Abstract
A laboratory incubation study was conducted to determine the effects of soil aggregate size, soil moisture content and manure application on nitrous oxide (N2O) production through nitrification and denitrification. In Southern Hokkaido, soil samples were taken from a mineral soil layer (2.5–10 cm) of a grassland to which chemical fertilizer and manure had been applied. The soil aggregates were air-dried and sieved with 4.5 mm and 2 mm sieves, and the soil moisture was adjusted to 60% and 80% of field water capacity (FWC). Immediately after moistening, incubation was initiated and lasted for 9 days at 20°C. Following the start of incubation, a flush of N2O, carbon dioxide (CO2) and nitric oxide (NO) was observed. Production of all gases was higher in larger aggregates from the manure-applied soil. Productions of CO2 and NO were not significantly influenced by soil moisture content, but N2O production was considerably higher in 80% FWC as compared with 60% FWC. Based on the results of the N2O–nitrogen (N)/NO–N ratio, the process of N2O production was mainly due to nitrification in 60% FWC and denitrification in 80% FWC. Soil chemical properties, especially ammonium–N –N), nitrate–N (NO3–N) and water extractable organic C (WEOC) and microbial biomass C (MBC) also changed immediately after moistening. These changes were higher in larger aggregates from the manure-applied soil. Potential denitrification enzyme activity (DEA) was significantly higher in larger aggregates from manure-applied soil with higher moisture content. The N2O production in both 60% and 80% FWC correlated significantly with MBC and DEA. Regardless of soil moisture conditions, MBC correlated significantly with DEA, WEOC consumption and apparent N mineralization. These facts suggest that larger soil aggregates could have quickly developed suitable internal conditions for microbial activity inside the aggregates and consequently stimulated N2O production through nitrification and denitrification.
Introduction
Nitrous oxide (N2O) is one of the most important “trace gases” in the atmosphere. It contributes to reactions that influence atmospheric chemistry and radiative properties. Nitrous oxide is reactive and participates in destruction of ozone in the stratosphere; it is stable for about 120 years and contributes to the greenhouse effect in the troposphere (Crutzen Citation1981). Among the various sources of N2O, about 13.5% of the gas is produced from agricultural soil (IPCC 2007). Accordingly, global attention has focused on agricultural soils for the amelioration of N2O gas production.
Soil N2O production is influenced by a range of microbiological, chemical and physical soil processes and properties. Generally N2O is formed by two processes: nitrification and denitrification (Davidson and Swank Citation1990). There are natural sources of N2O and there are anthropogenic sources of N2O. The combined soil processes of mineralization, nitrification and denitrification convert applied organic and inorganic N-compounds to N2O, which then diffuses from the soil to the atmosphere and remains highly variable in space and time (Wrage et al. Citation2001; Davidson et al. Citation2004; Toma et al. Citation2010). Physical-chemical properties of soil strongly influence N2O emission. Specifically, soil aggregate is an important controlling factor for soil properties through the modification of pore distribution affecting aeration, water retention and drainage.
Soil aggregates are defined as groups of soil particles that are bound to each other more strongly than to adjacent particles. Organic matter “glues” are produced when soil biota break down dead roots and litter holds the particles together. Threadlike strands of fungi also bind particles into aggregates. In fact, pore structure in aggregated soil is composed of intra- (micro) and inter-aggregate (macro) pores. Both types of pores affect the transport of water, solutes and gases. The macro pores are a major determining factor in the transport of substances through the soil, whereas the micro pores are important in the stock or supply of substances in the soil (Lefelaar Citation1993). Aggregation can physically protect soil organic carbon (C) from decomposition by trapping the C within the aggregates and thereby reducing direct contact between the organic matter and soil microorganisms (Angers et al. Citation1997; Gregorich et al. Citation1997). Aggregates not only physically protect soil organic matter (Tisdall and Oades Citation1982), but also influence microbial community structure (Hattori Citation1988), limit oxygen diffusion (Sexstone et al. Citation1985), regulate water flow (Prove et al. Citation1990), and determine nutrient adsorption and desorption (Linquist et al. Citation1997; Wang et al. Citation2001). Aggregate stability is a good indicator of the content of organic matter, biological activity, and nutrient cycling in the soil. Drury et al. (Citation2004) separated the soil samples into six aggregate fractions (<0.25, 0.20–0.50, 0.50–1.0, 1.0–2.0, 2.0–4.0, 4.0–8.0 mm) and found that N2O from denitrification increased with increasing aggregate size. Increased N2O production with an increase in aggregate size was also reported by Khalil et al. (Citation2005). Renault and Stengel (Citation1994) found that small aggregates remained aerobic until saturated, but large unsaturated aggregates tended to have an anaerobic center. Aggregate size may be one of the significant factors that regulate the N2O emission from soil.
