Abstract
In multi-soil-layering (MSL) systems, the effect of the materials and structural differences on the wastewater treatment performance and clogging mechanisms are not fully understood because of insufficient quantitative evaluations of water movement properties inside the system. In the present study, water movement properties were examined using laboratory-scale MSL systems consisting of one to six soil mixture layers (SML). The aims were to determine (1) changes in water movement characteristics inside the systems by varying the hydraulic loading rate (HLR), (2) the relationship between the number of inflow points and water movement characteristics, and (3) water movement characteristics and clogging mechanisms under wastewater treatment conditions.
As the HLR increased, the flow rates in the SML increased linearly, followed by a slight decline in the rate of increase, whereas flow rates in the permeable layers (PL) between the SML (PLb) increased exponentially except in the system with one layer. Water movement inside the systems with one inflow point inclined toward the central part of the system compared to that with three inflow points. Under wastewater treatment conditions, flow rates in the first SML decreased to 437 L m−2 day−1 from 1433 L m−2 day−1 in three days after applying wastewater. However, as the number of layers increased, high flow rates in the SML were maintained for longer periods. In the first to third SML, a decreasing trend of oxidation–reduction potential (Eh) values was correlated with a decrease in flow rates. Partial clogging in the first PLb was associated with Eh values in the PL of the upper part beginning to decrease. The study indicated that establishment of an optimum structure, materials, and aeration was required to maintain a proper level of permeability and anaerobic conditions inside the SML, which would facilitate simultaneous removal of organic matter, nitrogen and phosphorus.
Introduction
Wastewater treatment incorporating soil has been widely used not only in rural and some urban areas of developed countries but also in developing countries. Centralized water-borne sewage systems leading to multistage sewage treatment plants seem unsuitable as a blanket solution for developing countries, particularly in arid zones, due to their enormous investment, operating and maintenance costs and to their high water consumption (Zavala et al. Citation2002).
Clogging has been identified as one of the major problems in soil-based water treatment systems. It is believed that clogging results from accumulation of hydrated extracellular polymers (exopolymers), as well as cells with increased microorganism numbers and their secretions (Rodgers et al. Citation2004). Magesan et al. (Citation2000) showed that soil hydraulic conductivity decreased with increasing soil microbial biomass and carbohydrates. Other factors shown to contribute to clogging include deposition of organic or inorganic suspended solids in pore spaces and bacterially synthesized gases such as methane occluded in pore necks as bubbles (Baveye et al. Citation1998; Seki et al. Citation1998; Rodgers et al. Citation2004).
The author's group has attempted to address problems such as clogging associated with soil-based wastewater treatment systems and to develop a new system, designated as the multi-soil-layering (MSL) system. The MSL system consists of soil mixture layers (SML) alternating with permeable layers (PL) in a brick-like arrangement. Whereas the hydraulic loading rate (HLR) of conventional soil-based systems is typically 40–60 L m−2 day−1, demonstration-scale MSL systems have been shown to allow treatment of 30 mg L−1 biological oxygen demand (BOD) (on average) in river water and reduce this concentration to 2 mg L−1 (on average) with only limited fluctuations throughout the year at a high HLR of 4000 L m−2 day−1 (Unno et al. Citation2003). It has been shown that MSL systems do not require large areas for water treatment compared to conventional soil-based systems. The MSL system has the added advantage that its performance does not depend strongly on soil properties at the site, because the water purification function of the SML can be enhanced by incorporating a mixture of materials such as sawdust, jute, iron metal, charcoal and activated carbon (Wakatsuki et al. Citation1998, Citation1999; Luanmanee et al. Citation2001).
The MSL system offers the advantage of a reduction in clogging over the conventional method. However, some clogging problems remain in this system and its operation must be interrupted when surface ponding occurs as a result of clogging. Clogging is often caused by insufficient decomposition of organic materials due to a high HLR and an increase in microbial biomass following the rise in temperature from the end of winter to spring (Wakatsuki et al. Citation1998).
Many studies have been conducted on clogging in MSL systems. Aeration, particularly in upper parts of the system, is effective in its prevention (Sato et al. Citation2002b). This indicates that clogging probably occurs in the upper part of the system, and that aeration of this part enhances decomposition of the organic materials and biofilms that cause the clogging. Particle size distribution of the SML also influences clogging. Mixing a sandy soil into alluvial soil with high dispersibility was effective in prevention of clogging (Sato et al. Citation2005a). In addition, the size of the SML is related to clogging. A system with a wide size SML was observed to clog earlier than one with narrow size SML in research on high speed river water treatment (Sato et al. Citation2005a).
