-sintered bulk samples in the sea water solution is demonstrated by the potentiodynamic polarisation curves displayed in . The HEA sintered at 620°C exhibits the highest corrosion potential (Ecorr) and the lowest corrosion current density (icorr) with a wider passive region. The HEA sintered at 959°C possesses the best passivity behaviour (the lowest ipass) and a high pitting resistance (with the widest passive region). The corrosion rate (rcorr) can be calculated through Faraday’s law, as shown in the equation below:(1)
(1) where EW is the equivalent weight, and D is the density. shows all these corrosion parameters (and also the pit potential and shows the best behaviour of the HEA sintered at 620 against the stainless steel, tested under the same conditions.
Figure 19. Potentiodynamic polarisation curves of the as-sintered E2 (CuZr alloy) and E5 (CuZrAlTiNi HEA) bulk alloys at different sintering temperatures (from 520 °C to 959 °C), from [Citation104].
![Figure 19. Potentiodynamic polarisation curves of the as-sintered E2 (CuZr alloy) and E5 (CuZrAlTiNi HEA) bulk alloys at different sintering temperatures (from 520 °C to 959 °C), from [Citation104].](/cms/asset/4b960aaf-e968-475a-b07a-9451d5a10b7f/ypom_a_1584454_f0019_oc.jpg)
Table 5. Corrosion parameters of the samples tested (as-sintered E2 -CuZr alloy- and E5 -CuZrAlTiNi HEA- bulk alloys at different sintering temperatures -from 520 to 959°C-, in a sea water solution, from [Citation104].
Only one work [Citation84] has been found in which the behaviour of the HEA is less competitive than that of stainless steel 304, and the reason should be related to the different aging treatments that were applied to the material, this time manufactured by direct laser fabrication.
If the performance against corrosion is quite good, the response against oxidation at high temperature is truly promising. In [Citation47] a cermet based on an HEA (C1 cluster) was studied, and the oxidation tests were performed at the same time as cermets manufactured using conventional Ni/Co binders. The behaviour in the HEA-based cermet is three times superior. Similar behaviour can be found in [Citation54] wherein in addition to the electrochemical corrosion, oxidation was studied. A unique study on a refractory HEA (C7 cluster) made by additive manufacturing can be found in [Citation171]. Here, the behaviour was studied at high temperature in air, and a good performance was observed, suggesting good possible fusion applications.
The results explained above suggest that the HEAs have good potential for a niche of applications requiring good corrosion/oxidation behaviour.
7.2. Friction
Friction and wear usually go together in the classification of properties, but we will devote some space to wear in the next paragraph. Through some slight modifications to the composition of the HEA which is suitable thanks to the flexibility of the PM process, important improvements have been made in the friction behaviour of the alloys. Friction behaviour is important in a wide number of applications, especially in bearings. The chapter devoted to composites based on HEAs (HEACMs) in [Citation163], includes the profile of materials focused on here. In this later work, the advantages of PM for the development of this family of materials are highlighted because as-cast HEAs contain segregations, multiple phases, and brittle intermetallics at grain boundaries, all of which negatively influence the ductility and toughness and damage the friction features of these alloys.
Yadav et al. [Citation164,Citation165] performed an interesting study adding Bi and Pb dispersoids to two different HEAs, AlCrFeMnV (C9 cluster) and CuCrFeTiZn (C5 cluster), respectively. In both cases, the friction coefficient was reduced, and the wear resistance was increased by approximately 20%. In both cases, the mechanical properties of the base HEA were not significantly affected. In [Citation140] a similar study considering the addition of Mo to a base HEA (CoCrCuFe) was performed. The presence of the Mo introduced some precipitates into the microstructure that modify the friction behaviour, reducing the friction with the increasing amount of Mo.
The works of Zhang et al. [Citation120,Citation128] are in the same direction. In these studies, the base alloy was the core alloy of the C1–C4 clusters (CoCrFeNi), and the additions to the alloy were Ni coated with graphite, Ni coated with MoS2 and S (added as FeS). The friction and wear behaviour from room temperature to 800°C was studied. In all the cases, excellent self-lubrication behaviour and good wear resistance were exhibited. The friction coefficient was always reduced, and the wear performance improved up to five times at room temperature. In addition, the mechanical properties were not highly compromised.
To end this section on the friction properties, an interesting work on the machinability of an HEA manufactured by AM [Citation136] is discussed. Different machining conditions were applied to the CoCrFeNiMn alloy (C3 cluster), and the report concluded that with the suitable process combination, any practical surface quality and dimensional accuracy could be obtained.
7.3. Soft magnetic features
Ji et al. [Citation105] explored the soft magnetic features of a PM HEA (CoCrFeNiMn, C3 cluster). The hysteresis curve of the powders, after 60 h of MA, exhibits soft magnetic properties (see ). The values of the saturated magnetization and the remanence ratio are higher and lower, respectively, than values available in the literature [Citation238] for a similar cast alloy. This result indicates that the as-milled powder can be used as a soft magnetic material. Moreover, the powder exhibited superparamagnetic behaviour, observed for magnetic particles with sizes under 10 nm [Citation239]. Unfortunately, after SPS, the coarsening of the grains modified both the soft magnetic and the superparamagnetic features of the alloy.
Figure 20. Magnetic hysteresis curves of the CoCrFeNiMn HEAs measured at room temperature, from [Citation105].
![Figure 20. Magnetic hysteresis curves of the CoCrFeNiMn HEAs measured at room temperature, from [Citation105].](/cms/asset/d680e45a-fb45-4939-9d46-f28ca30e40d6/ypom_a_1584454_f0020_oc.jpg)
In a similar way, is the study of Yu et al. [Citation106], but in this case succeeding in a PM consolidated HEA. In this paper, the change of just one element in the alloy leads to a drastic change in the magnetic response. With the same alloy than the previous work of Ji et al. [Citation105] a similar result was obtained, but the Mn was changed to Cu in the composition (CoCrFeCuNi, also in the C3 cluster). The levels of the saturated magnetization, the remanence ratio and the coercivity, even after SPS, enable using this PM HEA in soft magnetic applications. The value obtained for the saturated magnetization is higher than that of the CoCrFeNiCuTi HEA obtained by casting [Citation238].
These studies demonstrate that this is another promising field of application for PM HEAs.
7.4. Hydrogen storage
Hydrogen is considered a good alternative to fossil fuels, but its possible application is linked to the development of materials capable of storing it. Some works have demonstrated the possibility of some alloys with the BCC structure to react with hydrogen, thus constituting a promising hydrogen storage material [Citation240]. Many HEA compositions can ensure the BCC structure with the proper combination of alloying elements (see ). Kunce et al. [Citation151,Citation175] produced two PM HEAs by additive manufacturing, with two different compositions (TiZrNbMoV and ZrTiVCrFeNi, belonging to the C8 and C5 clusters), trying to exploit the BCC structure for hydrogen storage. The feasibility of this potential application was measured through pressure-composition-temperature experiments, and both alloys can absorb and desorb hydrogen (until the hydrogen is 1.8% and 0.59% in weight, respectively). In the context of this experimental setup, the alloys were unable to completely desorb hydrogen.
Similarly, in another study involving PM HEA [Citation155] (MgZrTiFeCoNi, C4 cluster) tests were performed on the powder, and the phase transformation when the hydrogen was absorbed and desorbed was studied through in situ synchrotron XRD measurements. In this case, the samples could absorb and fully desorb 1.2% hydrogen by weight. Apparently, the structure changed from BCC to FCC during the absorption process and from FCC to BCC during the desorption process.
8. Alloying opportunities for PM HEAs
After the chapter related to special properties, and more in detail in the topic about ‘friction’, we have an example about ‘alloying opportunities’ in PM. In this paragraph, what was explained in terms of alloying procedure, only can be possible through PM technology. Now, we will develop other alternatives that only can be introduced into the HEA field by using PM.
8.1. HEA-strengthen metal matrix composites (MMCs)
We found some works where HEA powders are used as a ‘reinforcement’ in a metal matrix. The first example is a work developed by Chen et al. [Citation76] where the selected matrix is Cu. In this work, an AlCoNiCrFe (C1 cluster) powder is used to reinforce pure Cu. The HEA powder was obtained by MA and then mixed (low-energy milling) and sintered (hot pressed at 800°C/70 MPa/30 min). The composite was made by adding 10 and 20% (wt) HEA powder. The main result was an important increase in the compressive yield strength, without a significant decrease in the ultimate compression strength or the deformation at fracture.
One more typical configuration for MMCs is aluminium-based composites. In [Citation241] using the AA6061 as the matrix and a conventional PM route (mix-cold compaction-sintering), an MMC is manufactured using a CrMnFeNiCu PM HEA (C5 cluster). Two different amounts of reinforcements (10 and 20% wt) were used, and in both cases, the compressive strength was increased significantly (in the case of the 10% reinforcement, the fracture compressive strength was double that of the base alloy). In the work of Karthik et al. [Citation242] an aluminium matrix (in this case an AA5083 alloy) and HEA as reinforcement (CoCrFeNi, C3 cluster) were also used, in this case using 12% (vol) as reinforcement. Interestingly, this work used an additive manufacturing method based on friction deposition using a friction stir welding equipment. The obtained composite significantly improved the mechanical properties relative to those of the matrix (i.e. the ultimate tensile strength increased from 300 to 395 MPa and the HRB from 30 to 549), except the elongation, which reduced from 12% to 5%, as could be expected.
In this chapter, the work of Tan et al. [Citation81] is also of interest. In this case, an HEA was also used as a reinforcement (AlCoCrFeNi, C1 cluster), but the matrix, prepared from an amorphous Al alloy (Al65Cu16.5Ti18.5) that was manufactured by MA, was more innovative. To avoid microstructural coarsening, the composite was consolidated by SPS. Here, both the matrix and composites exhibit a very brittle behaviour, but the ultimate tensile strength of the composite increased from 1700 to 3120 MPa.
shows the powders used and the microstructure of the composite.
Figure 21. SEM images of the as-milled amorphous Al alloy powder (a), (b), the HEA gas-atomised particles and c) the composite; (d) magnified image corresponding to the area marked by the rectangular in (c), from [Citation81].
![Figure 21. SEM images of the as-milled amorphous Al alloy powder (a), (b), the HEA gas-atomised particles and c) the composite; (d) magnified image corresponding to the area marked by the rectangular in (c), from [Citation81].](/cms/asset/ad7afb8f-0433-48bf-87cf-780a31191bca/ypom_a_1584454_f0021_oc.jpg)
8.2. Particle reinforced HEA composites-HEA matrix composites – (HEAMCs)
In this case, the MMCs are composed of an HEA matrix and reinforced with Al2O3 or SiC. Table S8 of the accompanying supplementary data, Appendix A, is displayed the composition, the processing route and some compression properties and hardness of some works developed in this direction. In the bottom part of the table, some results regarding the same HEA without reinforcement and some other works from different authors for comparison. Although in most of the articles, the size of the reinforcement is in the range of nanometres, the effect of these reinforcements on the mechanical properties is not as important, as they improve only the compressive yield strength and the hardness.