The N2O losses from manure plots extended over a longer period of time and were greater in magnitude than the losses from chemical nitrogen (N) fertilization (Jones et al. Citation2007). Other studies (Scott et al. Citation2000) have observed higher N2O fluxes from manure and sewage applications as compared to chemical N fertilizer application. High N2O fluxes from manure treatments can be partly explained by the higher total N input than chemical fertilizer treatments, providing more available N that can be mineralized over a longer period of time (Jin et al. Citation2009). Shimizu et al. (Citation2010) stated that the cumulative N2O flux showed a significant positive correlation with mineral N surplus, which was calculated as the difference between the total mineral N supply and aboveground N uptake. Another reason for the increased N2O fluxes could be the addition of organic C with the manure, which is known to stimulate denitrification (Bremner Citation1997).
Soil moisture content can also have a significant effect on N2O emission. Dobbie and Smith (Citation2003) found that rainfall (particularly around the time of N application), with its consequent effect on the water filled pore space (WFPS), was the main driving factor of N2O emission during the growing season in a grassland. David and Josep (Citation2005) demonstrated two events following precipitation into dry soils: a gradual increase of CO2 from enhanced biological activity. Emissions of carbon dioxide (CO2), N2O and methane (CH4) are affected by precipitation in at least two ways: (1) soil water content affects soil aeration which, in turn, affects microbial processes of production and consumption of these trace gases, and (2) the amount of precipitation can alter root turnover, litter-fall, decomposition, and mineralization, which, in turn, affect the availability of C and N substrates for trace gas production (Davidson and Schimel Citation1995; Davidson et al. Citation2000). However, Wang and Cai (Citation2008) showed that N2O production in soil increased slightly with an increase in soil moisture content from 40% to 70% of water holding capacity (saturated water content), but increased dramatically at 100% of water holding capacity in an incubation study with disturbed soil. The microbial activity could increase with soil water content until diffusion of oxygen (O2) is restricted and the environment becomes anaerobic (Linn and Doran Citation1984). Thus, soil water content is one of the major controlling factors for denitrification and nitrification processes. Soil microbial C is an important indicator of soil fertility and biological quality. It also plays a vital role in soil nutrient transformations, acting as a labile nutrient pool available to plants (Jenkinson Citation1990). Denitrifying enzyme activity (DEA) was proposed by Smith and Tiedje (Citation1979) as a way of assessing the potential optimum activity of existing denitrifying enzymes in soil. So, DEA is considered to be an important factor in identifying the main driver of denitrification among different aggregate size, water content and fertilizer management. Soil moisture, soil respiration, soil aggregation and soil compaction are key factors determining the aeration of soils and the formation of anoxic microsites (Granli and Bøckman Citation1994), and these factors may interact with and amplify each other in their effect on N2O production. The effects of these soil variables on N2O production remain unclear (Nishina et al. Citation2009). So, the main purpose of this study was to identify the interaction effects of different aggregate size, moisture content and fertilizer management on N2O production through N mineralization, nitrification and denitrification.
