Abstract
We validated the Rothamsted Carbon (RothC) model against 14 experimental plots subjected to different paddy–upland rotation patterns, including continuous paddy rice cropping and different periods (short, medium, and long term) of upland conversion, in Akita prefecture, northern Japan. We ran the model using the original version of the RothC model, the paddy-soil version of the RothC model (which uses a reduced carbon decomposition rate), and alternating the use of the original and the paddy-soil versions for years with summer upland cropping and paddy rice cropping, respectively. The best simulation pattern was provided by the alternate use of the two versions. In comparison with the original RothC model, alternate use of the two versions showed a smaller root mean square error in all experimental plots and a smaller absolute value of the mean difference in 10 plots with continuous paddy rice cropping and short- and medium-term upland conversion. Alternate use of the two versions had a lower root mean square error and lower absolute value of the mean difference in long-term upland conversion plots compared to the values for the paddy-soil version and showed better performance than the paddy-soil version when straw was applied. The model performance from alternating use of the two versions compared well with the performance reported in previous studies. Alternating the use of the two model versions provides a good technique for simulating changes in soil organic carbon with time, even with a paddy–upland rotation pattern. In addition, this technique will enable a more accurate inventory of Japanese greenhouse gases and help land managers better manage soil fertility in a paddy–upland rotation system.
Introduction
Agricultural practices that lead to an increase in soil organic carbon (SOC) can help to mitigate climate change (Paustian et al. Citation1997; Lal Citation2004; Smith et al. Citation2007). The Kyoto Protocol permits carbon emissions to be offset by demonstrable removal of carbon from the atmosphere; this removal includes improved management of agricultural soils as well as afforestation and reforestation (IPCC Citation2000).
Soil organic matter (SOM) turnover models are effective in simulating changes in the SOC content caused by different agricultural management systems. Most existing models (e.g. review by McGill Citation1996) cannot be successfully applied to paddy soils because they were developed for upland soils. Soil organic matter dynamics in paddy soils differ considerably from that in upland soils because paddy soils are waterlogged, and therefore under anaerobic conditions, during the rice-growing period. These anaerobic conditions slow SOM decomposition, resulting in SOC levels that are higher in paddy fields than in upland soils (Mitsuchi Citation1974; Zhang and He Citation2004). Shirato and Yokozawa (Citation2005) successfully simulated changes in SOC in paddy soils by using a modified Rothamsted Carbon (RothC: Coleman and Jenkinson Citation1996) model, which is one of the leading SOM models. However, the datasets used in their study did not include paddy–upland rotation.
About 18% of paddy fields in Japan are now used for the cultivation of upland crops, not paddy rice, during the summer cropping season (Ministry of Agriculture, Forestry and Fisheries of Japan Citation2010). This is partly a result of surplus rice production caused by the decline in consumption and partly due to the benefits in rotating crop production (e.g. increased nutrient availability, reduced diseases). This rotation of paddy–upland crops, which includes summer upland crop cultivation, is distinguished from double cropping (e.g. summer paddy rice and winter wheat) in which the summer crop is always paddy rice and soils are submerged. Because draining paddy fields can cause SOC depletion (Mitsuchi Citation1974; Zhang and He Citation2004), longer periods in which paddy fields are used in upland crop cultivation without submergence can cause greater decline in SOC. Kitada et al. (Citation1993) reported that two or three years of summer upland crop cultivation followed by two or three years of summer paddy rice cropping would be the best interval to maintain soil fertility.
Neither the modified RothC model for paddy soils (Shirato and Yokozawa Citation2005) nor the original RothC model can suitably simulate changes in SOC from paddy–upland rotation because SOC dynamics differ depending on the relative frequency of paddy and upland soil conditions. Consequently, a method to simulate changes in SOC with time in paddy–upland rotation is needed. Because paddy–upland fields occupy approximately one-fifth of the total paddy field area, the accurate simulation of SOC in these upland areas would increase the accuracy of estimating greenhouse gas emissions at a national scale. The combined use of the two versions of the RothC model (paddy soil and original), for example, might address this problem, and comparing long-term datasets of the two versions and combination of these versions is, therefore, of interest. We found available long-term datasets of paddy–upland rotation experiments, which included several plots with different periods of paddy and upland soil water conditions, from Akita prefecture, northern Japan (Sumida et al. Citation2005). The validation of the models can be realized by using these datasets.