Although several measures have been taken to prevent clogging as noted earlier, the mechanisms determining how and where clogging occurs in MSL systems are unclear. In a previous study, we attempted to evaluate the water movement characteristics by installing water collection pipes inside MSL systems (Sato et al. 2005b). It was found that the outflow rate under the PL between the SML (PLb) significantly increased with an increase in HLR compared to that under the SML. However, it was difficult to confirm the clogging mechanisms because the evaluation only collected part of the water inside the system. Understanding of the clogging zone and its progress would be useful for developing measures to prevent clogging. The detection of clogging development would facilitate the maintenance of wastewater treatment in MSL systems.
It has also been observed that varying structures and materials influence wastewater treatment performance in MSL systems (Masunaga et al. Citation2003; Sato et al. Citation2005a; Chen et al. Citation2007). Their results indicate that the contact efficiency of the SML, which is probably the leading part of the water treatment processes, and wastewater is strongly related to performance. Therefore, elucidation of water movement mechanisms would also contribute to improved performance.
The foregoing discussion suggests that collecting all the water that flows through the SML and the PLb is necessary for elucidating water movement characteristics inside the system and the mechanisms underlying clogging development. Therefore, in this study six laboratory-scale MSL systems consisting of one to six SML were constructed, and water that flowed through the SML and the PLb was separately collected at the bottom of each system. The systems were used to determine: (1) changes in water movement characteristics inside the systems with varying HLR, (2) the relationship between the number of inflow points and the water movement characteristics, and (3) the water movement characteristics and clogging mechanisms under wastewater treatment conditions.
Materials AND Methods
The multi-soil-layering systems used in this study
shows the structure of the laboratory-scale MSL systems used in this study. For the MSL system, acrylic boxes of 10 cm (depth) × 50 cm (width) × 23 ∼ 73 cm (height) in size, forming an alternate brick layer-like pattern with zeolite and the SML, were used. The height of both the PL and SML was 5 cm and six MSL systems consisting of one to six SML were constructed. To collect the outflow water from the SML and the PLb separately, plastic divider plates were installed at the bottom of the systems as shown in . A 1.2 cm diameter hole was made at the bottom of each partition and a vinyl tube was attached to each hole. The SML contained a volcanic ash soil rich in organic matter classified as an Andisol (United States Department of Agriculture Citation1999), sawdust, approximately 1 mm diameter granular iron, and charcoal in ratios of 70%, 10%, 10% and 10%, respectively, on a dry weight basis. The Andisol was an A horizon sample from a soil at Mt Sanbe in Shimane, Japan, and had total carbon and nitrogen content of 5.5% and 0.3%, respectively. The bulk density of the SML was 0.84 g mL−1. The void spaces (PL) between each block and the block sides were filled with zeolite particles 3–5 mm in diameter. The individual systems and outflow positions are hereafter labeled as I–VI and 1–7, respectively. To measure oxidation-reduction potentials inside the system, 10 platinum electrodes were installed from the side in system VI, as shown in .
Experiment 1: Changes of water movement characteristics inside the systems by varying the hydraulic loading rate
To determine the water movement characteristics inside the system, well water was introduced into the systems at HLRs of 500, 1000, 2000, 3000, 4000, and 5000 L m−2 day−1. The quantity of outflow water from each vinyl tube was measured. The outflow rates were measured for 2 min to 12 h depending on the flow rate of each part of the system. The inflow water was divided into three points using a peristaltic pump with each point positioned right above the center of the SML in the top layer of the system as shown in . Whenever the HLR was changed, the outflow rate was measured after a continued inflow of more than one day. In addition, flow rates in the SML and the PLb in each system were calculated as outflow volumes per unit area per day in each partitioned part.
Experiment 2: Relationship between the number of inflow points and water movement characteristics
To determine the influence of inflow method, changes in water movement due to varying number of inflow points were investigated using well water and the same systems as for Experiment 1. Water movement from one inflow point set at the center of the top surface was measured and compared to that in Experiment 1 (with three inflow points). The outflow rates were measured at a HLR of 1000 and 5000 L m−2 day−1.
Experiment 3: Water movement characteristics under wastewater treatment conditions
In this experiment, domestic wastewater from a nearby community disposal plant was diluted three times with well water and was introduced into the same systems used for Experiments 1 and 2 to determine water movement inside the MSL system. The wastewater was diluted in a 1000 L storage tank approximately every three days. Average characteristics of the diluted wastewater were: pH 7.71, suspended solids (SS) 14.9 mg L−1, BOD 28.1 mg L−1, chemical oxygen demand (COD) 65.7 mg L−1, total nitrogen (T-N) 9.8 mg L−1 and total phosphorus (T-P) 1.0 mg L−1. Hydraulic loading rate was set at 1000 L m−2 day−1 and there were three inflow points as in Experiment 1. This experiment was started on August 12, 2005 and ended on December 22, 2005 (132 days). The outflow rates were measured five times during the period of the experiment, on August 15 (Day 3), August 30 (Day 18), September 29 (Day 48), November 9 (Day 89), and December 7 (Day 117). The flow rates were measured for 5 min to 8 h depending on the flow rate of each part. The results of Experiment 1 at a HLR of 1000 L m−2 day−1 were used as the reference data for Day 0. In addition, the oxidation-reduction potential (Eh) inside system VI was determined every 6 to 12 days. The results obtained when well water was introduced into the system at a HLR of 1000 L m−2 day−1 were used as the reference oxidation–reduction potential (Eh) data at Day 0.