Materials and Methods
Study site
Soil samples used in this experiment were taken from a managed grassland in Shizunai Experimental Livestock Farm of Hokkaido University in Southern Hokkaido, Japan (42°26′N, 142°29′E). The grassland soil was covered by reed canary grass (Phalaris arundinacea) and meadow foxtail grass (Aleopurus pratenisi). The soil is derived from Tarumae (b) volcanic ash, and is classified as Thaptic Melanudands (Soil Survey Staff Citation2006; Mollic Andosol (IUSS Citation2006)). The characteristics of the study site are described in detail by Shimizu et al. (Citation2009). On the grassland, the experimental plots were established in 2005 and treated with chemical fertilizer (fertilizer plot) and manure (manure plot) (Shimizu et al. Citation2009). Annual N application rate was 124 kg N ha−1 as ammonium sulfate and ammonium phosphate in the fertilizer plot, and 296 kg N ha−1 as beef cattle manure with bedding litter (bark) in the manure plot. Manure C at 6884 kg C ha−1 was also applied in the manure plot. Soil samples were taken from a 2.5–10 cm depth in each plot on 27 June 2008 when the first harvest of grass was completed. The collected samples were brought to the laboratory and immediately stored in refrigeration at 4°C. The fresh soil samples were then spread on trays and air dried at room temperature.
Aggregate preparation
Air-dried soil samples were gently crushed by hand in order to pass through a 4.5 mm sieve; roots and stones were removed from the samples by hand. The soil aggregates of <2 mm were then separated using a 2 mm sieve. In order to measure the field water capacity (FWC), about 10 g of air dried soil of each aggregate size was saturated with water by draining loosely packed soil in a funnel of 0.55 g cm−3 for 24 h. The FWC of fertilizer-applied soil (hereinafter referred to as “FS”) with 4.5 mm and 2 mm aggregate fractions were 0.96 and 0.93 g g−1, and of manure-applied soil (hereinafter referred to as “MS”) with 4.5 mm and 2 mm were 1.01 and 0.98 g g−1, respectively. The FWC of aggregate fractions was determined to calculate the amount of distilled water that was added to achieve the desired moisture contents, which were 60% and 80% of FWC. Aggregate preparation and adjustment of moisture contents were performed at room temperature (20°C). The incubation procedure was initiated immediately after adding the distilled water to soil samples.
Incubation procedure
After adjusting the soil moisture content, 20 g dry-basis soil samples were loosely packed to a volume of 80 ml and to a bulk density of 0.52 g cm−3 in plastic cups and put into 1.8 L Mason jars. The jars were sealed tightly. A jar without a soil sample was also prepared and labeled as a blank. Ambient wet air was passed through a vinyl tube connected to the jar at a rate of 0.2 ml min−1 for 30 min to replace the gas in the jar completely. About 250 ml of an air sample was extracted from the headspace of the blank jar into a Tedlar bag using a 50 ml syringe for nitric oxide (NO) and CO2 analysis, and a sub-sample of 20 ml was taken from the Tedlar bag and injected into an evacuated 10 ml vial using a 25 ml syringe for N2O analysis. These air samples were regarded as time 0 min. The Mason jars were left at 20°C for 24 h, and then air samples were again taken in the same manner. The jars were opened after the air sampling and were immediately closed tightly in order to avoid change to soil moisture by evaporation from the aggregates. Incubation was conducted for 9 days from 17 to 25 June 2010. Three replicates were conducted in the experiment.
Nitrous oxide concentration was determined by a gas chromatograph with electron capture detector (model GC-14B, Shimadzu, Kyoto, Japan). Carbon dioxide concentration was analyzed with an infrared CO2 gas analyzer (ZFP9GC11, Fuji Electric System, Tokyo, Japan). Nitric oxide concentration was analyzed with a Chemiluminescence N Oxide Analyzer (Model 265 P, Kimoto Electric, Osaka, Japan). Gas production rate (F, µg kg−1 h−1) was estimated using the following equation:
The cumulative gas production (F) during the incubation (mg kg−1 9 d−1) was estimated using the following equation:
Soil properties analysis
Before and after incubation, concentrations of water extractable organic C (WEOC), –N and
and soil pH, total carbon (C) and total nitrogen (N) were analyzed. Also analyzed were soil microbial biomass C (MBC) before incubation and potential denitrification enzyme activity (DEA) after incubation. All analyses were conducted with three replicates. Wet soil samples were extracted with distilled water for measurement of WEOC,
and soil pH, and with 2 mol L−1KCl for measurement of
–N about two hours before and immediately after the incubation. Soil pH,
and WEOC concentrations were analyzed using a combined electrode pH meter (F-8 pH meter, Horiba, Japan), an ion chromatographer (QIC analyzer, Dionex Japan, Osaka, Japan) and a total organic carbon analyzer (Model TOC-5000A, Shimadzu, Kyoto, Japan), respectively. The
–N concentration was analyzed using colorimetry with indophenol-blue (Uvmini-1240, Shimadzu).