There are another two other works, focused on the same material (CoCrMnNi, cluster C1 reinforced 5% − wt.- of SiC <50 nm, manufactured through MA + mMixing + HIP), but focused ion the electrochemical response (without reporting any information regarding mechanical properties). As could be expected, the presence of nanoparticles in the microstructure introduced local Cr-depleted zones, and this could promote an acceleration of the corrosion process.
can help us analyse these results, considering that the plot shows differences in terms of composition and reinforcement, but differences also exist in other important variables such as the size of the reinforcement and the processing conditions. Clearly, the influence of reinforcement in conjunction with changes in composition can cause important changes in the mechanical properties, helping us to tailor the material to one specific application.
Figure 22. Ultimate compression strength vs. deformation at fracture for some HEA base composites and HEAs.
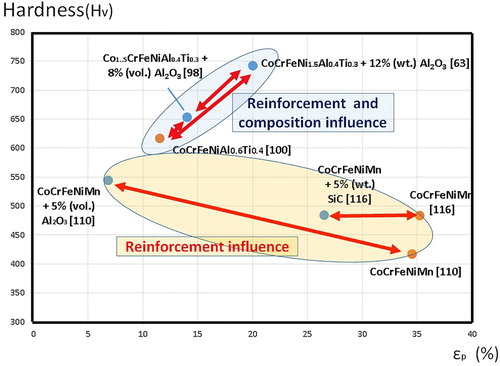
Sharma et al. [Citation163] highlighted the possible role of these HEAMCs in applications requiring improved wear and friction properties beyond the required mechanical properties.
8.3. Cemented carbides/cermets
Cemented carbides (mainly based on WC as the hard phase and Co as the binder) and cermets (mainly based on TiCN as the hard phase and Co-Ni as the binder) have been competing for years in the cutting tool markets and in wear applications. However, for many different reasons, the last 15 years have seen a tendency both at the industrial level and in academia to find new materials for cutting and wear applications, trying to avoid Co and Ni as binders. Some of the reasons for this research are related to some specific properties such as resistance to corrosion or oxidation, but others are linked to the strategic role of Co and Ni in the global market and the health issues related to Co and Ni. Considering these circumstances, the appearance of HEAs as materials with a simple structural configuration (BCC or FCC), along with promising oxidation and corrosion performance, a promising behaviour at high temperature and suitable for processing by PM, makes this new family of alloys a strong candidate to be used as binders in hardmetals.
Since the appearance of PM HEAs, many works were performed to try to analyse the feasibility of these materials as a binder, especially since 2014 ([Citation47,Citation150,Citation243]) and 2015 [Citation48]. Over the last few months, there has been a dramatic increase in papers dealing with this topic ([Citation62,Citation129,Citation159,Citation167]). Many of these papers deal with improving the oxidation behaviour [Citation47] or the wear behaviour [Citation131,Citation138,Citation157,Citation243] and the relationship between the fracture toughness and hardness (always in comparison with the conventional cermets/cemented carbides based on Co or Co-Ni). In this latter respect, analyses some results from different articles and compares these results with some traditional Co-WC [Citation243–250] or Co-WC-VC [Citation45,Citation95,Citation251–253] hardmetals produced by equivalent processing routes. Table S9 of the accompanying supplementary data, Appendix A, includes information regarding the binder phase (an HEA or a Co-based binder), as well as the hard phases and the processing route, including results concerning hardness and fracture toughness.
Figure 23. Hardness vs. fracture toughness of different cermets and cemented carbides made with HEA binders, and some conventional Co-based materials for comparison.
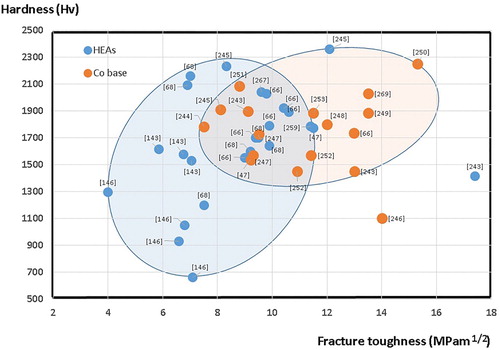
This figure shows how PMHEAs are competitive with other conventional hardmetals. In a large space, the range of properties is similar, and we have to consider that most of materials processed with conventional composition have been manufactured with hard phases from nanosized powders and materials with enhanced fracture toughness [Citation254]. Most of the HEAs used in these studies belong to the C1 cluster. Refractory HEAs (clusters C6–C7–C8) with, in principle, higher hardness and presumably better behaviour at high temperature could be good candidates to develop cermets and cemented carbides [Citation163].
One important issue to be solved in the development of cermets is the wetting behaviour of the binder in the hard phase, in this case the HEA on the TiC or the TiNC. This topic has been treated in some works [Citation48,Citation150,Citation159]. Some of these studies have performed wetting studies using the sessile drop test on TiCN substrates, which demonstrated the very good wetting of the HEA.
To finalise this chapter, it is also interesting to mention that [Citation138] suggests that HEAs can also be a good binder to manufacture diamond tools.
8.4. Oxide dispersion strengthened (ODS) alloys: HEAODS
Three works were found related to this topic, two performed by Hadraba et al. [Citation115,Citation123] and the third by Moravcik et al. [Citation255]. This very interesting strategy may open a completely new research area of high interest. In the first two works, an HEA (CoCrFeNiMn, C3 cluster) was manufactured by MA and SPS (1150 °C/50 MPa/5 min). The same alloy was produced by adding oxygen, Y and Ti during the MA step to produce dispersed oxides on the order of 0.3% (wt). The mechanical properties (in compression and tension) and the creep behaviour in uniaxial compression mode (between 700 and 800°C) were tested in these studies. The results were quite promising because the yield strength (both at compression and tension) and the ultimate tensile strength increased on the order of 30% in the case of the ODS alloy, and in the tests performed at 800°C, the response increased by up to 70%. In addition, the results of the compression creep study showed better performance in the ODS HEA. No work has compared these HEAODSs with ODS steels tested under the same conditions, but the values obtained can be considered promising. The negative aspect is, similar to that of the ODS steels, the ductility was much lower in the HEAODSs than that of the original alloy.
In [Citation255] the HEA (CoCrFeNiTi, C4 cluster) is also manufactured by MA and SPS (1150°C/30 MPa/20 min.). After the MA a powder with a preferential FCC phase is obtained, but after SPS some intermetallic (Ni3Ti type) can be found. In this work after the SPS consolidation, the bulk material is submitted to different annealing temperatures (700, 900 and 1100°C) under Ar atmosphere. After the annealing grain growth is produced and some dispersed oxides and also the above-mentioned intermetallics finely dispersed in the structure make some changes in the mechanical behaviour. The highest values for tensile strength are obtained for the SPS materials but an slightly decrease in the UTS values (from 1569 to 1467 MPa) are compensated with an important increase of the elongation in the annealed materials (from 6 to 14%).
9. Future work
Many studies have been performed on PM HEAs, but many further works could be done to improve the knowledge of this family of materials. Today, so many instruments are available that can be used in materials science that the space to explore any field of materials is unlimited. In the next sections, some ideas are introduced to offer some possible research lines to those who want to be introduced to the PM HEA field, to enable them to develop knowledge in those spaces with room for innovation. These points are not exhaustive, and some matters are not covered, but all the proposed topics can be considered important.
9.1. Thermodynamic modelling
Today, there are many tools to develop accurate thermodynamic calculations to predict the expected phases for a determined composition at a specific temperature. In a complex system such as an HEA, using these tools to have an idea about what we can estimate for a certain combination of elements could be an important advantage. For instance, we have seen in many works how the structural composition could change by modifying the composition of one single element such as Al [Citation44,Citation55,Citation126], Ti [Citation85,Citation171], Fe [Citation33], Mn [Citation176] or the modification of two elements, such as Al-Ti [Citation67] or Cu-V [Citation170]. Through thermodynamical calculations, those changes could be calculated and predicted before investigating them experimentally. One example is shown in [Citation147], , wherein a pseudobinary phase diagram (CoFeCrNi)-Mo (obtained with the help of Thermo-Calc software, based on the Calphad method [Citation256]) is analysed for the possible presence of σ and µ phases depending on the Mo content. On the diagram are marked lines and stars with the composition of the (σ+µ) precipitates on the γ matrix, for each of the studied alloys (Mo0.1, Mo0.2 and Mo0.3).
Figure 24. The pseudo binary phase diagram of the (CoCrFeNi)-Mo alloy system. The star stands for a mixture of the FCC, σ and µ phases in the Mox (x = 0.1, 0.2 and 0.3) at 973 K (700 °C), 1073 K (800 °C), 1173 K (900 °C) and 1273 K (1000 °C). From [Citation147].
![Figure 24. The pseudo binary phase diagram of the (CoCrFeNi)-Mo alloy system. The star stands for a mixture of the FCC, σ and µ phases in the Mox (x = 0.1, 0.2 and 0.3) at 973 K (700 °C), 1073 K (800 °C), 1173 K (900 °C) and 1273 K (1000 °C). From [Citation147].](/cms/asset/932cea5c-aa6f-459c-98c5-472ee2f1f3c7/ypom_a_1584454_f0024_oc.jpg)
In all the papers studied to prepare this review, only a few used any kind of thermodynamical calculations. More than the abovementioned paper highlight the use of Thermo-Calc to predict the present phases (FCC, BCC, liquid) as a function of the temperature in the AlCoCrFeNi system [Citation58] and to predict the presence of graphite in an HEA-based cemented carbide as a function of the carbon content [Citation173]. Thermo-Calc was also used in [Citation174] to predict the present phases (HCP, BCC and liquid) as a function of the temperature in the TiNbTa0.5Zr and TiNbTa0.5ZrAl0.2 systems. In [Citation153] another thermodynamic software, JMAtPro [Citation257], was used to simulate the phase equilibrium in the solidification of the NiCrCoTiV system.
In their review of nanocomposites and HEAs, Sharma et al. [Citation163] emphasised the important role of thermodynamical calculations in the design of new possibilities in this field.
9.2. Thermal analysis
Many thermal analysis techniques could be applied in the development of PM HEAs that could help to reduce the weight of experimentalism in the development of the final proposed alloy. However, only a few techniques focus on some specific steps of the processes. Differential scanning calorimetry (DSC) and differential thermal analysis (DTA) have been widely used to analyse the phase transformation in the atomised or mechanically alloyed powders before the consolidation step (i.e. [Citation143,Citation159] among many others), but only a few works use these kinds of techniques to study the consolidated material manufactured by the conventional PM route [Citation141,Citation161]) or AM ([Citation77,Citation258]). In [Citation65] and [Citation166] thermal analysis techniques are used to evaluate changes with different cooling or heating rates. In the case of [Citation12] CCT (continuous cooling temperature) diagrams are developed (later the application to heat treatments will also be discussed). Dilatometry, which is a highly useful technique used in the development of many PM families of materials, is scarcely used for PM HEAs [Citation124].