Results
Experiment 1: Changes of water movement characteristics inside the systems by varying the hydraulic loading rate
shows the relationship between the HLR and flow rate and percentage of flow volume in each layer. The water movement properties in the MSL system with six layers were evaluated by combining the results of each system.
Figure 2. Relationship between hydraulic loading rate (HLR) and flow rate and percentage of outflow volume in each layer. The graphs depicted with dot patterns indicate data in the soil mixture layers.
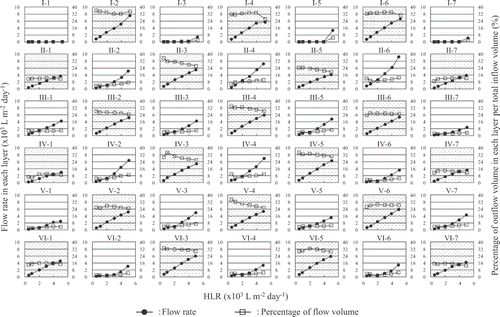
As the HLR increased, the flow rates in the SML increased linearly, followed by a slight decline in the rate of increase, whereas the rates in the PLb increased exponentially except for system I. When the flow rates in the SML and the PLb directly under it were compared (example I-2 and II-2), the latter was generally slower than the former up to a HLR of 4000 L m−2 day−1. In system I at a HLR of 5000 L m−2 day−1, outflow from the PLb was observed for the first time.
shows the relationship between HLR and the total proportion of water flow volume in the SML of each layer. The results from systems I, III, V and II, IV, VI are expressed separately because the component ratios of the SML and the PLb in the lowest layer of each system were different in these two groups (). The water movement in system I was influenced by inflow from the three points and there was significant flow of water through the SML ( and ). In system II, the proportion of water flow through the SML was also considerably affected by the inflow points, and was the lowest of all the systems. In system III, the proportion of water flow in the central part (III-4) was higher than that in III-2 and III-6 (). Despite the observed bias in flow, dispersion of water flow occurred as water moved down because the water flowed out from the PLb in system III in contrast to system I. The total proportion of water flow in the SML in system IV was higher than that in system II (), and dispersion proceeded with an increase in layers. Over the HLR range of 500 to 2000 L m−2 day−1, the total proportion of water flow in system IV increased with increasing HLR (). Additionally, the proportion of flow volume at both ends of system IV (IV-1 and IV-7) showed similar tendencies (). The dispersion was accelerated by the increase in HLR. However, for HLRs of more than 2000 L m−2 day−1 the total proportion decreased. The proportions of water flow in systems V and III were almost equal as shown in . However, whereas the percentage of flow volume in III-4 was the highest of the three SML of system III over all HLRs, the percentage of flow volume in V-4 significantly decreased and those of V-2 and V-6 increased with increasing HLR. Thus, the water flow inside system V dispersed more than inside system III with increasing HLR. Also, the total proportion of water flow in system VI was highest among systems II, IV and VI. The percentages of flow volume in VI-1 and VI-7 were higher than those in II-1, II-7, IV-1 and IV-7 (). Lateral flows inside the system increased as water moved down to the lower layers.
Experiment 2: Relationship between the number of inflow points and water movement characteristics
shows the flow rates in each layer of the system when inflow at one and three points was used at HLRs of 1000 and 5000 L m−2 day−1. When using one point of inflow, the water movement inclined toward the central part of the system at both HLRs compared to that using three points. In system I with one point of inflow, the inflow water only discharged from the SML in the center of the system (I-4) at the HLR of 1000 L m−2 day−1. In contrast, at the HLR of 5000 L m−2 day−1, the water was unable to infiltrate into the SML completely and flowed out through the PLb on both sides. Although such a bias in flow was observed with one inflow point, the flow rates at both sides of the system (such as positions 1, 2, 6, and 7) increased with increasing numbers of layers, and the dispersion of water flow proceeded as water moved down. The coefficient of variation in each system significantly decreased with increased numbers of layers. However, the flow rate in the central part of each system using one inflow point was higher than that of the systems using three inflow points, even in system VI ().