Total C and N concentrations of the soil samples were measured using an N/C analyzer (Sumigraph NC-1000, Sumika Chemical Analysis Service, Ehime, Japan) after soil samples were air dried and ground.
Microbial biomass C was analyzed before incubation by the chloroform fumigation-extraction method. It was calculated by the following formula:
Potential DEA was determined by the acetylene block technique, which inhibits the final conversion of N2O to N2 gas (Tiedje Citation1994). Soil samples were incubated under an anaerobic condition at 25°C with solution treated with chloramphenicol (1 g L−1) and (200 mg KNO3–N L−1). Fifteen g of wet soil was placed in a 100 ml conical flask, and 15 ml treated solution was added to the flask. The flasks were evacuated and flushed with N2 to ensure anaerobic conditions, and acetylene (C2H2) gas was added to a final concentration of 10% (10 kPa) in the headspace. The headspace gas was sampled with a syringe at 2 and 4 h and denitrification rates were calculated using regression coefficients obtained from plotting N2O concentrations against sampling time.
Judgment of denitrification and nitrification
The N2O–N/NO–N ratio is the index of N2O production from nitrification or denitrification (Lipschultz et al. Citation1981). The ratio is <1 during nitrification, 1–100 during both nitrification and denitrification, and >100 during denitrification. Using the N2O and NO production rates during the incubation, the N2O–N/NO–N ratio was calculated for the evaluation of denitrification and nitrification.
Statistical analyses
Statistical analyses were performed with SPSS 13.0 for Windows 2004 (SPSS Inc. Chicago, Illinois, USA). The differences between the treatments in gas productions, soil chemical properties, MBC and DEA were analyzed using analysis of variance (ANOVA). The differences of soil chemical properties before and after incubation were analyzed with paired t-test and simple linear regression analyses. Pearson's correlation coefficients (Pearson's R) were calculated between gas productions and soil chemical properties MBC and DEA.
Results
Soil chemical properties
shows the soil chemical properties (WEOC, –N,
, pH, total C and total N) measured before and after incubation, MBC measured before incubation, and DEA measured after incubation. Paired t-test for differences between soil chemical properties before and after incubation showed that WEOC,
–N, pH and total N were significantly different before and after incubation. In all treatments, fertilizer management (chemical fertilizer or manure), soil moisture content (60% or 80% of FWC) and aggregate size (<2 mm or 4.5 mm), WEOC, pH and total N decreased and
–N increased during the incubation. Nitrate-N concentration of fertilizer-applied soil decreased, but that of manure-applied soil increased during the incubation. However, there was no apparent trend in total C. These results indicate that soil properties, especially WEOC and
–N, changed immediately after the addition of water into soil and the influence of the change continued throughout the incubation. Results of ANOVA showed that all the soil chemical properties, MBC and DEA were significantly higher in 4.5 mm aggregates than in 2 mm aggregates. WEOC,
–N,
, MBC and DEA were higher in 80% of FWC than in 60% of FWC, but pH, total C and total N were not influenced by soil moisture. Manure-applied soil showed higher MBC and soil chemical properties except for total N than FS. However, DEA was not influenced by the fertilizer managements.