Information about the appearances or disappearances of desired phases (such as a specific BCC or FCC phase) or undesired phases (σ phase, carbides or intermetallics, for example), the real melting point of any HEA system, the kinetics of the transformations in the phases, the dimensional behaviour with the temperature or the possible undesired oxidation processes could be analysed and studied with thermal analysis techniques. This is another good opportunity for studying this family of materials.
9.3. Structural and microstructural knowledge of the PM HEAs
In most of the analysed works, the present phases have been thoroughly studied by XRD and/or TEM. However, only a few studies are complemented with an electron back-scattered diffraction (EBSD) analysis. In the world of HEAs, in principle, the size of the grain in the microstructure, the orientation of the phases, the twinning effect, etc. are much important than in other families of alloys because they are one multicomponent alloy, usually with only one main phase in the microstructure. For this reason, small changes in some microstructural features could be crucial to improving the mechanical properties, and here, the role of EBSD could be important. For example, [Citation34,Citation100,Citation145,Citation148] analysed twinning and its effects on the mechanical properties (using TEM), but only in the last work was EBSD used to demonstrate the main conclusions.
Nevertheless, some interesting uses of EBSD have been found for different purposes. In many works [Citation51,Citation89,Citation113,Citation130,Citation135,Citation137] where the shaping method is an AM technique, it is important to analyse the effect of the growth direction on the consolidation, grain size and grain orientation, and the mechanical properties. Thus, EBSD has been used profusely in these works. In other articles, the shaping method exhibited a special influence on the grain size and its orientation, such as shock wave compaction [Citation114] or hot extrusion [Citation198]. EBSD is important when a tool is needed to easily distinguish the FCC and the BCC phases with the same chemical composition [Citation88] or when the grain size is crucial in the range of properties to be studied, as in the case in the creep mode [Citation123] or in the ODS alloys [Citation115]. To finish with this topic, we also mention those works where the heritage of the grain from the powder is important in the further development of the processing, which is the case when we modify the grain size and the size distribution of the original powder [Citation121].
In alloys with high complexity in terms of alloying elements such as the HEAs, the partitioning of the different elements in the different grains/phases of the microstructure and the possible correlation with those microstructural features that affect the properties could be an interesting area of study. This information can be obtained by many techniques linked with electron microscopy and perhaps the most powerful technique, by atom probe. The use of this technique is scarce in the analysed papers [Citation84,Citation118].
9.4. Heat treatments
Heat treating monophasic or biphasic alloys, wherein phases are stable in most of the range of temperatures and the solubility does not change as a function of the phases, appears to make no sense at first glance. However, many papers have signalled that heat treatments may be promising for PM HEAs.
In [Citation103] an AlCoCuZnNi (cluster C2) PM HEA was developed by MA and SPS. The main obtained phase was FCC after both MA and SPS. SPS was applied at different temperatures, and the formation of a minor phase with an ordered FCC structure L12 that precipitated in the main FCC phase was detected. Those precipitates change in size and shape depending on the temperature as does the measured hardness. This L12 phase exhibited a stoichiometry close to that of Co9Al6Ni3(Cu,Zn). The authors explained the strengthening mechanism by comparing it with the mechanism of the γ′ (Ni3Al) phase in Ni-based superalloys that precipitate in the γ phase, which is a solid solution of Ni with the other elements. The presence of these ordered precipitates (in volume, shape, size) modifies the mechanical behaviour of the superalloys at high temperature and the coherency strain between the precipitates and the matrix. There is clearly an opportunity for heat treatments of PM HEAs.
Another example can be found in [Citation80] where the AlCoCrFeNi (cluster C1) PM HEA is made by dry mixing and SPS. After SPS, an FCC phase and two different BCC phases (one ordered and one disordered) are found in the microstructure. The microstructural configuration of these phases is a network of the FCC phase and surrounding grains containing a matrix of the ordered BCC with precipitates of the disordered BCC (see ).
Figure 25. Microstructures of the SPSed AlCoCrFeNiHEA:(a) low-magnification back-scattered electron image (BEI), (b) high-magnification secondary electron image (SEI) of the BCC phase, from [Citation80].
![Figure 25. Microstructures of the SPSed AlCoCrFeNiHEA:(a) low-magnification back-scattered electron image (BEI), (b) high-magnification secondary electron image (SEI) of the BCC phase, from [Citation80].](/cms/asset/fb5276f9-b2a7-45de-bc31-8629845f6a38/ypom_a_1584454_f0025_ob.jpg)
In the right image of , the microstructure is quite similar to the γ/γ′ structure in Ni-based superalloys. As in the previous example, the size, distribution and morphology of these phases are temperature/time dependent, and the coherent strain between the phases is responsible for the final mechanical properties. The advantages of having a dual microstructure with BCC and FCC that could combine hardness and ductility could also be beneficial for wear applications [Citation163]. In paragraph 6.1 was also discussed how a bimodal grain structure enhance the twin effect and the mechanical properties.
In the two previous examples, simply adjusting the temperature can substantially modify the relationship among the present phases and their influence on the microstructure and the mechanical properties. Using temperature to finely tune the microstructure is clearly a research field to be explored.
However, changes in the main phase are not the only desirable changes. In PM, many other phases can appear and disappear in HEAs; most of these phases are undesirable, but a convenient treatment could be used to improve the microstructural features and properties. Among these phases, a σ phase was observed in many compositions [Citation58,Citation67,Citation176] among many others), as well as carbides, especially Cr23C6 [Citation85,Citation116,Citation131] and intermetallics such as Al3Ti ([Citation81]). All of these phases can be properly treated (through heat treatments) to optimise their presence in the microstructure.
In this sense, a very interesting approach was demonstrated in [Citation65]. In this work, three different PM HEAs with different Al contents (AlxCoCrFeNi, cluster C1) were tested. The samples were manufactured from gas-atomised powders and HIP. The HIP treatment was adapted to produce different cooling rates and thus to prepare continuous cooling diagrams (). This type of diagram enables the planning of possible heat treatments that could control the presence of the σ phase. In this work, the presence of the σ phase produced a notable loss of ductility, which could be avoided with the proper cooling rate.
The highest strength conventional metals and alloys used at high temperatures almost always rely on the controlled distribution of a second phase [Citation16]. This common strategy to increase strength in most structural materials is also suitable for HEAs, and an appropriate way to follow this recommendation is heat treatment.
Figure 26. Cooling curve transformation diagram for Al0.85CoCrFeNi HEA, from[Citation65].
![Figure 26. Cooling curve transformation diagram for Al0.85CoCrFeNi HEA, from[Citation65].](/cms/asset/a138bcbb-5815-44f2-925f-fc59121df896/ypom_a_1584454_f0026_oc.jpg)
9.5. Mechanical properties at high temperature
The main review papers published on HEAs [Citation5,Citation8,Citation18,Citation42] emphasised the potential of these alloys for high-temperature applications. Some reviews have specifically focused on the high potential of these materials for high-temperature applications [Citation203,Citation204]. However, in chapter 7 of this review, a few papers are discussed that deal with tensile properties, and in particular, an unusually high number of papers discussed the behaviour of PM HEAs at high temperature. To validate PM as an alternative processing route to produce HEAs, this manufacturing strategy must be shown to be competitive in the high-temperature niche. Therefore, high-temperature properties are the last topic proposed for future work. This topic has been covered less than the other topics, but it may be the most important gap in the literature to fill.
9.6. High-pressure torsion
It has been found just a recent work on this topic, and related HEAs [Citation259]. A HEA (CoCrFeMnNi, C3 cluster) is developed by using high-pressure torsion directly form elemental powders. A high pressure of 5 GPa is used and bulk material with a very fine grain and extremely high hardness (over 6700 MPa) is obtained. Without any further heat treatment, just using pressure, a FCC+BCC structure is obtained. This work is the first one published in this field and opens another interesting field of research.
10. Summary
This review article has covered the field of HEAs consolidated by PM. In this sense, this review focused on powder development and PM consolidation and the related properties obtained by this processing route demonstrated in papers dealing with this central matter. In addition, potential opportunities for PM to produce ‘different’ HEAs are also discussed since PM offers some alternative routes to obtain special compositions. Moreover, PM represents an easy way to obtain some special materials (especially composites) that are difficult to obtain by conventional casting routes. This review shows that the potential of PM is vast, and the level of the obtained properties is highly competitive with HEAs obtained by ingot casting for many different fields. PMHEAs may even be more competitive with traditional materials for the HEAs in some specific applications. However, this review demonstrates that considerable work must be done to provide the market with reasons to use PM instead of other processing routes. Now that PM is established as a processing route, further research must be conducted to cover properly the topic.
Supplemental Material
Download MS Word (251.4 KB)Acknowledgements
Authors wish to thank Dr Mario Torralba for his help in the statistical analysis developed in section 2 of this paper.
Disclosure statement
No potential conflict of interest was reported by the authors.
Notes on contributors
Professor J. M. Torralba has published more than 500 scientific papers, has supervised 27 Ph.D. thesis and 900 Diploma Thesis. He has received many awards being Fellow of the American Powder Metallurgy Institute and the European Powder Metallurgy Association. He has been involved in 35 competitive projects (in regional, national and international frameworks), being the principal investigator in 25. He has managed several research grants with industries, including the Höganäs Chair in Powder Metallurgy. His main scientific interest and activity is related to Powder Metallurgy. He is Regional Editor of Journal of Materials Processing Technology and Metals. Editor in Chief (coeditor) of Powder Metallurgy.
Dr P. Alvaredo completed the degree of Chemistry at Universidad Autónoma de Madrid (UAM) and a Master Degree in Materials Science and Engineering at Universidad Carlos III de Madrid where she also obtained her Ph.D. in Materials Science and Engineering. Her activity is developed in the field of cermets and cemented carbides, high entropy alloys and Ti alloys. All of her main research lines are focused in Powder Metallurgy technology. Within the framework of Powder Metallurgy she has worked in the design of new compositions using thermodynamic simulation methods, characterisation of powders and consolidation by conventional PM routes and Additive Manufacturing techniques. During her career Paula Alvaredo has been involved in 11 international and national projects. She has published 15 scientific papers in peer-reviewed international journals and she has co-authored 37 presentations in international and national conferences.
Dr Andrea García-Junceda graduated in both Chemistry and Materials Engineering at the Complutense University of Madrid. Ph.D. in Materials Science and Technology from the same university in 2007. She is currently leading the Solid State Processing Group at IMDEA Materials Institute, located in Madrid. This group is collaborating with important leading research centres, universities and companies in the field of powder metallurgy. The main goal of the group is focused on the design and development of advanced alloys with outstanding properties by PM routes. She is co-author of 28 journal publications. She has participated in 50 conferences and she has been involved in 14 research projects. In addition, currently she works as an associate professor at Carlos III University of Madrid.