Figure 4. Relationship between the number of inflow points and the flow rate in each layer. Black and white bars indicate the flow rate in the soil mixture layers and the permeable layers between the soil mixture layers, respectively. HLR, hydraulic loading rate; CV, coefficient of variation. (a) HLR: 1,000 L m−2 day−1 and (b) HLR: 5,000 L m−2 day−1.
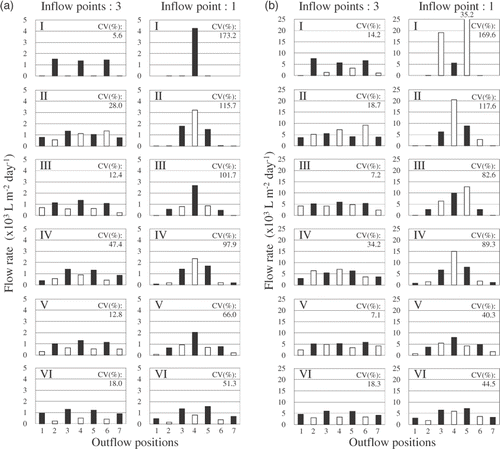
Experiment 3: Water movement characteristics under wastewater treatment conditions
Fluctuations of flow rate and the percentage of flow volume in each layer during 132 days of wastewater inflow are shown in . In system I, the flow rates of the SML on Day 3 decreased by 69.4% (mean value) compared to those at Day 0. After that, the rates gradually decreased with time. In contrast, the flow rate in the PLb increased with a decrease in the flow rate of the SML because the wastewater that was unable to infiltrate into the SML flowed into the PLb. In system II, the flow rates in II-1 and II-5 on Day 3 were higher than those on Day 0. The wastewater that intensively flowed into the PLb in the first layer infiltrated into the SML in the second layer. Additionally, higher flow rates in the SML were maintained in the lower layers and became longer in duration as the number of layers increased. In system VI, high fluctuation in the rate in the SML was not observed during the course of this study. In the PLb of system I, the flow rate in I-3 highly decreased to 280 L m−2 day−1 on Day 117 (). In contrast, these rates in I-1 and I-5 increased considerably.
Figure 5. Changes in flow rate and percentage of outflow volume in each layer during wastewater treatment. The graphs depicted with dot patterns indicate data in the soil mixture layers. Day 0 data show the flow rates and percentages when well water was introduced into the systems at a hydraulic loading rate of 1000 L m−2 day−1 in Experiment 1 ().
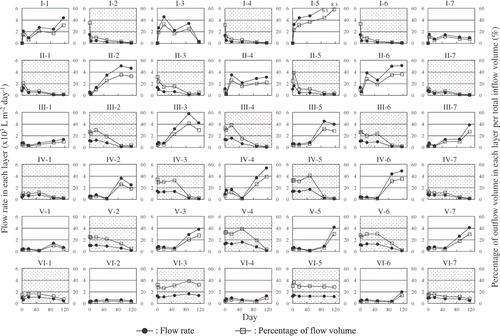
shows changes in Eh in system VI during wastewater treatment. The Eh in the first SML (1) decreased to −168 mV on Day 3, thereafter running at an average of −260 mV. This trend showed good agreement with the change of flow rate in I-4 during wastewater treatment (). The drop in Eh of the second SML (3) occurred later than that of (1), and the decreasing trend correlated with the change of flow rate in II-5. The decrease in Eh of the third SML (5) occurred later than those in (1) and (3). After 48 days, the Eh of (5) gradually decreased, following the decline in flow rates in the SML of system III ( and ). However, the decrease in Eh of the fourth SML (7) did not completely agree with declines in flow rates in the SML of system IV, and similarly there was no significant relationship between the changes in Eh of the fifth SML (9) and the flow rates in the SML of system V. In addition, although a slight decrease in Eh was observed from Days 20 to 30 in (5), (7), (9), and (10), the Eh of (9) and (10) increased around Day 90 ().
Figure 6. Changes in oxidation–reduction potential (Eh) of each layer in system VI during wastewater treatment. The graphs depicted with dot patterns indicate data in the soil mixture layers. Day 0 data show Eh when well water was introduced into the systems at a hydraulic loading rate of 1000 L m−2 day−1.
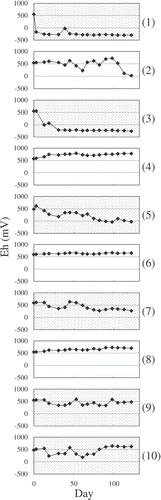
All Eh values in the PL except in (2) continuously showed high values. Oxidation–reduction potential in (2) were approximately 500 mV up to Day 100, although small fluctuations were observed. After that, the Eh gradually decreased to around 0 mV. When the Eh started to decrease, partial clogging in the PLb of system I also occurred, as shown in .