Table 1. Soil properties before and after incubation from different treatments
Gas production rates
shows the change in N2O production rates during the incubation. The N2O production rate was highest immediately after the incubation started. However, the highest N2O production rate was considerably different between 60% and 80% of FWC. In 60% of FWC, the highest production rate ranged from 1.97 (FS and 2 mm aggregates) to 7.01 (MS and 4.5 mm aggregates) µg kg−1 h−1, while in 80% of FWC, it ranged from 229 µg kg−1 h−1 (FS and 2 mm aggregates) to 711 µg kg−1 h−1 (MS and 4.5 mm aggregates). There was a tendency for MS to show a higher N2O production rate than FS in both soil moisture contents. In MS, N2O production rate decreased dramatically after the first flush, while it decreased slowly in FS. N2O production rate was higher in 4.5 mm aggregates compared to 2 mm aggregates for each fertilizer management with the same soil moisture content, except in the FS with 60% of FWC. The CO2 production rate showed a peak ranging from 2134 µg kg−1 h−1 (FS 2 mm aggregate with 60% of FWC) to 3817 µg kg−1 h−1 (MS 4.5 mm aggregate with 60% of FWC) until the fourth day after incubation started, and decreased gradually to 743 to 1816 µg kg−1 h−1 (). In each field management with each soil moisture content, CO2 production rate was higher in 4.5 mm aggregates compared to 2 mm aggregates. There was a tendency for CO2 production rate in both aggregate sizes to be higher in manure-applied soil than in fertilizer-applied soil. However, in manure-applied soil, it was higher in 80% than that in 60% of FWC, while there was no such tendency in fertilizer-applied soil.
Figure 1. Nitrous oxide (N2O) production rate during incubation from (a) fertilizer and (b) manure-applied soils with 60% of field water capacity (FWC), and from (c) fertilizer and (d) manure-applied soils with 80% of FWC. Data of every treatment represents means ± standard deviation. (n = 3). N, nitrogen.
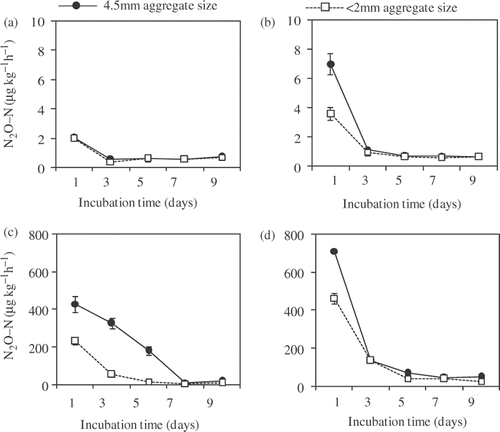
Figure 2. Carbon dioxide (CO2) production rate during incubation from (a) fertilizer and (b) manure-applied soils with 60% of field water capacity (FWC), and from (c) fertilizer and (d) manure-applied soils with 80% of FWC. Data of every treatment represents means ± standard deviation. (n = 3). C, carbon.
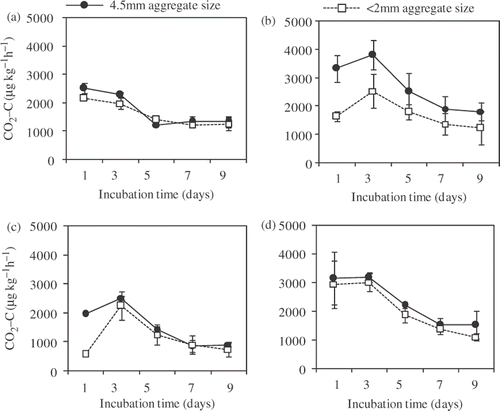
The production rate of NO during the incubation is illustrated in . The NO production rate was highest immediately after the incubation started, ranging from 0.90 µg kg−1 h−1 (FS and 2 mm aggregates with 80% of FWC) to 5.02 µg kg−1 h−1 (MS and 4.5 mm aggregates with 60% of FWC). The first flush of NO production was larger in 60% than that in 80% of FWC, and it was larger in 4.5 mm aggregates than in 2 mm aggregates in 60% of FWC, but similar between 2 mm and 4.5 mm aggregates in 80% of FWC. The production rate of NO in 60% of FWC decreased dramatically after the first flush, while in 80% of FWC it decreased only slightly after the first flush, then increased gradually. There was a tendency for the NO production rate in each aggregate size with the same soil moisture content to be higher in MS than that in FS.