Our objective was to apply the two versions of the RothC model (original and paddy soil), individually and in combination, to datasets from long-term experiments on paddy–upland rotation for validating model performance under rotational systems.
Materials and Methods
Model description
The current version of the RothC model, RothC-26.3, is derived from earlier versions developed by Jenkinson and Rayner (Citation1977). It separates incoming plant residues into decomposable plant materials (DPMs) and resistant plant materials (RPMs), both undergoing decomposition to produce microbial biomass (BIO) and humified organic matter (HUM) and to release carbon dioxide (CO2) (Coleman and Jenkinson Citation1996). The clay content of the soil determines the proportion of carbon allocated to CO2 or to BIO + HUM. Both BIO and HUM undergo further decomposition to produce more CO2, BIO, and HUM. The model also includes a pool of inert organic matter (IOM). Each compartment, except for IOM, undergoes decomposition by first-order kinetics at its own characteristic rate, which is determined using modifiers for soil moisture, temperature, and plant cover. The input parameters include monthly average air temperature, monthly precipitation, monthly open-pan evaporation, soil clay content, monthly carbon input from plant residues or farmyard manure (FYM), and monthly information on soil cover (whether the soil is bare or covered with vegetation).
Long-term experimental datasets
summarizes two experimental sites (A and B) in paddy–upland rotation conducted in the research field of the Daisen branch, National Agricultural Research Center for the Tohoku Region. The soils at both sites are classified as Gray Lowland soils in the Japanese soil classification system for cultivated soils in Japan, with second approximation and as Typic Fluvaquents (Nishida et al. Citation2007) in the US Soil Taxonomy system (Soil Survey Staff Citation1998). These sites were originally used as paddy fields of single rice cropping for more than 100 years prior to the paddy–upland rotation experiments. Values in were obtained from Sumida et al. (Citation2005) and Nishida et al. (Citation2007). Initial SOC contents (in t C ha−1) were calculated from the measured SOC concentration and bulk density of topsoil to the depth shown in . For Site B, SOC in 1968 was used as the initial SOC, although the experiment started in 1970. Soil organic carbon concentration was measured by dry combustion method. Simulations were carried out using these depths.
Table 1. Summary of two long-term experimental sites
Site A had three rotation patterns after 1990 ()—continuous paddy, short-term upland conversion, and medium-term upland conversion—and a total of six plots with (n = 3) or without (n = 3) the application of rice straw (). Prior to 1990 all plots at Site A were continuously cultivated for paddy rice. In plots placed in short-term upland conversion, a soybean crop was cultivated seven times between 1990 and 2007 at one-year intervals (except 2001–2002, which was two years). Medium-term upland conversion had 13 soybean crops in this same period at three-year intervals (except 1999–2002, which was four years).
Table 2. Crop rotations of two long-term experimental sites
Table 3. The amount of carbon input to soils from crop residue and applied rice straw in each plot of Site A
Site B had eight plots and two rotation patterns—continuous paddy and long-term upland conversion. Each rotation pattern had a plot with rice straw compost and with or without nitrogen fertilizer and without rice straw compost and with or without nitrogen fertilizer (). Prior to 1982 all plots were continuously cultivated for paddy rice. In plots placed in long-term upland conversion, a soybean crop was cultivated 20 times between 1982 and 2007, including an 18-year continuous period between 1982 and 1999.
Table 4. The amount of carbon input to soils from crop residue and applied compost in each plot of Site B
Although both of the two experiments did not have replication of plots, these long-term experiments of paddy–upland rotation are still precious and useful, because rice field experiments in Japan are generally well managed and some significant trends can be found in these experiments without replication.