Discussion
Water movement properties in the multi-soil-layering system
In Experiment 1, flow rates in the SML increased linearly and the tendency to increase then slightly declined as the HLR increased (). This contrasts with our previous study where flow rates in the SML were almost constant in the HLR range of 1000–5500 L m−2 day−1 (Sato et al. Citation2005b). In contrast, flow rates in the PLb in this and in our previous study were similar and increased exponentially as the HLR increased. Differences in these results probably reflect only part of the water inside the system being collected in the previous study where 25 sets of water collection pipes (2 cm × 2 cm V-shape, 15 cm long) were installed under the SML and PLb in the MSL systems. The quantity of water that flowed out from each water collection pipe was measured at HLRs of 1000, 3000, and 5500 L m−2 day−1. The width of the water collection pipe was only about 2.5 cm in our previous study, whereas that of the SML was 12.0 cm (that of the PLb was 3.5 cm). Moreover, unstable flow (such as fingers) probably occurred in the transition of infiltrating water from a SML (fine-textured upper layer) to a PL (coarse-textured lower layer) (Hillel Citation1998). Thus water flow volumes in the SML were probably underestimated in our previous study.
The flow rates of the SML were generally higher compared to those of the PLb immediately beneath it (). The decreased flow rate in the PLb might be attributable to the generation of fingers and lateral flow into the SML adjacent to the PLb, because the water potential of the SML was lower than that of the zeolite. In addition, the exponential rise of flow rate in the PLb with an increase in HLR probably resulted from the change of water potential in the SML adjacent to the PLb. At the lower HLR, absorption of water into the SML with a lower potential than that in the PLb was probably suppressed to increase the flow rate in the PLb, except for systems I and II which were significantly influenced by the method of inflow. At the higher HLR, it is likely that the flow rate in the PLb increased relatively with an increase in water potential of the SML, because of its increased water content. Furthermore, in system I at the HLR of 5000 L m−2 day−1, the water that could not flow into the SML drained into the PLb (). The considerable increase in the flow rate of the PLb at a higher HLR presumably related to the water percolation capacity in the SML.
Although total proportions of water flow volume in the SML increased or remained around the same level over the HLR range from 500 to 2000 L m−2 day−1, those proportions gradually decreased at HLRs of more than 2000 L m−2 day−1 (). This suggests that enhancement of the contact efficiency of the SML and wastewater is very important in high speed treatments such as with a HLR of more than 2000 L m−2 day−1.
The increase in water dispersion with increasing numbers of layers ( and ) was probably caused by the lower water potential and limitation of water percolation capacity in the SML as discussed earlier. In the wastewater treatment using only gravel size materials, such as the PL of the MSL system (example, trickling filter), short-cut flow was probably generated under unsaturated flow conditions. Also, under the concentrated inflow method used in Experiment 2, short-cut flow would be more likely to develop. The structure of the MSL system, in contrast, provided effective water dispersion, and made possible a high speed water treatment maintaining the high water purification capacity of soil compared to conventional soil-based water treatment systems. However, in order to increase the water purification efficiency by using all of the materials in the MSL system, it is desirable and important to introduce the water to the system in as dispersed a way as possible. In addition, biased flow in the system arising from concentrated inflow would increase the risk of clogging in the upper part of the system.
In system I, under wastewater treatment conditions, the flow rates of the SML dropped sharply on Day 3, and then gradually decreased with time (). A similar tendency was observed in column experiments using an Andisol soil under saturated conditions (Seki et al. Citation1998). The decrease in flow rate was probably due to the deposition of microbial cells, hyphae, hydrated extracellular polymers, and suspended solids in the wastewater into pore spaces. These rates decreased to approximately 30 L m−2 day−1 on Day 117 () and were equivalent to HLRs in a conventional soil trench system (Perkins Citation1989). In the case of the conventional soil-based system, clogging in the surface soil caused only biased flow, surface ponding and runoff. In a MSL system, in contrast, even though the flow rate of the SML decreased, its structure made possible the continuation of the wastewater treatment, because water that could not flow into the SML flowed effectively into the next SML ().
In system I, the wastewater that was unable to flow into I-2, I-3, I-4 and I-6 positions due to clogging in I-3 flowed intensively through I-1 and I-5 on Day 117 (). After such partial clogging occurred in the PLb, it was suspected that clogging would readily occur in I-1 and I-5 due to concentrated flow in those parts of the system. These results suggest that clogging of the MSL system occurred in the first layer by, initially, the flow rate in the PLb of the first layer increasing with a decrease in the flow rate in the SML, and thereafter clogging in the PLb from the resulting greater water flow resulting in clogging of the whole MSL system. It has been observed in previous studies that clogging of a system may be recovered by decomposition of organic materials and biofilms after a rest period of two to three months (Wakatsuki et al. Citation1998, Citation1999). Based on this, it is proposed that alternate use of systems every three to four months would be desirable under the conditions used in this study, such as the system structure, materials used, the HLR, and wastewater quality.