ORCID
J. M. Torralba http://orcid.org/0000-0003-2032-4144
Andrea García-Junceda http://orcid.org/0000-0003-4100-8730
References
- Radetzki M. Seven thousand years in the service of humanity—the history of copper, the red metal. Resour Policy. 2009 Dec;34(4):176–184.
- Kim Y-W. Intermetallic alloys based on gamma titanium aluminide. JOM. 1989 Jul;41(7):24–30.
- Cantor B, Chang ITH, Knight P, et al. Microstructural development in equiatomic multicomponent alloys. Mater Sci Eng A. 2004 Jul;375–377:213–218.
- Yeh J-W, Chen S-K, Lin S-J, et al. Nanostructured high-entropy alloys with multiple principal elements: Novel alloy design Concepts and Outcomes. Adv Eng Mater. 2004 May;6(5):299–303.
- Miracle DB, Senkov ON. A critical review of high entropy alloys and related concepts. Acta Mater. 2017 Jan;122:448–511.
- Yeh J-W. Recent progress in high-entropy alloys. Ann Chim Sci des Matériaux. 2006 Dec;31(6):633–648.
- Tsai M-H, Yeh J-W. High-entropy alloys: a critical review. Mater Res Lett. 2014;2(3):107–123.
- Ye YF, Wang Q, Lu J, et al. High-entropy alloy: challenges and prospects. Mater Today. 2016;19(6):349–362.
- Pickering EJ, Jones NG. High-entropy alloys: a critical assessment of their founding principles and future prospects. Int Mater Rev. 2016;61(3):183–202.
- Cantor B. Multicomponent and high entropy alloys. Entropy. 2014;16(9):4749–4768.
- Tsai M-H. Physical properties of high entropy alloys. Entropy. 2013;15(12):5338–5345.
- Zhang Y, Zuo T, Cheng Y, et al. High-entropy alloys with high saturation magnetization, electrical resistivity, and malleability. Sci Rep. 2013 Mar;3:1455.
- Shi Y, Yang B, Liaw PK. Corrosion-resistant high-entropy alloys: a review. Metals. 2017;7(2):43.
- Qiu Y, Gibson MA, Fraser HL, et al. Corrosion characteristics of high entropy alloys. Mater Sci Technol. 2015;31(10):1235–1243.
- John Mary S, Nagalakshmi R, Rajendran S, et al. High entropy alloys and corrosion resistance. A bird’s eye view. Eur Chem Bull. 2014;3(12):1031–1035.
- Miracle DB, Miller JD, Senkov ON, et al. Exploration and development of high entropy alloys for structural applications. Entropy. 2014;16(1):494–525.
- Miracle DB. Critical assessment 14: high entropy alloys and their development as structural materials. Mater Sci Technol. 2015;31(10):1142–1147.
- Zhang Y, Zuo TT, Tang Z, et al. Microstructures and properties of high-entropy alloys. Prog Mater Sci. 2014;61:1–93.
- Gludovatz B, Hohenwarter A, Catoor D, et al. A fracture-resistant high-entropy alloy for cryogenic applications. Science. 2014;345(6201):1153–1158.
- Diao HY, Feng R, Dahmen KA, et al. Fundamental deformation behavior in high-entropy alloys: an overview. Curr Opin Solid State Mater Sci. 2017 Oct;21(5):252–266.
- Li W, Liaw PK, Gao Y. Fracture resistance of high entropy alloys: a review. Intermetallics. 2018;99:69–83.
- Murty BS, Yeh JW, Ranganathan S. High-entropy alloys. Amsterdam: Elsevier Science; 2014.
- Gao MC, Yeh J-W, Liaw PK, et al., Eds. High-entropy alloys. Cham: Springer International Publishing; 2016.
- Varalakshmi S, Kamaraj M, Murty BS. Synthesis and characterization of nanocrystalline AlFeTiCrZnCu high entropy solid solution by mechanical alloying. J Alloys Compd. 2008 Jul;460(1–2):253–257.
- Zhang KB, Fu ZY, Zhang J, et al. Nanocrystalline CoCrFeNiCuAl high-entropy solid solution synthesized by mechanical alloying. J Alloys Compd. 2009;485(1):L31–L34.
- Chen Y-L, Hu Y-H, Tsai C-W, et al. Alloying behavior of binary to octonary alloys based on Cu–Ni–Al–Co–Cr–Fe–Ti–Mo during mechanical alloying. J Alloys Compd. 2009 May;477(1–2):696–705.
- Koch CC. Nanocrystalline high-entropy alloys. J Mater Res. 2017;32(18):3435–3444.
- Kumar A, Gupta M. An Insight into Evolution of light weight high entropy alloys: a review. Metals. 2016;6(9):199.
- Senkov ON, Wilks GB, Miracle DB, et al. Refractory high-entropy alloys. Intermetallics. 2010 Sep;18(9):1758–1765.
- Yeh J-W. Alloy design strategies and future Trends in high-entropy alloys. JOM. 2013;65(12):1759–1771.
- Milligan GW, Cooper MC. A study of the comparability of external criteria for hierarchical cluster analysis. Multivariate Behav Res. 1986;21(4):441–458.
- Qiu X-W. Microstructure and properties of AlCrFeNiCoCu high entropy alloy prepared by powder metallurgy. J Alloys Compd. 2013 Apr;555:246–249.
- Yuhu F, Yunpeng Z, Hongyan G, et al. Alnicrfexmo0.2CoCu high entropy alloys prepared by powder metallurgy. Rare Met Mater Eng. 2013 Jun;42(6):1127–1129.
- Fang S, Chen W, Fu Z. Microstructure and mechanical properties of twinned Al0.5CrFeNiCo0.3C0.2 high entropy alloy processed by mechanical alloying and spark plasma sintering. Mater Des. 2014;54:973–979.
- babu CS, Sivaprasad K, Muthupandi V, et al. Characterization of nanocrystalline AlCoCrCuNiFeZn high entropy alloy produced by mechanical alloying. Procedia Mater Sci. 2014 Jan;5:1020–1026.
- Fu Z, Chen W, Chen Z, et al. Influence of Ti addition and sintering method on microstructure and mechanical behavior of a medium-entropy Al0.6CoNiFe alloy. Mater Sci Eng A. 2014 Dec;619:137–145.
- Zhang H, Pan Y, He Y, et al. Microstructure and properties of 6FeNiCoSiCrAlTi high-entropy alloy coating prepared by laser cladding. Appl Surf Sci. 2011;257(6):2259–2263.
- Shaofeng Y, Yan Z, Jialin C, et al. Microstructure and properties of Al0.4FeCrNiCo1.5Ti0.3 high entropy alloy prepared by MA-HP technique. Rare Met Mater Eng. 2014 Dec;43(12):2948–2952.
- Ji W, Fu Z, Wang W, et al. Mechanical alloying synthesis and spark plasma sintering consolidation of CoCrFeNiAl high-entropy alloy. J Alloys Compd. 2014;589:61–66.
- Zhu G, Liu Y, Ye J. Fabrication and properties of Ti(C,N)-based cermets with multi-component AlCoCrFeNi high-entropy alloys binder. Mater Lett. 2013 Dec;113:80–82.
- Chen Z, Chen W, Wu B, et al. Effects of Co and Ti on microstructure and mechanical behavior of Al0.75FeNiCrCo high entropy alloy prepared by mechanical alloying and spark plasma sintering. Mater Sci Eng A. 2015;648:217–224.
- Chen Y-L, Hu Y-H, Tsai C-W, et al. Structural evolution during mechanical milling and subsequent annealing of Cu–Ni–Al–Co–Cr–Fe–Ti alloys. Mater Chem Phys. 2009 Dec;118(2–3):354–361.
- Baldenebro-Lopez FJ, Herrera-Ramírez JM, Arredondo-Rea SP, et al. Simultaneous effect of mechanical alloying and arc-melting processes in the microstructure and hardness of an AlCoFeMoNiTi high-entropy alloy. J Alloys Compd. 2015;643:S250–S255.
- Sriharitha R, Murty BS, Kottada RS. Phase formation in mechanically alloyed AlxCoCrCuFeNi (x = 0.45, 1, 2.5, 5 mol) high entropy alloys. Intermetallics. 2013;32:119–126.
- Fu Z, Chen W, Wen H, et al. Effects of Co and sintering method on microstructure and mechanical behavior of a high-entropy Al0.6NiFeCrCo alloy prepared by powder metallurgy. J Alloys Compd. 2015 Oct;646:175–182.
- Chen W, Fu Z, Fang S, et al. Processing, microstructure and properties of Al0.6CoNiFeTi0.4 high entropy alloy with nanoscale twins. Mater Sci Eng A. 2013 Mar;565:439–444.
- Zhu G, Liu Y, Ye J. Early high-temperature oxidation behavior of Ti(C,N)-based cermets with multi-component AlCoCrFeNi high-entropy alloy binder. Int J Refract Met Hard Mater. 2014;44:35–41.
- Ji W, Zhang J, Wang W, et al. Fabrication and properties of TiB2-based cermets by spark plasma sintering with CoCrFeNiTiAl high-entropy alloy as sintering aid. J Eur Ceram Soc. 2015 Mar;35(3):879–886.
- Veronesi P, Rosa R, Colombini E, et al. Microwave-Assisted Preparation of high entropy alloys. Technologies. 2015 Oct;3(4):182–197.
- Fu Z, Chen W, Wen H, et al. Microstructure and strengthening mechanisms in an FCC structured single-phase nanocrystalline Co25Ni25Fe25Al7.5Cu17.5 high-entropy alloy. Acta Mater. 2016 Apr;107:59–71.
- Shiratori H, Fujieda T, Yamanaka K, et al. Relationship between the microstructure and mechanical properties of an equiatomic AlCoCrFeNi high-entropy alloy fabricated by selective electron beam melting. Mater Sci Eng A. 2016;656:39–46.
- Qiu X, Huang C, Wu M, et al. Structure and properties of AlCrFeNiCuTi six principal elements equimolar alloy. J Alloys Compd. 2016 Feb;658:1–5.
- Zhang KB, Fu ZY, Zhang JY, et al. Microstructure and mechanical properties of CoCrFeNiTiAlx high-entropy alloys. Mater Sci Eng A. 2009 May;508(1–2):214–219.
- Raphel A, Kumaran S, Kumar KV, et al. Oxidation and corrosion resistance of AlCoCrFeTiHigh entropy alloy. Mater Today Proc. 2017 Jan;4(2):195–202.
- Wang P, Cheng X, Cai H, et al. Influence of increasing Al concentration on phase, microstructure and mechanical behaviors of Ni1.5CoFeCu1−xAlxV0.5 high entropy alloys. Mater Sci Eng A. 2017 Dec;708:523–536.