Model run
Equilibrium run prior to experiments
In modeling each set of experimental data, we set the initial SOC content to that measured at the beginning of each experiment () and then simulated the change in SOC with time for each plot under each management practice during the experimental period. To run the model, the initial amount of SOC in each of the five compartments (DPM, RPM, BIO, HUM, and IOM) is required, but usually only the total carbon content is available and the allocation of SOC in the five compartments is unknown. However, as described by Jenkinson et al. (Citation1999), assuming that the SOC content has reached equilibrium, the RothC model can be run inversely to calculate how much carbon needs to enter the soil annually to maintain a specific level of SOC. In the calculation process to produce the equilibrium condition, the allocation of SOC into each of the five compartments is also determined. To achieve this equilibrium condition, the RothC model should be run for a continuous duration of 10,000 years to stabilize the steady-state condition. We thus ran the model inversely for a duration of 10,000 years to calculate the amount of carbon input required to produce the SOC content at the beginning of each experiment and to set the SOC allocation in the five compartments to that at equilibrium. Soils were assumed to be covered with vegetation from May to September in this equilibrium run, assuming summer cropping (paddy rice) and winter fallow. Soils were assumed to be flooded only from May to September in case of using paddy-soil version of the RothC model. The carbon input for the equilibrium condition () was added to the soils only in September (month of harvest) because the annual distribution of inputs makes little difference in the calculated SOC, even if carbon is added in a single pulse (Coleman and Jenkinson Citation1996). A DPM/RPM ratio of 1.44, a value typical for most agricultural crops and grass (Coleman and Jenkinson Citation1996), was used. The values of IOM shown in were set by the equation IOM = 0.049 × SOC1.139 (Falloon et al. Citation1998). Monthly average air temperature and monthly precipitation (average of 30 years from 1961 to 1990) were obtained from the Japan Meteorological Agency (Citation1996). The potential evapotranspiration value of Thornthwaite (Citation1948) was used for the determination of the monthly open-pan evaporation.
Model run after start of experiments
Once the starting SOC content and its initial allocation into each of the five compartments had been established, the model was run with carbon input and soil cover information for each plot for each year until 2007. and show the average values of the annual carbon input to soils in Sites A and B, respectively, for the experimental period (however, each year's value was used in the simulation). Values include carbon input from crop residues (e.g. roots + stubble), rice straw (Site A), and rice straw compost (Site B).
Table 5. Monthly factors used in the Rothamsted Carbon (RothC) simulations to correct the decomposition rate of carbon based on whether paddy rice or upland crops were used
At Site A, cattle manure (2.5 t C ha−1 year−1) was applied to all plots from 1968 to 1987. This carbon input was included in the calculation but not included in . Cattle manure was treated as FYM in the RothC calculation. After 1988, no FYM was applied. Rice straw was applied from 1990 in those plots with rice straw. At Site B, plots were separated into with or without rice straw compost in 1970 and divided into eight different treatments in 1982. The amount of rice straw compost was 1.796 t C ha−1 year−1 from 1970 to 1987 and 1.244 t C ha−1 year−1 from 1988 to 2007. This rice straw compost was treated as FYM in the RothC model. Carbon inputs from rice straw in and rice straw compost in were calculated from the fresh amount, moisture, and carbon content of applied rice straw and rice straw compost from Sumida et al. (Citation2005) and Nishida et al. (Citation2007).
For both Sites A and B, only roots and stubble were incorporated into soils; other residues were removed from fields during rice cropping. Annual carbon inputs (in t ha−1; roots + stubble) of rice were estimated by multiplying the brown rice yield (in t ha−1) by 0.092, using a water content in brown rice of 15% and the proportion of dry matter production of each part of the rice plant as reported by Ogawa et al. (Citation1988): 37.2% grain, 8.2% chaff, 44.6% leaves and stalks, 6.7% stubble, and 3.3% roots, assuming 40% carbon concentration in dry matter. The proportion of dry matter production of each crop part can vary, even in the same crop, with varieties, weather, or nutrient condition. We used data from Ogawa et al. (Citation1988), however, because there was no other reliable datasets that include all crop parts, including belowground biomass and stubble as separate from the remaining aboveground biomass.
For soybean, the roots, stubble, and fallen leaves were incorporated into soils at Site A and all residues (roots, stubble, chaff, leaves, and stems) were incorporated into soils at Site B (except in 2003 and 2004, when only roots, stubble, and fallen leaves were incorporated). Carbon inputs as soybean residue were estimated by multiplying soybean grain yield by 0.31 for Site A and by 0.69 for Site B (but 0.31 in 2003 and 2004), using a water content of 15% in grain, the proportion of dry matter production of each part of the soybean plant as reported by Ogawa et al. (Citation1988) (33.1% grain, 17.9% chaff, 42.7% leaves and stalks, 3.3% stubble, and 3.0% roots), and 0.4 as the proportion of fallen leaves to all aboveground residues (leaves + stems + chaff; Hara et al. Citation2005), assuming 40% carbon concentration in dry matter. For simplicity, plant input carbon was added to the soils only in the month of harvest. A DPM/RPM ratio of 1.44 was used for all incoming plant residue. Soil cover information was obtained from the dates of sowing and harvesting as noted in Sumida et al. (Citation2005) and Nishida et al. (Citation2007).