In the first to third SML, the trend of decreasing Eh values was correlated with changing flow rates ( and ). This drop in Eh is probably attributable to oxygen consumption during decomposition of organic matter by microorganisms which proliferated due to inputs of some nutrients and organic matter from the wastewater. In addition, gas exchange might be difficult in the SML due to the decreased flow rate. In the fourth and fifth SML, however, there was no significant relationship between Eh and flow rates ( and ). This is probably because the organic matter content of the wastewater decreased from the first to the fourth and fifth layers. Water quality analysis suggests that the organic matter, as shown by BOD and COD, was largely decomposed in the upper layer (Sato et al. 2011).
Although a slight decrease in Eh values was observed from Days 20 to 30 in the third to sixth SML (), these decreases were probably attributable to enhanced decomposition of the mixed sawdust by increased microbial activity following the input of nutrients in the wastewater. In the fifth and sixth SML, however, the decreased Eh values increased around Day 90 (). This might suggest that the mixed sawdust in the SML was significantly decomposed during the 90 days. The sawdust was added to the SML as an electron donor to enhance denitrification. Considering the lifetime of material for denitrification, an effective period of about three months is too short, especially for treatment of a wastewater with a low carbon/nitrogen ratio. It is necessary to further study the slow decomposition of organic matter over prolonged periods, and methods that could be used to supply organic matter to a MSL system during wastewater treatment.
In the PL, aerobic conditions were maintained (). This is probably because gas exchange between the atmosphere and PL took place readily due to the use of gravel-sized material. There were relatively aerobic and anaerobic conditions in the PL and inside the SML, respectively. Thus, these different conditions could coexist together in the MSL system. However, Eh values in (2) gradually decreased from around Day 100 (). This was probably because a proliferation of bacteria and an accumulation of organic matter from the wastewater at that position in the system.
Relationship between water movement and structure in the multi-soil-layering system
Many studies have been conducted on the relationship between wastewater treatment efficiencies and the width and height of the SML in MSL systems. Masunaga et al. (Citation2002) and Chen et al. (Citation2007) have demonstrated that the ability to reduce BOD and T-P increased with reduction in the width of the SML. Their results suggest that the contact efficiency between the SML and wastewater increases with an increase in the side areas of the SML due to reduced width and division of the SML. In addition, our results of the present study indicate that the increase in the side areas was particularly effective when the flow rate in the PLb increased with a decrease in the flow rate in the SML during wastewater treatment and when the proportion of water flow in the PLb was high in the high speed treatment. Also, it is suggested that an increase in the number of PLb and SML due to a reduction in width led to dispersed wastewater flow inside the system similar to the effect of an increase in inflow points, and resulted in a further improvement of the treatment ability of the MSL system.
Sato et al. (Citation2005a) found that reducing the width prevented clogging in an experiment of direct river water treatment at a HLR of 4000 L m−2 day−1. In the present study, it is suggested that clogging in the MSL system was caused by a decrease in the flow rates of the PLb in the first layer due to the accumulation of biofilm and suspended solids. Further, it also suggests that the broad size of the SML and fewer PLb led to a concentrated flow of wastewater into the PLb and promoted clogging. Reducing the width of the SML and increasing the number of PLb possibly led to more dispersed wastewater flow inside the system and inhibited clogging in the PLb. Sato et al. (Citation2005a) reported that inorganic SS derived from wastewater infiltrated deeper into the system following a reduction in the width of the SML.
Chen et al. (Citation2007) reported that an increase in the number of layers following a reduction in the height of the SML resulted in a large increase in the ability to reduce COD, BOD, SS and T-P. Furthermore, an increase in the surface area of the SML following a reduction of its height was more effective than reducing the width. In the present study, it took longer before the flow rate in the SML started to decrease as the number of layers increased during wastewater treatment. Findings on soil-based water treatment systems indicate that clogging occurred near the ground surface where wastewater was irrigated, and that organic matter from the wastewater was also mainly decomposed within a few centimeters of the surface (Pell and Nyberg Citation1989; Seki et al. Citation1998), because the large supply of organic matter and nutrients from wastewater and oxygen from the air increased microbial activity near the surface. Consequently, with high SML, clogging near the surface of the SML probably inhibited infiltration of wastewater into deeper parts of the SML, and largely prevented their playing a part in the wastewater treatment. In contrast, increasing the number of layers by reducing the height probably leads to more efficient use of the soil materials for wastewater treatment and enhancement of the decomposition of organic matter and the adsorption of phosphorus to soil and iron materials.