- Prasad H, Singh S, Panigrahi BB. Mechanical activated synthesis of alumina dispersed FeNiCoCrAlMn high entropy alloy. J Alloys Compd. 2017;692:720–726.
- Li J, Craeghs W, Jing C, et al. Microstructure and physical performance of laser-induction nanocrystals modified high-entropy alloy composites on titanium alloy. Mater Des. 2017 Mar;117:363–370.
- Mohanty S, Maity TN, Mukhopadhyay S, et al. Powder metallurgical processing of equiatomic AlCoCrFeNi high entropy alloy: microstructure and mechanical properties. Mater Sci Eng A. 2017 Jan;679:299–313.
- Yang C-C, Hang Chau JL, Weng C-J, et al. Preparation of high-entropy AlCoCrCuFeNiSi alloy powders by gas atomization process. Mater Chem Phys. 2017 Dec;202:151–158.
- Ganji RS, Sai Karthik P, Bhanu Sankara Rao K, et al. Strengthening mechanisms in equiatomic ultrafine grained AlCoCrCuFeNi high-entropy alloy studied by micro- and nanoindentation methods. Acta Mater. 2017 Feb;125:58–68.
- Riva S, Tudball A, Mehraban S, et al. A novel high-entropy alloy-based composite material. J Alloys Compd. 2018 Jan;730:544–551.
- Yang S, Pi J, Yang W, et al. Deformation twinning structure and interface in a FCC-based Al0.3FeNiCo1.2CrCu high-entropy alloy matrix composites. Mater Lett. 2018 Mar;214:50–52.
- Yang S, Yan X, Yang K, et al. Effect of the addition of nano-Al2O3 on the microstructure and mechanical properties of twinned Al0.4FeCrCoNi1.2Ti0.3 alloys. Vacuum. 2016 Sep;131:69–72.
- Zhang KB, Fu ZY, Zhang JY, et al. Characterization of nanocrystalline CoCrFeNiTiAl high-entropy solid solution processed by mechanical alloying. J Alloys Compd. 2010;495(1):33–38.
- Joseph J, Hodgson P, Jarvis T, et al. Effect of hot isostatic pressing on the microstructure and mechanical properties of additive manufactured AlxCoCrFeNi high entropy alloys. Mater Sci Eng A. 2018 Aug;733:59–70.
- Luo W, Liu Y, Luo Y, et al. Fabrication and characterization of WC-AlCoCrCuFeNi high-entropy alloy composites by spark plasma sintering. J Alloys Compd. 2018 Jul;754:163–170.
- Erdogan A, Yener T, Zeytin S. Fast production of high entropy alloys (CoCrFeNiAlxTiy) by electric current activated sintering system. Vacuum. 2018 Sep;155:64–72.
- Zhou PL, Xiao DH, Zhou PF, et al. Microstructure and properties of ultrafine grained AlCrFeCoNi/WC cemented carbides. Ceram Int. 2018;44(14):17160–17166.
- Colombini E, Rosa R, Trombi L, et al. High entropy alloys obtained by field assisted powder metallurgy route: SPS and microwave heating. Mater Chem Phys. 2018 May;210:78–86.
- Yusenko KV, Riva S, Crichton WA, et al. High-pressure high-temperature tailoring of high entropy alloys for extreme environments. J Alloys Compd. 2018 Mar;738:491–500.
- Wang C, Ji W, Fu Z. Mechanical alloying and spark plasma sintering of CoCrFeNiMnAl high-entropy alloy. Adv Powder Technol. 2014;25(4):1334–1338.
- Sriharitha R, Murty BS, Kottada RS. Alloying, thermal stability and strengthening in spark plasma sintered AlxCoCrCuFeNi high entropy alloys. J Alloys Compd. 2014;583:419–426.
- Kunce I, Polanski M, Karczewski K, et al. Microstructural characterisation of high-entropy alloy AlCoCrFeNi fabricated by laser engineered net shaping. J Alloys Compd. 2015 Nov;648:751–758.
- Gómez-Esparza CD, Baldenebro-López F, González-Rodelas L, et al. Series of nanocrystalline NiCoAlFe(Cr, Cu, Mo, Ti) high-entropy alloys produced by mechanical alloying. Mater Res. 2016 Aug;19(suppl 1):39–46.
- Gómez-Esparza CD, Ochoa-Gamboa RA, Estrada-Guel I, et al. Microstructure of NiCoAlFeCuCr multi-component systems synthesized by mechanical alloying. J Alloys Compd. 2011 Jun;509:S279–S283.
- Chen J, Niu P, Wei T, et al. Fabrication and mechanical properties of AlCoNiCrFe high-entropy alloy particle reinforced Cu matrix composites. J Alloys Compd. 2015;649:630–634.
- Brif Y, Thomas M, Todd I. The use of high-entropy alloys in additive manufacturing. Scr Mater. 2015 Apr;99:93–96.
- Fujieda T, Shiratori H, Kuwabara K, et al. First demonstration of promising selective electron beam melting method for utilizing high-entropy alloys as engineering materials. Mater Lett. 2015;159:12–15.
- Joseph J, Jarvis T, Wu X, et al. Comparative study of the microstructures and mechanical properties of direct laser fabricated and arc-melted AlxCoCrFeNi high entropy alloys. Mater Sci Eng A. 2015;633:184–193.
- Zhang A, Han J, Meng J, et al. Rapid preparation of AlCoCrFeNi high entropy alloy by spark plasma sintering from elemental powder mixture; 2016.
- Tan Z, Wang L, Xue Y, et al. High-entropy alloy particle reinforced Al-based amorphous alloy composite with ultrahigh strength prepared by spark plasma sintering. Mater Des. 2016 Nov;109:219–226.
- Joseph J, Stanford N, Hodgson P, et al. Tension/compression asymmetry in additive manufactured face centered cubic high entropy alloy. Scr Mater. 2017 Mar;129:30–34.
- Veronesi P, Colombini E, Rosa R, et al. Microwave processing of high entropy alloys: A powder metallurgy approach. Chem Eng Process Process Intensif. 2017 Dec;122:397–403.
- Wang R, Zhang K, Davies C, et al. Evolution of microstructure, mechanical and corrosion properties of AlCoCrFeNi high-entropy alloy prepared by direct laser fabrication. J Alloys Compd. 2017;694:971–981.
- Nam S, Kim MJ, Hwang JY, et al. Strengthening of Al0.15CoCrCuFeNiTix–C (x = 0, 1, 2) high-entropy alloys by grain refinement and using nanoscale carbides via powder metallurgical route. J Alloys Compd. 2018 Sep;762:29–37.
- Praveen S, Murty BS, Kottada RS. Alloying behavior in multi-component AlCoCrCuFe and NiCoCrCuFe high entropy alloys. Mater Sci Eng A. 2012;534:83–89.
- Lu T, Scudino S, Chen W, et al. The influence of nanocrystalline CoNiFeAl0.4Ti0.6Cr0.5 high-entropy alloy particles addition on microstructure and mechanical properties of SiCp/7075Al composites. Mater Sci Eng A. 2018;726:126–136.
- Pohan RM, Gwalani B, Lee J, et al. Microstructures and mechanical properties of mechanically alloyed and spark plasma sintered Al0.3CoCrFeMnNi high entropy alloy. Mater Chem Phys. 2018 May;210:62–70.
- Kuwabara K, Shiratori H, Fujieda T, et al. Mechanical and corrosion properties of AlCoCrFeNi high-entropy alloy fabricated with Selective electron beam melting. Addit Manuf. 2018;23:264–271.
- Fu Z, Chen W, Jiang Z, et al. Influence of Cr removal on the microstructure and mechanical behaviour of a high-entropy Al0.8Ti0.2CoNiFeCr alloy fabricated by powder metallurgy. Powder Metall. 2018;61(2):106–114.
- Colombini E, Lassinantti Gualtieri M, Rosa R, et al. SPS-assisted Synthesis of SICp reinforced high entropy alloys: reactivity of SIC and effects of pre-mechanical alloying and post-annealing treatment. Powder Metall. 2018;61(1):64–72.
- Varalakshmi S, Kamaraj M, Murty BS. Processing and properties of nanocrystalline CuNiCoZnAlTi high entropy alloys by mechanical alloying. Mater Sci Eng A. 2010 Feb;527(4):1027–1030.
- Chen Y-L, Hu Y-H, Hsieh C-A, et al. Competition between elements during mechanical alloying in an octonary multi-principal-element alloy system. J Alloys Compd. 2009 Jul;481(1–2):768–775.
- Varalakshmi S, Kamaraj M, Murty BS. Formation and stability of equiatomic and nonequiatomic nanocrystalline CuNiCoZnAlTi high-entropy alloys by mechanical alloying. Metall Mater Trans A. 2010 Oct;41(10):2703–2709.
- Fu Z, Chen W, Wen H, et al. Microstructure and mechanical behavior of a novel Co20Ni20Fe20Al20Ti20 alloy fabricated by mechanical alloying and spark plasma sintering. Mater Sci Eng A. 2015;644:10–16.
- Wang P, Cai H, Cheng X. Effect of Ni/Cr ratio on phase, microstructure and mechanical properties of NixCoCuFeCr2−x (x = 1.0, 1.2, 1.5, 1.8 mol) high entropy alloys. J Alloys Compd. 2016 Mar;662:20–31.
- Tariq NH, Naeem M, Hasan BA, et al. Effect of W and Zr on structural, thermal and magnetic properties of AlCoCrCuFeNi high entropy alloy. J Alloys Compd. 2013 Apr;556:79–85.
- Yang S, Zhang Y, Yan X, et al. Deformation twins and interface characteristics of nano-Al2O3 reinforced Al0.4FeCrCo1.5NiTi0.3 high entropy alloy composites. Mater Chem Phys. 2018 May;210:240–244.
- Chen W, Fu Z, Fang S, et al. Alloying behavior, microstructure and mechanical properties in a FeNiCrCo0.3Al0.7 high entropy alloy. Mater Des. 2013 Oct;51:854–860.
- Fu Z, Chen W, Fang S, et al. Alloying behavior and deformation twinning in a CoNiFeCrAl0.6Ti0.4 high entropy alloy processed by spark plasma sintering. J Alloys Compd. 2013 Mar;553:316–323.
- Varalakshmi S, Appa Rao G, Kamaraj M, et al. Hot consolidation and mechanical properties of nanocrystalline equiatomic AlFeTiCrZnCu high entropy alloy after mechanical alloying. J Mater Sci. 2010 Oct;45(19):5158–5163.
- Ge W, Wu B, Wang S, et al. Characterization and properties of CuZrAlTiNi high entropy alloy coating obtained by mechanical alloying and vacuum hot pressing sintering. Adv Powder Technol. 2017 Oct;28(10):2556–2563.
- Mohanty S, Gurao NP, Biswas K. Sinter ageing of equiatomic Al20Co20Cu20Zn20Ni20 high entropy alloy via mechanical alloying. Mater Sci Eng A. 2014;617:211–218.