Three simulations
We ran three different combinations (A, B, and C; ) of the two versions (original and paddy–soil versions) of the RothC model, which was applied both for equilibrium run prior to the experiment and model run after experiments started:
1. | The original version (RothC-26.3; Coleman and Jenkinson Citation1996). This simulation was expected to underestimate the SOC, at least in the continuous paddy plots, because the original model was developed for simulating SOC dynamics in non-waterlogged soils. | ||||
2. | The paddy–soil version (Shirato and Yokozawa Citation2005). In this simulation, the decomposition rate of all compartments was 0.2 and 0.6 times that of the original model during the rice cropping period (May–September, ) and outside this period (October–April, ), respectively, in years with summer paddy rice crops (year for paddy rice in ). Decomposition was therefore 0.6 times slower than the original throughout the year in years with upland crops (year for upland crop in ). This simulation was expected to fit continuous paddy plots but overestimate SOC in other plots, especially long-term upland conversion plots. | ||||
3. | Alternating use of original and paddy-soil versions. The paddy-soil version was used in years with summer paddy rice (year for paddy rice in ), and consequently the decomposition rate was reduced as noted earlier. In this simulation, the original version was used in years with summer upland crops (second year in ), without changing the decomposition rate (factor = 1). We expected this simulation to provide a good estimation of SOC. |
Evaluation of model performance
Two statistical indices were used to evaluate model performance: the root mean square error (RMSE), which represents the degree of coincidence, and the mean difference (M), which is a measure of model bias (positive values indicate consistent underestimation of measured data and negative values indicate consistent overestimation of measured data). Smaller value of RMSE and absolute value of M means good model performance. The statistical software package MODEVAL (Smith et al., Citation1996) was used for the calculation of these quantities.
Results and Discussion
Model versus observations
The original model () performed poorly compared to the other two simulations, indicating that the original model, which was developed for upland soil conditions, cannot accurately simulate SOC turnover in paddy soils even when upland crops are included in the rotation schedule. In contrast, simulations with the paddy–soil version () and with the alternate use of the two versions () showed generally good performance. Performance of the paddy–soil version appeared to be enhanced in plots without straw when short-term upland conversion was practiced, whereas performance from alternating the two versions appeared to be enhanced in plots with straw when short-term upland conversion or medium-term upland conversion was practiced. In continuous paddy plots, the paddy–soil version was used for both the paddy–soil and alternating versions; results were therefore the same for both versions and showed a slight overestimation of SOC in plots with straw and a slight underestimation in plots without straw. The differences in SOC between plots with and without straw were consistently larger in the simulations compared to the observations.
Figure 1. Comparison of simulation results for Site A. (A) Original model, (B) paddy–soil version, and (C) alternate use of the two versions. Solid and dashed lines show predicted changes in soil organic carbon (SOC) in plot with and without straw application, respectively. Closed and open triangles show observed SOC in plot with and without straw, respectively. P, continuous paddy; SU, short-term upland conversion; MU, medium-term upland conversion.
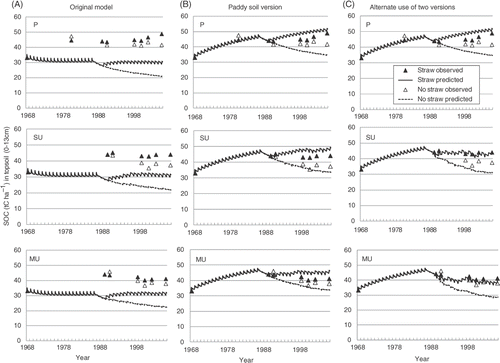
Results from continuous paddy plots differed from long-term upland conversion results at Site B (). In continuous paddy plots, the original model performed poorly compared to the other two simulations, as expected. The results of the paddy–soil and alternating versions were again the same (only the paddy–soil version was used), and both simulated the SOC well in plots with compost but underestimated the SOC in plots without compost. In long-term upland conversion plots, the paddy–soil version overestimated the SOC. This suggests that the soil condition of plots subjected to long-term upland conversion changes to an aerobic condition, which the paddy–soil version could not incorporate in the simulation. In plots with a long-term upland conversion pattern, the simulation of alternating uses provided results that better matched observations, even though it slightly overestimated the SOC. Performance of the original model () underestimated the results in the continuous paddy plots but provided relatively good estimation under long-term upland conversion plots although it was worse than alternating uses.