In this study, although Eh was measured only at the center of the SML, the area of the SML adjacent to the PL was probably maintained in a relatively aerobic condition suitable for decomposition of organic matter, because the Eh of the PL was relatively high (). Based on the earlier discussion, it also is therefore suggested that the reason why the MSL system exhibited a high performance of wastewater treatment compared to the conventional soil-based system was that the soil surface layers with high microbial activities and water purification abilities were multi-layered.
Operation control of a multi-soil-layering system: clogging detection and aeration regime
This study showed that some central parts of the SML became anaerobic, and thus provided suitable conditions for denitrification. However, flow rates in the SML decreased at the same time. Sato et al. (Citation2002b) found that aeration in the upper part of a MSL system had a highly inhibitory effect on clogging, because aeration assisted the decomposition of organic matter. It is clear that control of water percolation in the SML by aeration and using highly permeable materials is important. It is likely, however, that excessive permeability in the SML will decrease adsorption of contaminants and lead to low denitrification efficiency due to the establishment of aerobic conditions by high gas exchange. To facilitate simultaneous removal of organic matter, nitrogen and phosphorus, establishment of the optimum structure, materials, and operational control, such as HLR and aeration regime, is required to maintain a proper level of permeability and anaerobic conditions inside the SML.
The changes of flow rate and Eh were highly related to each other in the SML and PL of the upper part of the system ( and ). This suggests that Eh might be used as a sensor of clogging. When several systems are operated alternately to avoid shutdown of the whole operation by clogging, it is important to know the progress of clogging to determine the time to switch to another system.
Sato et al. (Citation2002a) proposed that the best indicator to control aeration was the pH of the treated water. Under excessive aeration, nitrate leaching followed high nitrification rates and the pH decreased. In contrast, under conditions with a lack of aeration, nitrate concentrations were low because of high denitrification, and the pH increased. Likewise, the present study suggested that accumulation of organic matter and a decrease in water permeability cause very low Eh conditions. In contrast, under excess of high Eh conditions, although decomposition of organic matter will be accelerated, nitrogen removal will decrease due to high nitrification and low denitrification rates. Taken together, these studies suggest that measurement of both Eh and pH inside the system might be used to determine the optimum aeration regime. Soil is a complicated system and difficult to regulate. However, in order to optimize the water purification capacity of soil, it is necessary to further investigate whether measurements of pH and Eh can be used as indicators for detection of clogging and optimum control of aeration.
Conclusion
Water movement properties and clogging mechanisms in a MSL system were investigated. Water movement was influenced by water potential and permeability of materials, the inflow method and the HLR. Under wastewater treatment, higher flow rates in the SML were maintained in lower layers, and the duration of such higher rates became longer as the number of layers increased. Clogging of the MSL system was caused by an increase in the flow rate of the PLb due to a decreased flow rate of the SML in the first layer and subsequent clogging in the PLb due to the greater water flow.
Suggestions and recommendations for a MSL system in this study are summarized in and are as follows: (1) increase the number of SML by reducing the width as much as possible, especially at high HLR, (2) increase the number of layers by reducing the height of each layer to as low as possible, (3) introduce water to the system through as many dispersal points as possible, (4) use systems alternately every three to four months under the conditions used in this study, (5) the enhancement and maintenance of contact efficiency of the SML and wastewater is more important at HLRs of more than 2000 L m−2 day−1 under the conditions used in this study, (6) control the proper permeability of the SML by using highly permeable materials, (7) aeration to the upper part of the system to prevent clogging, maintain the proper permeability of the SML, thus enhancing the decomposition of organic matter, and (8) measure the pH and Eh inside the system during wastewater treatment to detect clogging and control aeration.
Table 1. Suggestions and recommendations for wastewater treatment by the MSL system
References
- Baveye, P , Vandevivere, P , Hoyle, BL , DeLeo, PC , and de Lozada, DS , 1998. Environmental impact and mechanisms of the biological clogging of saturated soils and aquifer materials , Crit. Rev. Environ. Sci. Technol. 28 (1998), pp. 123–191.
- Chen, X , Sato, K , Wakatsuki, T , and Masunaga, T , 2007. Effect of structural difference on wastewater treatment efficiency in multi-soil-layering systems: relationship between soil mixture block size and removal efficiency of selected contaminants , Soil Sci. Plant Nutr. 53 (2007), pp. 206–214.
- Hillel, D , 1998. "Entry of water into soil". In: Hillel, D , ed. Environmental Soil Physics . San Diego, CA: Academic Press; 1998. pp. 385–426.