Figure 3. Nitric oxide (NO) production rate during incubation from (a) fertilizer and (b) manure-applied soils with 60% of field water capacity (FWC), and from (c) fertilizer and (d) manure-applied soils with 80% of FWC. Data of every treatment represents means ± standard deviation. (n = 3). N, nitrogen.
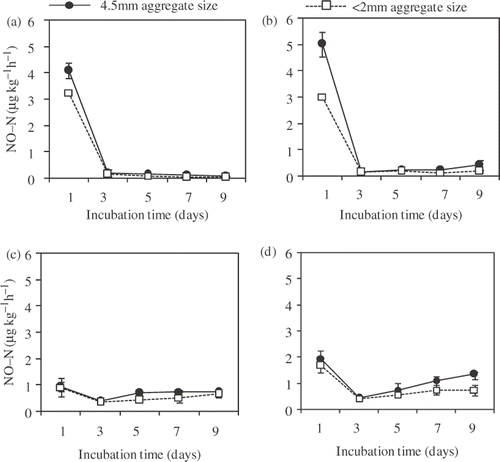
Ratio of N2O–N/NO–N
The ratio of N2O–N/NO–N in 60% of FWC mostly ranged from 1–100 and decreased with an increase in N2O production (). However, the ratio with 80% of FWC was >100 or near 100 and increased with an increase in N2O production (). The results indicate that the N2O production during incubation in 60% of FWC was derived mainly from nitrification while N2O production in 80% of FWC was derived from denitrification.
Cumulative gas productions
Cumulative N2O production was considerably different between 60% of FWC and 80% of FWC (). In 60% of FWC, cumulative N2O production was similar among fertilizer managements and aggregate sizes, ranging from 0.170 mg kg−1 9 day−1 (FS and 2 mm aggregates) to 0.400 mg kg−1 9 day−1 (MS and 4.5 mm aggregates), while it ranged from 12.0 mg kg−1 9 day−1 (FS and 2 mm aggregates) to 40.7 mg kg−1 9 day−1 (FS and 4.5 mm aggregates) in 80% of FWC. The cumulative N2O production was significantly influenced by fertilizer management, aggregate size, and soil moisture content. There was an interaction between each pair of treatments and among the three treatments, due to a large difference in N2O production between moisture contents.
Table 2. Cumulative productions of carbon dioxide (CO2), nitrous oxide (N2O) and nitric oxide (NO) from different treatments
Cumulative CO2 production ranged from 258 mg kg−1 9 day−1 (FS and 2 mm aggregates with 80% of FWC) to 611 mg kg−1 9 day−1 (MS and 4.5 mm aggregates with 60% of FWC) (). The cumulative CO2 production was significantly influenced by fertilizer management and aggregate size, but not influenced by soil moisture content. There was no significant interaction among the treatments.
Cumulative NO production ranged from 0.114 mg kg−1 9 day−1 (FS and 2 mm aggregates with 80% of FWC) to 0.227 mg kg−1 9 day−1 (MS and 4.5 mm aggregates with 80% of FWC) (). The cumulative NO production was not influenced by soil moisture content but was significantly higher in the larger MS aggregate. However, there was a significant interaction between fertilizer management and soil moisture or management and aggregate size.
presents the Pearson's correlation coefficients of the relationship between gas productions and each of the soil chemical properties before and after incubation, MBC and DEA. Since there was a significant difference in WEOC, –N,
, MBC, DEA, and cumulative N2O between 60% of FWC and 80% of FWC ( and ), regression analysis was separately conducted for each soil moisture treatment.