Figure 2. Comparison of simulation results for Site B. (A) Original model, (B) paddy–soil version, and (C) alternate use of the two versions. Thick solid and dotted lines show predicted soil organic carbon (SOC) in –compost plots with and without nitrogen (N) fertilizer application, respectively. Thin solid and dotted lines show predicted changes in SOC in +compost plot with and without N fertilizer application, respectively. Closed and open circles show observed SOC in –compost plot with and without N fertilizer application, respectively. Closed and open triangles show observed SOC in +compost plot with and without N fertilizer application, respectively. P, continuous paddy; LU, long-term upland conversion.
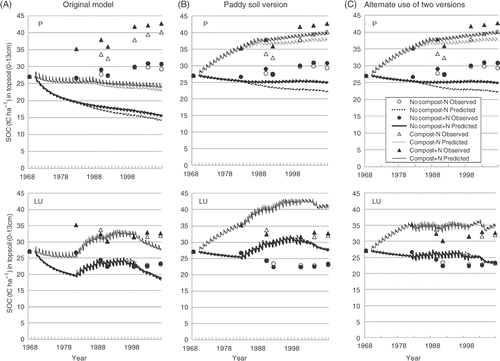
Overall, alternating the use of the two versions provided the best results among the three simulations; however, this needs to be confirmed quantitatively by statistical analyses.
Comparison of the three simulations
Using statistical analyses, the original model was shown to least accurately represent SOC conditions at the six plots of Site A and the four continuous paddy plots of Site B (). In these 10 plots, the higher RMSE and M values of the original model compared to the other two versions indicated that the original model underestimated the SOC. In contrast, the paddy–soil version overestimated the SOC in the four long-term upland conversion plots of Site B (indicated by the high positive RMSE values and high negative M values). The best simulation results were obtained from the alternate use of the two versions, which outperformed the original model in all 14 plots. The alternating use of the two versions also outperformed the paddy–soil version in all long-term upland conversion plots. In short- and medium-term upland conversion plots, the alternating use of the two versions showed better performance than the paddy–soil version in plots with straw application. Overall, we conclude that the alternate use of the two versions provided the best results for simulating SOC conditions.
Figure 3. Comparison of root mean square error (RMSE) and mean difference (M) among the three model simulations. Open, closed and gray bars show original model, paddy–soil version and alternate use of two versions, respectively. P, continuous paddy; SU, short-term upland conversion; MU, medium-term upland conversion; LU, long-term upland conversion.
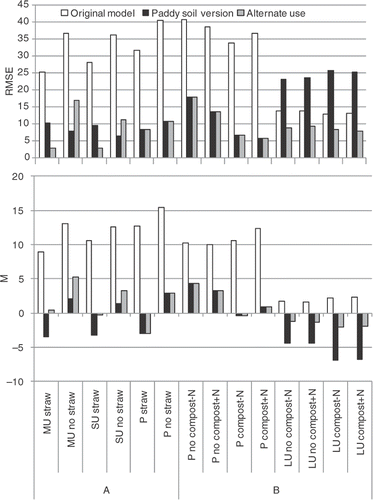
Comparison of root mean square error (RMSE) and mean difference (M) values with previous studies
illustrates a comparison between averages of RMSE and absolute values of M in our study () and previous studies using the RothC simulation in Japan. Shirato and Yokozawa (Citation2005) tested the original RothC model against nine long-term datasets of paddy soils () and developed the modified version for paddy soils () that was used in our study. They showed that the modified version for paddy soils had good performance. Shirato et al. (Citation2004) also tested the RothC model for Andosols () and developed a modified version (). They showed that the modified version for Andosols performed well, too (see difference between D and E in ). The average RMSE value that we obtained when alternating the use of the two versions was close to that of the paddy–soil version and less than that of the Andosols version. Similarly, the absolute value of M in our study was slightly greater than the paddy–soil version but less than the Andosols version. In short, the performance of alternate use of the two versions in our study was similar to or better than the performance of the modified model for paddy–soil and Andosols, which had good performance, and much better than the original RothC model for paddy soils and Andosols.