- Ge W, Wang Y, Shang C, et al. Microstructures and properties of equiatomic CuZr and CuZrAlTiNi bulk alloys fabricated by mechanical alloying and spark plasma sintering. J Mater Sci. 2017 May;52(10):5726–5737.
- Ji W, Wang W, Wang H, et al. Alloying behavior and novel properties of CoCrFeNiMn high-entropy alloy fabricated by mechanical alloying and spark plasma sintering. Intermetallics. 2015 Jan;56:24–27.
- Yu PF, Zhang LJ, Cheng H, et al. The high-entropy alloys with high hardness and soft magnetic property prepared by mechanical alloying and high-pressure sintering. Intermetallics. 2016;70:82–87.
- Wang B, Fu A, Huang X, et al. Mechanical properties and microstructure of the CoCrFeMnNi high entropy alloy under high strain rate compression. J Mater Eng Perform. 2016 Jul;25(7):2985–2992.
- Liu B, Wang J, Liu Y, et al. Microstructure and mechanical properties of equimolar FeCoCrNi high entropy alloy prepared via powder extrusion. Intermetallics. 2016;75:25–30.
- Wang J, Liu Y, Liu B, et al. Flow behavior and microstructures of powder metallurgical CrFeCoNiMo0.2 high entropy alloy during high temperature deformation. Mater Sci Eng A. 2017 Mar;689:233–242.
- Rogal Ł, Kalita D, Litynska-Dobrzynska L. Cocrfemnni high entropy alloy matrix nanocomposite with addition of Al2O3. Intermetallics. 2017;86:104–109.
- Eißmann N, Klöden B, Weißgärber T, et al. High-entropy alloy CoCrFeMnNi produced by powder metallurgy. Powder Metall. 2017;60(3):184–197.
- Shang C, Axinte E, Sun J, et al. Cocrfeni(W1−xMox) high-entropy alloy coatings with excellent mechanical properties and corrosion resistance prepared by mechanical alloying and hot pressing sintering. Mater Des. 2017 Mar;117:193–202.
- Haase C, Tang F, Wilms MB, et al. Combining thermodynamic modeling and 3D printing of elemental powder blends for high-throughput investigation of high-entropy alloys – Towards rapid alloy screening and design. Mater Sci Eng A. 2017;688:180–189.
- Yim D, Kim W, Praveen S, et al. Shock wave compaction and sintering of mechanically alloyed CoCrFeMnNi high-entropy alloy powders. Mater Sci Eng A. 2017 Dec;708:291–300.
- Hadraba H, Chlup Z, Dlouhy A, et al. Oxide dispersion strengthened CoCrFeNiMn high-entropy alloy. Mater Sci Eng A. 2017 Mar;689:252–256.
- Rogal Ł, Kalita D, Tarasek A, et al. Effect of SiC nano-particles on microstructure and mechanical properties of the CoCrFeMnNi high entropy alloy. J Alloys Compd. 2017;708:344–352.
- Liu Y, Wang J, Fang Q, et al. Preparation of superfine-grained high entropy alloy by spark plasma sintering gas atomized powder. Intermetallics. 2016;68:16–22.
- Sathiyamoorthi P, Basu J, Kashyap S, et al. Thermal stability and grain boundary strengthening in ultrafine-grained CoCrFeNi high entropy alloy composite. Mater Des. 2017 Nov;134:426–433.
- Sun C, Li P, Xi S, et al. A new type of high entropy alloy composite Fe18Ni23Co25Cr21Mo8WNb3C2 prepared by mechanical alloying and hot pressing sintering. Mater Sci Eng A. 2018;728:144–150.
- Zhang A, Han J, Su B, et al. A promising new high temperature self-lubricating material: CoCrFeNiS0.5 high entropy alloy. Mater Sci Eng A. 2018;731:36–43.
- Yim D, Jang MJ, Bae JW, et al. Compaction behavior of water-atomized CoCrFeMnNi high-entropy alloy powders. Mater Chem Phys. 2018 May;210:95–102.
- Praveen S, Basu J, Kashyap S, et al. Exceptional resistance to grain growth in nanocrystalline CoCrFeNi high entropy alloy at high homologous temperatures. J Alloys Compd. 2016 Mar;662:361–367.
- Dobeš F, Hadraba H, Chlup Z, et al. Compressive creep behavior of an oxide-dispersion-strengthened CoCrFeMnNi high-entropy alloy. Mater Sci Eng A. 2018;732:99–104.
- Mane RB, Panigrahi BB. Effect of alloying order on non-isothermal sintering kinetics of mechanically alloyed high entropy alloy powders. Mater Lett. 2018 Apr;217:131–134.
- Cheng H, Chen W, Liu X, et al. Effect of Ti and C additions on the microstructure and mechanical properties of the FeCoCrNiMn high-entropy alloy. Mater Sci Eng A. 2018 Mar;719:192–198.
- Alcalá MD, Real C, Fombella I, et al. Effects of milling time, sintering temperature, Al content on the chemical nature, microhardness and microstructure of mechanochemically synthesized FeCoNiCrMn high entropy alloy. J Alloys Compd. 2018;749:834–843.
- Xie Y, Cheng H, Tang Q, et al. Effects of N addition on microstructure and mechanical properties of CoCrFeNiMn high entropy alloy produced by mechanical alloying and vacuum hot pressing sintering. Intermetallics. 2018 Feb;93:228–234.
- Zhang A, Han J, Su B, et al. Microstructure, mechanical properties and tribological performance of CoCrFeNi high entropy alloy matrix self-lubricating composite. Mater Des. 2017;114:253–263.
- Velo IL, Gotor FJ, Alcalá MD, et al. Fabrication and characterization of WC-HEA cemented carbide based on the CoCrFeNiMn high entropy alloy. J Alloys Compd. 2018;746:1–8.
- Zhu ZG, Nguyen QB, Ng FL, et al. Hierarchical microstructure and strengthening mechanisms of a CoCrFeNiMn high entropy alloy additively manufactured by selective laser melting. Scr Mater. 2018 Sep;154:20–24.
- Zhou R, Chen G, Liu B, et al. Microstructures and wear behaviour of (FeCoCrNi)1-x(WC)x high entropy alloy composites. Int J Refract Met Hard Mater. 2018 Sep;75:56–62.
- Szklarz Z, Lekki J, Bobrowski P, et al. The effect of SiC nanoparticles addition on the electrochemical response of mechanically alloyed CoCrFeMnNi high entropy alloy. Mater Chem Phys. 2018 Aug;215:385–392.
- Wang B, Huang X, Fu A, et al. Serration behavior and microstructure of high entropy alloy CoCrFeMnNi prepared by powder metallurgy. Mater Sci Eng A. 2018 May;726:37–44.
- Zhou R, Liu Y, Zhou C, et al. Microstructures and mechanical properties of C-containing FeCoCrNi high-entropy alloy fabricated by selective laser melting. Intermetallics. 2018;94:165–171.
- Li R, Niu P, Yuan T, et al. Selective laser melting of an equiatomic CoCrFeMnNi high-entropy alloy: Processability, non-equilibrium microstructure and mechanical property. J Alloys Compd. 2018;746:125–134.
- Guo J, Goh M, Zhu Z, et al. On the machining of selective laser melting CoCrFeMnNi high-entropy alloy. Mater Des. 2018;153:211–220.
- Piglione A, Dovgyy B, Liu C, et al. Printability and microstructure of the CoCrFeMnNi high-entropy alloy fabricated by laser powder bed fusion. Mater Lett. 2018 Aug;224:22–25.
- Zhang M, Zhang W, Liu Y, et al. Fecocrnimo high-entropy alloys prepared by powder metallurgy processing for diamond tool applications. Powder Metall. 2018;61(2):123–130.
- Joo S-H, Kato H, Jang MJ, et al. Structure and properties of ultrafine-grained CoCrFeMnNi high-entropy alloys produced by mechanical alloying and spark plasma sintering. J Alloys Compd. 2017 Mar;698:591–604.
- Yang Q, Tang Y, Wen Y, et al. Microstructures and properties of CoCrCuFeNiMox high-entropy alloys fabricated by mechanical alloying and spark plasma sintering. Powder Metall. 2018;61(2):115–122.
- Mane RB, Panigrahi B. Sintering mechanism of CoCrFeMnNi high-entropy alloy powders. Powder Metall. 2018;61(2):131–138.
- Praveen S, Murty BS, Kottada RS. Phase Evolution and densification behavior of nanocrystalline multicomponent high entropy alloys during spark plasma sintering. JOM. 2013;65(12):1797–1804.
- He Z-W, Wang M-Z, Hao X-L, et al. Novel cemented carbide produced with TiN0.3 and high-entropy alloys. Rare Met. 2017 Jun;36(6):494–500.
- Praveen S, Anupam A, Tilak R, et al. Phase evolution and thermal stability of AlCoCrFe high entropy alloy with carbon as unsolicited addition from milling media. Mater Chem Phys. 2018;210:57–61.
- Qiu Z, Yao C, Feng K, et al. Cryogenic deformation mechanism of CrMnFeCoNi high-entropy alloy fabricated by laser additive manufacturing process. Int J Light Mater Manuf. 2018 Mar;1(1):33–39.
- de la Obra AG, Avilés MA, Torres Y, et al. A new family of cermets: Chemically complex but microstructurally simple. Int J Refract Met Hard Mater. 2017 Feb;63:17–25.
- Liu WH, Lu ZP, He JY, et al. Ductile CoCrFeNiMox high entropy alloys strengthened by hard intermetallic phases. Acta Mater. 2016 Sep;116:332–342.
- Wu B, Chen W, Jiang Z, et al. Influence of Ti addition on microstructure and mechanical behavior of a FCC-based Fe30Ni30Co30Mn10 alloy. Mater Sci Eng A. 2016 Oct;676:492–500.
- Fu Z, Chen W, Xiao H, et al. Fabrication and properties of nanocrystalline Co0.5FeNiCrTi0.5 high entropy alloy by MA–SPS technique. Mater Des. 2013 Feb;44:535–539.
- Lin C-M, Tsai C-W, Huang S-M, et al. New TiC/Co1.5CrFeNi1.5Ti0.5 cermet with slow TiC coarsening during sintering. JOM. 2014 Oct;66(10):2050–2056.
- Kunce I, Polanski M, Bystrzycki J. Structure and hydrogen storage properties of a high entropy ZrTiVCrFeNi alloy synthesized using laser Engineered Net shaping (LENS). Int J Hydrogen Energy. 2013;38(27):12180–12189.
- Moravcik I, Cizek J, Zapletal J, et al. Microstructure and mechanical properties of Ni1,5Co1,5CrFeTi0,5 high entropy alloy fabricated by mechanical alloying and spark plasma sintering. Mater Des. 2017 Apr;119:141–150.
- Cai Z, Jin G, Cui X, et al. Experimental and simulated data about microstructure and phase composition of a NiCrCoTiV high-entropy alloy prepared by vacuum hot-pressing sintering. Vacuum. 2016 Feb;124:5–10.