- Luanmanee, S , Attanandana, T , Masunaga, T , and Wakatsuki, T , 2001. The efficiency of a multi-soil-layering system on domestic wastewater treatment during the ninth and tenth years of operation , Ecol. Eng. 18 (2001), pp. 185–199.
- Magesan, GN , Williamson, JC , Yeates, GW , and Lloyd-Jones, AR , 2000. Wastewater C:N ratio effects on soil hydraulic conductivity and potential mechanisms for recovery , Bioresour. Technol. 71 (2000), pp. 21–27.
- Masunaga, T , Sato, K , and Wakatsuki, T , , 2002: Environmental remediation using purification function of soil by multi-soil-layering system. Proc. of the 17th World Congress of Soil Science, Symposium 55, Registration No. 1108 in CD-ROM, 14–21 August 2002, Bangkok, Thailand.
- Masunaga, T , Sato, K , Zennami, T , Fujii, S , and Wakatsuki, T , 2003. Direct treatment of polluted river water by the multi-soil-layering method , J. Water Env. Tech. 1 (2003), pp. 97–104.
- Pell, M , and Nyberg, F , 1989. Infiltration of wastewater in a newly started pilot sand-filter system: I. Reduction of organic matter and phosphorus , J. Environ. Qual. 18 (1989), pp. 451–457.
- Perkins, RJ , 1989. Onsite Wastewater Disposal . Chelsea, MI: Lewis Publishers; 1989.
- Rodgers, M , Mulqueen, J , and Healy, MG , 2004. Surface clogging in an intermittent stratified sand filter , Soil Sci. Soc. Am. J. 68 (2004), pp. 1827–1832.
- Sato, K , Iha, Y , Luanmanee, S , Masunaga, T , and Wakatsuki, T , , 2002a: Long term on-site experiments and mass balances in waste water treatment by multi-soil-layering system. Proc. of the 17th World Congress of Soil Science, Symposium 55, Registration No. 1261 in CD-ROM, 14–21 August 2002, Bangkok, Thailand.
- Sato, K , Iwashima, N , Wakatsuki, T , and Masunaga, T , , 2011: Quantitative evaluation of treatment processes and mechanisms of organic matter, phosphorus, and nitrogen removal in a multi-soil-layering system. Soil Sci. Plant Nutr. 57, 475–486.
- Sato, K , Masunaga, T , Inada, K , Tanaka, T , Arai, Y , Unno, S , and Wakatsuki, T , 2005a. The development of high speed treatment of polluted river water by the multi-soil-layering method: examination of various materials and structures , Jpn. J. Soil Sci. Plant Nutr. 76 (2005a), pp. 449–458, (in Japanese with English abstract).
- Sato, K , Masunaga, T , and Wakatsuki, T , 2002b. Treatment of bio-genic wastewater by multi-soil-layering method: effect of various positions of aeration using small model systems , J. Jpn. Soc. Water Env. 25 (2002b), pp. 234–241, (in Japanese with English abstract).
- Sato, K , Masunaga, T , and Wakatsuki, T , 2005b. Water movement characteristics in a multi-soil-layering system , Soil Sci. Plant Nutr. 51 (2005b), pp. 75–82.
- Seki, K , Miyazaki, T , and Nakano, M , 1998. Effects of microorganisms on hydraulic conductivity decrease in infiltration , Eur. J. Soil Sci. 49 (1998), pp. 231–236.
- Unno, S , Wakatsuki, T , Masunaga, T , and Iyota, K , 2003. Study on direct treatment of river by multi-soil-layering method and its characteristics of water purification , J. Jpn. Coc. Civil Eng. 726/II-62 (2003), pp. 121–129, (in Japanese with English summary).
- United States Department of Agriculture, 1999. Soil Taxonomy . Washington, DC: United States Department of Agriculture; 1999.
- Wakatsuki, T , Masunaga, T , Kita, K , Shirahama, M , Zennami, T , and Harada, T , 1998. Wastewater treatment using soil ecosystems – pedosphere ecological engineering (Dojouseitaikei wo mochiita suishitsujouka – Dojouken no seitaikougaku) , J. Water Waste (YOUSUI TO HAISUI) 40 (1998), pp. 874–882, (in Japanese).
- Wakatsuki, T , Mori, J , Masunaga, T , and Kudou, K , 1999. Wastewater treatment based on soil ecosystems using multi-soil-layering method (Tadandojousouhou niyoru dojouseitaikei wo mochiita suishitujoukakouhou) , Environ. Conserv. Eng. (KANKYOU GIJUTSU) 28 (1999), pp. 806–813, (in Japanese).
- Zavala, MAL , Funamizu, N , and Takakuwa, T , 2002. Onsite wastewater differential treatment system: modeling approach , Water Sci. Technol. 46 (2002), pp. 317–324.