Figure 4. Comparisons of model performances of our study (A), previous studies (B) and (C) from Shirato and Yokozawa (Citation2005), and (D) and (E) from Shirato et al. (Citation2004). Closed and open bars show averages of root mean square error (RMSE) and absolute value of mean difference (M), respectively. Numbers above bars are values of the average. Numbers in parentheses in the labels on the x-axis indicate the number of plots.
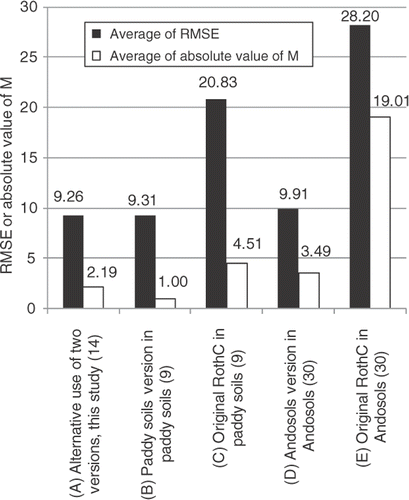
Smith et al. (Citation1997) compared the performance of nine SOM models by using long-term data sets from seven sites across a range of land uses, soil types, and climatic zones. They reported that the RothC model fell into a group of six models performing significantly better than another group of three models. In their study, the average values of RMSE were between 7 and 10 for the six better performing models (value of approximately nine for the RothC model), while they were between 17 and 23 for the other three models. The average RMSE value in our study (9.26) was therefore similar in performance to the better performing models. This comparison supports the high performance of the alternate use of two versions in our study, too. Because the observed SOC has error, too, the RMSE cannot be zero even if the model could be perfect. The RMSE value of around 10 means, therefore, very high level of performance.
We conclude from these comparisons that the alternate use of the paddy–soil version with the original RothC version provides a good technique for simulating changes in SOC with time in paddy–upland rotation experiments. This technique will lead to more accurate inventories of Japanese greenhouse gas emissions. Moreover, this modeling approach can help us manage soil fertility appropriately in paddy–upland rotation where the decline of soil fertility is a major concern.
Acknowledgments
This work was financially supported by the Ministry of Agriculture, Forestry and Fisheries, Japan (Development of mitigation and adaptation techniques to global warming in the sectors of agriculture, forestry, and fisheries).
References
- Coleman, K , and Jenkinson, DS , 1996. "RothC-26.3-A model for the turnover of carbon in soil". In: Powlson, DS , Smith, P , and Smith, JU , eds. Evaluation of Soil Organic Matter Models . Berlin: Springer-Verlag; 1996. pp. 237–246.
- Falloon, P , Smith, P , Coleman, K , and Marshall, S , 1998. Estimating the size of the inert organic matter pool from total soil organic carbon content for use in the Rothamsted Carbon Model , Soil Biol. Biochem. 30 (1998), pp. 1207–1211.
- Hara, Y , Huruhata, M , Yamashita, H , Tsuchiya, K , and Kusa, K , 2005. Effects of previous crops (soybean and paddy rice) and straw management on wheat growth in rotational cropping field , Bull. Kyushu Natl. Agric. Exp. Stn. 91 (2005), pp. 69–73, (in Japanese).
- Intergovernmental Panel on Climate Change (IPCC), 2000. Land use, Land-use Change, and Forestry . Cambridge: Cambridge University Press; 2000.
- Japan Meteorological Agency, 1996. Normal Values on Surface Meteorological Observation. (CD-ROM) . Tokyo: Japan Meteorological Agency; 1996.
- Jenkinson, DS , Meredith, J , Kinyamario, JI , Warren, GP , Wong, MTF , Harkness, DD , Bol, R , and Coleman, K , 1999. Estimating net primary production from measurements made on soil organic matter , Ecology 80 (1999), pp. 2762–2773.