- Liu B, Wang J, Chen J, et al. Ultra-high strength TiC/refractory high-entropy-alloy composite prepared by powder metallurgy. JOM. 2017 Apr;69(4):651–656.
- Zepon G, Leiva DR, Strozi RB, et al. Hydrogen-induced phase transition of MgZrTiFe0.5Co0.5Ni0.5 high entropy alloy. Int J Hydrogen Energy. 2018 Jan;43(3):1702–1708.
- Fu Z, MacDonald BE, Zhang D, et al. Fcc nanostructured TiFeCoNi alloy with multi-scale grains and enhanced plasticity. Scr Mater. 2018 Jan;143:108–112.
- Holmström E, Lizárraga R, Linder D, et al. High entropy alloys: Substituting for cobalt in cutting edge technology. Appl Mater Today. 2018 Sep;12:322–329.
- Cai Z, Cui X, Liu Z, et al. Microstructure and wear resistance of laser cladded Ni-Cr-Co-Ti-V high-entropy alloy coating after laser remelting processing. Opt Laser Technol. 2018;99:276–281.
- Prieto E, De Oro Calderon R, Konegger T, et al. Processing of a new high entropy alloy: AlCrFeMoNiTi. Powder Metall. 2018;61(3):258–265.
- Koundinya NTBN, Sajith Babu C, Sivaprasad K, et al. Phase Evolution and thermal analysis of nanocrystalline AlCrCuFeNiZn high entropy alloy produced by mechanical alloying. J Mater Eng Perform. 2013 Oct;22(10):3077–3084.
- Maulik O, Kumar D, Kumar S, et al. Structural evolution of spark plasma sintered AlFeCuCrMgx (x = 0, 0.5, 1, 1.7) high entropy alloys. Intermetallics. 2016;77:46–56.
- Mridha S, Samal S, Khan PY, et al. Processing and consolidation of nanocrystalline Cu-Zn-Ti-Fe-Cr high-entropy alloys via mechanical alloying. Metall Mater Trans A. 2013 Oct;44(10):4532–4541.
- Sharma AS, Yadav S, Biswas K, et al. High-entropy alloys and metallic nanocomposites: processing challenges, microstructure development and property enhancement. Mater Sci Eng R Reports. 2018 Sep;131:1–42.
- Yadav S, Kumar A, Biswas K. Wear behavior of high entropy alloys containing soft dispersoids (Pb, Bi). Mater Chem Phys. 2018 May;210:222–232.
- Yadav S, Sarkar S, Aggarwal A, et al. Wear and mechanical properties of novel (CuCrFeTiZn)100-xPbx high entropy alloy composite via mechanical alloying and spark plasma sintering. Wear. 2018;410:93–109.
- Kumar D, Maulik O, Kumar S, et al. Phase and thermal study of equiatomic AlCuCrFeMnW high entropy alloy processed via spark plasma sintering. Mater Chem Phys. 2018;210:71–77.
- Murali M, Babu SPK, Majhi J, et al. Processing and characterisation of nano crystalline AlCoCrCuFeTix high-entropy alloy. Powder Metall. 2018;61(2):139–148.
- Dobbelstein H, Thiele M, Gurevich EL, et al. Direct metal deposition of refractory high entropy alloy MoNbTaW. Phys Procedia. 2016 Jan;83:624–633.
- Kang B, Lee J, Ryu HJ, et al. Ultra-high strength WNbMoTaV high-entropy alloys with fine grain structure fabricated by powder metallurgical process. Mater Sci Eng A. 2018;712:616–624.
- Wang P, Cai H, Zhou S, et al. Processing, microstructure and properties of Ni1.5CoCuFeCr0.5−xVx high entropy alloys with carbon introduced from process control agent. J Alloys Compd. 2017 Feb;695:462–475.
- Waseem OA, Lee J, Lee HM, et al. The effect of Ti on the sintering and mechanical properties of refractory high-entropy alloy TixWTaVCr fabricated via spark plasma sintering for fusion plasma-facing materials. Mater Chem Phys. 2018;210:87–94.
- Waseem OA, Ryu HJ. Powder metallurgy processing of a WxTaTiVCr high-entropy alloy and its derivative alloys for fusion material applications. Sci Rep. 2017;7(1):1926.
- Linder D, Holmström E, Norgren S. High entropy alloy binders in gradient sintered hardmetal. Int J Refract Met Hard Mater. 2018 Feb;71:217–220.
- Cao Y, Liu Y, Liu B, et al. Precipitation behavior during hot deformation of powder metallurgy Ti-Nb-Ta-Zr-Al high entropy alloys. Intermetallics. 2018 Sep;100:95–103.
- Kunce I, Polanski M, Bystrzycki J. Microstructure and hydrogen storage properties of a TiZrNbMoV high entropy alloy synthesized using Laser Engineered Net Shaping (LENS). Int J Hydrogen Energy. 2014 Jun;39(18):9904–9910.
- Raza A, Kang B, Lee J, et al. Transition in microstructural and mechanical behavior by reduction of sigma-forming element content in a novel high entropy alloy. Mater Des. 2018 May;145:11–19.
- Song R, Wei L, Yang C, et al. Phase formation and strengthening mechanisms in a dual-phase nanocrystalline CrMnFeVTi high-entropy alloy with ultrahigh hardness. J Alloys Compd. 2018 May;744:552–560.
- Senkov O, Isheim D, Seidman D, et al. Development of a refractory high entropy Superalloy. Entropy. 2016 Mar;18(3):102.
- Zhang Y, Zhou YJ, Lin JP, et al. Solid-solution phase formation rules for multi-component alloys. Adv Eng Mater. 2008;10(6):534–538.
- Guo S, Liu CT. Phase stability in high entropy alloys: formation of solid-solution phase or amorphous phase. Prog Nat Sci Mater Int. 2011 Dec;21(6):433–446.
- Tian F, Varga LK, Chen N, et al. Empirical design of single phase high-entropy alloys with high hardness. Intermetallics. 2015;58:1–6.
- Guo S, Hu Q, Ng C, et al. More than entropy in high-entropy alloys: forming solid solutions or amorphous phase. Intermetallics. 2013 Oct;41:96–103.
- Guo S, Ng C, Lu J, et al. Effect of valence electron concentration on stability of fcc or bcc phase in high entropy alloys. J Appl Phys. 2011 May;109(10):103505.
- Tsai M-H, Tsai K-Y, Tsai C-W, et al. Criterion for sigma phase formation in Cr- and V-containing high-entropy alloys. Mater Res Lett. 2013;1(4):207–212.
- Górecki K, Zýka J, Malek J, et al. Sintering and heat treatment of Al 15 Ti 5 Co 35 Ni 25 Fe 20 high-entropy alloy. IOP Conf Ser Mater Sci Eng. 2017 Feb;179:012027.
- Qiu X-W, Zhang Y-P, He L, et al. Microstructure and corrosion resistance of AlCrFeCuCo high entropy alloy. J Alloys Compd. 2013;549:195–199.
- Eymann K, Riedl T, Bram A, et al. Consolidation of mechanically alloyed nanocrystalline Cu–Nb–ZrO2 powder by spark plasma sintering. J Alloys Compd. 2012;535:62–69.
- Qiu X-W, Liu C-G. Microstructure and properties of Al2CrFeCoCuTiNix high-entropy alloys prepared by laser cladding. J Alloys Compd. 2013 Mar;553:216–220.
- Munir ZA, Anselmi-Tamburini U, Ohyanagi M. The effect of electric field and pressure on the synthesis and consolidation of materials: A review of the spark plasma sintering method. J Mater Sci. 2006 Feb;41(3):763–777.
- Gali A, George EP. Tensile properties of high- and medium-entropy alloys. Intermetallics. 2013 Aug;39:74–78.
- Curtze S, Kuokkala V-T. Dependence of tensile deformation behavior of TWIP steels on stacking fault energy, temperature and strain rate. Acta Mater. 2010 Sep;58(15):5129–5141.
- Santos EC, Shiomi M, Osakada K, et al. Rapid manufacturing of metal components by laser forming. Int J Mach Tools Manuf. 2006 Oct;46(12–13):1459–1468.
- Gu DD, Meiners W, Wissenbach K, et al. Laser additive manufacturing of metallic components: materials, processes and mechanisms. Int Mater Rev. 2012;57(3):133–164.
- Frazier WE. Metal additive manufacturing: a review. J Mater Eng Perform. 2014 Jun;23(6):1917–1928.
- Gorsse S, Hutchinson C, Gouné M, et al. Additive manufacturing of metals: a brief review of the characteristic microstructures and properties of steels, Ti-6Al-4V and high-entropy alloys. Sci Technol Adv Mater. 2017;18(1):584–610.
- He Q, Yang Y. On lattice distortion in high entropy alloys. Front Mater. 2018;5:42.
- Otto F, Dlouhý A, Somsen C, et al. The influences of temperature and microstructure on the tensile properties of a CoCrFeMnNi high-entropy alloy. Acta Mater. 2013 Sep;61(15):5743–5755.
- Cai B, Liu B, Kabra S, et al. Deformation mechanisms of Mo alloyed FeCoCrNi high entropy alloy: In situ neutron diffraction. Acta Mater. 2017 Apr;127:471–480.
- Tsai SKCC-W, Chen Y-L, Tsai M-H, et al. Deformation and annealing behaviors of high-entropy alloy Al0.5CoCrCuFeNi. J Alloys Compd. 2009 Nov;486(1–2):427–435.
- El-Danaf E, Kalidindi SR, Doherty RD. Influence of grain size and stacking-fault energy on deformation twinning in fcc metals. Metall Mater Trans A. 1999 May;30(5):1223–1233.
- Zhang KLY, Tao NR. Effect of stacking-fault energy on deformation twin thickness in Cu–Al alloys. Scr Mater. 2009 Feb;60(4):211–213.
- Ma E. Eight routes to improve the tensile ductility of bulk nanostructured metals and alloys. JOM. 2006;58(4):49–53.
- Praveen S, Kim HS. High-entropy alloys: potential candidates for high-temperature applications – an overview. Adv Eng Mater. 2018; 20(1):1700645.
- Lu Y, Dong Y, Guo S, et al. A promising new class of high-temperature alloys: eutectic high-entropy alloys. Sci Rep. 2014 Aug;4:6200.
- Chauhan A, Litvinov D, Aktaa J. High temperature tensile properties and fracture characteristics of bimodal 12Cr-ODS steel. J Nucl Mater. 2016 Jan;468:1–8.
- Zhou YJ, Zhang Y, Wang YL, et al. Solid solution alloys of AlCoCrFeNiTix with excellent room-temperature mechanical properties. Appl Phys Lett. 2007 Apr;90(18):181904.
- Dong Y, Zhou K, Lu Y, et al. Effect of vanadium addition on the microstructure and properties of AlCoCrFeNi high entropy alloy. Mater Des. 2014 May;57:67–72.
- Salishchev GA, Tikhonovsky MA, Shaysultanov DG, et al. Effect of Mn and V on structure and mechanical properties of high-entropy alloys based on CoCrFeNi system. J Alloys Compd. 2014 Apr;591:11–21.
- Stepanov ND, Shaysultanov DG, Salishchev GA, et al. Structure and mechanical properties of a light-weight AlNbTiV high entropy alloy. Mater Lett. 2015 Mar;142:153–155.
- Zhou YJ, Zhang Y, Wang YL, et al. Microstructure and compressive properties of multicomponent Alx(TiVCrMnFeCoNiCu)100−x high-entropy alloys. Mater Sci Eng A. 2007 Apr;454–455:260–265.
- Wang XF, Zhang Y, Qiao Y, et al. Novel microstructure and properties of multicomponent CoCrCuFeNiTix alloys. Intermetallics. 2007 Mar;15(3):357–362.
- Wang YP, Li BS, Ren MX, et al. Microstructure and compressive properties of AlCrFeCoNi high entropy alloy. Mater Sci Eng A. 2008 Sep;491(1–2):154–158.
- Li BS, Wang YP, Ren MX, et al. Effects of Mn, Ti and V on the microstructure and properties of AlCrFeCoNiCu high entropy alloy. Mater Sci Eng A. 2008 Dec;498(1–2):482–486.
- Senkov ON, Wilks GB, Scott JM, et al. Mechanical properties of Nb25Mo25Ta25W25 and V20Nb20Mo20Ta20W20 refractory high entropy alloys. Intermetallics. 2011 May;19(5):698–706.
- Ma SG, Zhang Y. Effect of Nb addition on the microstructure and properties of AlCoCrFeNi high-entropy alloy. Mater Sci Eng A. 2012 Jan;532:480–486.
- Dong Y, Lu Y, Kong J, et al. Microstructure and mechanical properties of multi-component AlCrFeNiMox high-entropy alloys. J Alloys Compd. 2013 Oct;573:96–101.
- Senkov ON, Woodward C, Miracle DB. Microstructure and properties of aluminum-containing refractory high-entropy alloys. JOM. 2014 Oct;66(10):2030–2042.
- Yao MJ, Pradeep KG, Tasan CC, et al. A novel, single phase, non-equiatomic FeMnNiCoCr high-entropy alloy with exceptional phase stability and tensile ductility. Scr Mater. 2014 Feb;72–73:5–8.
- Kuznetsov AV, Shaysultanov DG, Stepanov ND, et al. Tensile properties of an AlCrCuNiFeCo high-entropy alloy in as-cast and wrought conditions. Mater Sci Eng A. 2012 Jan;533:107–118.
- Tang Z, Senkov ON, Parish CM, et al. Tensile ductility of an AlCoCrFeNi multi-phase high-entropy alloy through hot isostatic pressing (HIP) and homogenization. Mater Sci Eng A. 2015 Oct;647:229–240.
- Wani IS, Bhattacharjee T, Sheikh S, et al. Ultrafine-Grained AlCoCrFeNi2.1 Eutectic high-entropy alloy. Mater Res Lett. 2016;4(3):174–179.
- Dong Y, Gao X, Lu Y, et al. A multi-component AlCrFe2Ni2 alloy with excellent mechanical properties. Mater Lett. 2016 Apr;169:62–64.
- Liu WH, He JY, Huang HL, et al. Effects of Nb additions on the microstructure and mechanical property of CoCrFeNi high-entropy alloys. Intermetallics. 2015 May;60:1–8.
- Ma SG, Zhang SF, Qiao JW, et al. Superior high tensile elongation of a single-crystal CoCrFeNiAl0.3 high-entropy alloy by Bridgman solidification. Intermetallics. 2014 Nov;54:104–109.
- He JY, Liu WH, Wang H, et al. Effects of Al addition on structural evolution and tensile properties of the FeCoNiCrMn high-entropy alloy system. Acta Mater. 2014 Jan;62:105–113.
- Shun T-T, Du Y-C. Microstructure and tensile behaviors of FCC Al0.3CoCrFeNi high entropy alloy. J Alloys Compd. 2009 Jun;479(1–2):157–160.
- Zuo T, Ren S, Liaw PK, et al. Processing effects on the magnetic and mechanical properties of FeCoNiAl0.2Si0.2 high entropy alloy. Int J Miner Metall Mater. 2013 Jun;20(6):549–555.
- Wu YD, Cai YH, Wang T, et al. A refractory Hf25Nb25Ti25Zr25 high-entropy alloy with excellent structural stability and tensile properties. Mater Lett. 2014 Sep;130:277–280.
- Tsai C-W, Tsai M-H, Tsai K-Y, et al. Microstructure and tensile properties of Al0.5CoCrCuFeNi alloys produced by simple rolling and annealing. Mater Sci Technol. 2015;31(10):1178–1183.
- Deng Y, Tasan CC, Pradeep KG, et al. Design of a twinning-induced plasticity high entropy alloy. Acta Mater. 2015 Aug;94:124–133.
- Tsai C-W, Tsai M-H, Yeh J-W, et al. Effect of temperature on mechanical properties of Al0.5CoCrCuFeNi wrought alloy. J Alloys Compd. 2010 Feb;490(1–2):160–165.
- Niinomi M. Mechanical properties of biomedical titanium alloys. Mater Sci Eng A. 1998 Mar;243(1–2):231–236.
- Lu JW, et al. Microstructure and mechanical properties of new high strength beta-titanium alloy Ti-1300. Mater Sci Eng A. 2015 Jan;621:182–189.
- Chen J, Young B. Stress–strain curves for stainless steel at elevated temperatures. Eng Struct. 2006 Jan;28(2):229–239.
- Venkatesh V, Rack HJ. Elevated temperature hardening of INCONEL 690. Mech Mater. 1998 Sep;30(1):69–81.
- Schaeublin R, Leguey T, Spätig P, et al. Microstructure and mechanical properties of two ODS ferritic/martensitic steels. J Nucl Mater. 2002 Dec;307–311:778–782.
- Sundar RS, Deevi SC. High-temperature strength and creep resistance of FeAl. Mater Sci Eng A. 2003 Sep;357(1–2):124–133.
- Zhang KB, Fu ZY, Zhang JY, et al. Annealing on the structure and properties evolution of the CoCrFeNiCuAl high-entropy alloy. J Alloys Compd. 2010 Jul;502(2):295–299.
- Shinde SR, Ogale SB, Higgins JS, et al. Co-occurrence of superparamagnetism and Anomalous Hall effect in highly reduced cobalt-doped Rutile TiO2-δ films. Phys Rev Lett. 2004;92(16):166601.
- Aoki M, Noritake T, Ito A, et al. Improvement of cyclic durability of Ti–Cr–V alloy by Fe substitution. Int J Hydrogen Energy. 2011 Sep;36(19):12329–12332.
- Prabakaran RK, Naveen Sait A, Senthilkumar V. Synthesis and characteristization of high entropy alloy (CrMnFeNiCu) reinforced AA6061 aluminium matrix composite. Mech Mech Eng. 2017;21(2):415–424.
- Karthik GM, Panikar S, Ram GDJ, et al. Additive manufacturing of an aluminum matrix composite reinforced with nanocrystalline high-entropy alloy particles. Mater Sci Eng A. 2017;679:193–203.
- Chen C-S, Yang C-C, Chai H-Y, et al. Novel cermet material of WC/multi-element alloy. Int J Refract Met Hard Mater. 2014 Mar;43:200–204.
- Shen TT, Xiao DH, Ou XQ, et al. Effects of LaB6 addition on the microstructure and mechanical properties of ultrafine grained WC–10Co alloys. J Alloys Compd. 2011 Jan;509(4):1236–1243.
- Zhou P-F, Xiao D-H, Yuan T-C. Comparison between ultrafine-grained WC–Co and WC–HEA-cemented carbides. Powder Metall. 2017;60(1):1–6.
- El-Eskandarany MS, Mahday AA, Ahmed H, et al. Synthesis and characterizations of ball-milled nanocrystalline WC and nanocomposite WC–Co powders and subsequent consolidations. J Alloys Compd. 2000 Nov;312(1–2):315–325.
- Sun L, Jia C, Lin C, et al. VC addition prepared ultrafine WC-11Co composites by spark plasma sintering. J Iron Steel Res Int. 2007 Sep;14(5):85–89.
- Cha SI, Hong SH, Kim BK. Spark plasma sintering behavior of nanocrystalline WC–10Co cemented carbide powders. Mater Sci Eng A. 2003 Jun;351(1–2):31–38.
- Kim H-C, Shon I-J, Jeong I-K, et al. Rapid sintering of ultra fine WC and WC-Co hard materials by high-frequency induction heated sintering and their mechanical properties. Met Mater Int. 2007 Feb;13(1):39–45.
- Michalski A, Siemiaszko D. Nanocrystalline cemented carbides sintered by the pulse plasma method. Int J Refract Met Hard Mater. 2007 Mar;25(2):153–158.
- Lin C, Kny E, Yuan G, et al. Microstructure and properties of ultrafine WC–0.6VC–10Co hardmetals densified by pressure-assisted critical liquid phase sintering. J Alloys Compd. 2004 Nov;383(1–2):98–102.
- Sivaprahasam D, Chandrasekar SB, Sundaresan R. Microstructure and mechanical properties of nanocrystalline WC–12Co consolidated by spark plasma sintering. Int J Refract Met Hard Mater. 2007 Mar;25(2):144–152.
- Zhu LH, Huang QW, Zhao HF. Preparation of nanocrystalline WC-10Co-0.8VC by spark plasma sintering. J Mater Sci Lett. 2003;22:1631–1633.
- Fang ZZ, Wang X, Ryu T, et al. Synthesis, sintering, and mechanical properties of nanocrystalline cemented tungsten carbide – A review. Int J Refract Met Hard Mater. 2009;27(2):288–299.
- Moravcik I, Gouvea L, Hornik V, et al. Synergic strengthening by oxide and coherent precipitate dispersions in high-entropy alloy prepared by powder metallurgy. Scr Mater. 2018;157:24–29.
- Andersson J-O, Helander T, Höglund L, et al. Thermo-Calc & DICTRA, computational tools for materials science. Calphad. 2002 Jun;26(2):273–312.
- Saunders N, Guo UKZ, Li X, et al. Using JMatPro to model materials properties and behavior. JOM. 2003;55(12):60–65.
- Sistla HR, Newkirk JW, Frank Liou F. Effect of Al/Ni ratio, heat treatment on phase transformations and microstructure of AlxFeCoCrNi2−x (x = 0.3, 1) high entropy alloys. Mater Des. 2015 Sep;81:113–121.
- Kilmametov A, Kulagin R, Mazilkin A, et al. High-pressure torsion driven mechanical alloying of CoCrFeMnNi high entropy alloy. Scr Mater. 2019;158:29–33.