- Jenkinson, DS , and Rayner, JH , 1977. The turnover of soil organic matter in some of the Rothamsted classical experiments , Soil Sci. 123 (1977), pp. 298–305.
- Kitada, K , Shimoda, H , Kamekawa, K , and Akiyama, Y , 1993. Changes in soil nutrients affected by rotation of upland and paddy crop in Gray Lowland Soil and search for the most suitable term of rotation , Jpn. J. Soil Sci. Plant Nutr. 64 (1993), pp. 154–160, (in Japanese with English abstract).
- Lal, R , 2004. Soil carbon sequestration to mitigate climate change , Geoderma 123 (2004), pp. 1–22.
- McGill, WB , 1996. "Review and classification of ten soil organic matter (SOM) models". In: Powlson, DS , Smith, P , and Smith, JU , eds. Evaluation of Soil Organic Matter Models . Berlin: Springer-Verlag; 1996. pp. 111–132.
- Ministry of Agriculture, Forestry and Fisheries of Japan, 2010. Statistics of the Crop Cultivation Area in Japan 2009 . Tokyo (in Japanese): The Ministry of Agriculture, Forestry and Fisheries of Japan; 2010.
- Mitsuchi, M , 1974. Characters of humus formed under rice cultivation , Soil Sci. Plant Nutr 20 (1974), pp. 249–259.
- Nishida, M , Iwaya, K , Sumida, H , and Kato, N , 2007. Changes in natural 15 N abundance in paddy soils under different, long-term soil management regimes in the Tohoku region of Japan , Soil Sci. Plant Nutr. 53 (2007), pp. 310–317.
- Ogawa, K , Takeuchi, Y , and Katayama, M , 1988. Biomass production and the amounts of absorbed inorganic elements by crops in arable lands in Hokkaido, and its evaluation , Res. Bull. Hokkaido Natl. Agric. Exp. Stn. 149 (1988), pp. 57–91, (in Japanese with English summary).
- Paustian, K , Andren, O , Janzen, HH , Lal, R , Smith, P , Tian, G , Tiessen, H , Van Noordwijk, M , and Woomer, PL , 1997. Agricultural soils as a sink to mitigate CO2 emissions , Soil Use Manage. 13 (1997), pp. 230–244.
- Shirato, Y , Hakamata, T , and Taniyama, I , 2004. Modified Rothamsted Carbon Model for Andosols and its validation: changing humus decomposition rate constant with pyrophosphate-extractable Al , Soil Sci. Plant Nutr. 50 (2004), pp. 149–158.
- Shirato, Y , and Yokozawa, M , 2005. Applying the Rothamsted Carbon Model for long-term experiments on Japanese paddy soils and modifying it by simple tuning of the decomposition rate , Soil Sci. Plant Nutr. 51 (2005), pp. 405–415.
- Smith, P , Martino, Z , Cai, Z , et al., 2007. "Agriculture. In: Climate Change 2007: Mitigation". In: Contribution of Working Group III to the Fourth Assessment Report of the Intergovernmental Panel on Climate Change . Cambridge: Cambridge University Press; 2007.
- Smith, J , Smith, P , and Addiscott, T , 1996. "Quantitative methods to evaluate and compare soil organic matter (SOM) models". In: Powlson, DS , Smith, P , and Smith, JU , eds. Evaluation of Soil Organic Matter Models . Berlin: Springer-Verlag; 1996. pp. 181–199.
- Smith, P , Smith, JU , Powlson, DS , et al., 1997. A comparison of the performance of nine soil organic matter models using seven long-term experimental datasets , Geoderma 81 (1997), pp. 153–225.
- Soil Survey Staff, 1998. Keys to Soil Taxonomy, . Washington, DC: USDA-Natural Resources Conservation Service; 1998.
- Sumida, K , Kato, N , and Nishida, M , 2005. Depletion of fertility and crop productivity in succession of paddy rice-soybean rotation , Bull. Natl. Res. Cent. Tohoku Reg. 103 (2005), pp. 39–52, (in Japanese with English abstract).
- Thornthwaite, CW , 1948. An approach toward a rational classification of climate , Geogr. Rev. 38 (1948), pp. 55–94.
- Zhang, M , and He, Z , 2004. Long-term changes in organic carbon and nutrients of an Ultisol under rice cropping in southeast China , Geoderma 118 (2004), pp. 167–179.