Table 3. Pearson's correlation coefficient (r) of nitrous oxide (N2O), carbon dioxide (CO2) and nitric oxide (NO) productions to soil chemical properties, microbial biomass carbon (C) (MBC) and denitrification enzyme activity (DEA)
The results of regression analysis for cumulative N2O production in 60% of FWC showed a significant positive correlation with WEOC, –N, and pH before and after incubation and MBC. However, it was significantly correlated with
only after incubation. On the other hand, the results for cumulative N2O production in 80% of FWC had a significant positive correlation with WEOC,
–N,
, pH and MBC before incubation and WEOC,
, pH and DEA after incubation. Regardless of the moisture content, N2O production significantly correlated with WEOC,
–N,
before and after incubation, MBC before incubation and DEA after incubation. N2O production did not strongly correlate with pH, total N and total C.
Cumulative CO2 production in 60% of FWC showed a similar tendency to that of cumulative N2O production. In other words, cumulative CO2 production in 60% of FWC showed a significant positive correlation with WEOC, –N, and pH before and after incubation and MBC, and it was significantly correlated with
concentrations only after incubation. On the other hand, cumulative CO2 production in 80% of FWC was different from that of the cumulative N2O production in that 80% of FWC showed significant and positive correlation with
, and pH before and after incubation. Although there was a significant correlation with WEOC before incubation, correlation with WEOC after incubation was not significant. Without considering moisture content, CO2 production showed significant correlation with
–N and pH before and after incubation, while it did not show significant correlation with other soil properties.
Cumulative NO production in 60% of FWC showed a significant correlation with MBC, DEA and all soil properties except for total N before incubation. While cumulative NO production in 80% of FWC showed a significant correlation with WEOC, –N and pH before and after incubation, it was significantly correlated with
after incubation. Regardless of the moisture content, NO production showed a significant correlation with WEOC,
–N, pH, total C before and after incubation, MBC before incubation and DEA after incubation. Nitric oxide production showed a significant correlation with
only after incubation.
Interaction of gases
The relationship between cumulative N2O and CO2 production was significant in 60% of FWC (r = 0.860, P < 0.01, n = 12) while there was no significant relationship in 80% of FWC (r = 0.536, NS, n = 12). The relationship between NO and CO2 production was also significant in 60% (r = 0.762, P < 0.01, n = 12) and 80% of FWC (r = 0.776, P < 0.01, n = 12).
Discussion
Soil properties
Soil properties, especially WEOC and –N, changed immediately after adding the water for both aggregates and this situation continued throughout the incubation process () because the added water could activate the microbial processes inside the soil aggregate. In a lab incubation study, Martin and David (Citation1996) found a rapid change in WEOC when moistening the dry and sieved soil samples and there was a 40% decline from the initial WEOC values after 9 weeks. The results of this study also showed a trend of decrease in WEOC during the 9-day incubation for all treatments (). On the one hand, the concentrations of
–N increased and that of
decreased with incubation time in fertilizer-applied soil (). A similar result was reported by Zhang and Wienhold (Citation2002). They showed that at soil moisture levels greater than 80% of WFPS,
concentration declined rapidly and
–N concentration increased; this is likely due to anaerobic conditions existing at higher WFPS levels during an incubation study. Mori et al. (Citation2010) also reported a similar result from an incubation study with different moisture contents. On the other hand, our results showed that the
content of MS increased after incubation, indicating that the consumption of
by denitrification was slower than the production of
from nitrification. Actually, the
–N content after incubation is the net result of the mineralization of organic N and nitrification, and the
content is the net result of
production from nitrification and consumption by denitrification. Wang and Cai (Citation2008) reported a similar result where
content increased after incubation even at a soil moisture content of 100% of FWC. In our study, larger aggregates always showed higher concentration of soil properties, especially WEOC,
–N,
and MBC compared to those of smaller aggregates (). Several studies showed increasing organic C, MBC and potentially mineralized organic matter with increasing aggregate sizes (Puget et al.
Citation1995; Jastrow et al.
Citation1996; Semenov et al.
Citation2010). Therefore, macro aggregates are generally associated with larger concentrations of soil organic C, mineralizable nutrients and microbial biomass as compared with micro aggregates (Gupta and Germida Citation1988).
Carbon mineralization
The effect of different moisture contents on CO2 production was not significant in this study (). Different fertilizer managements and aggregate sizes might have more prominent effects on CO2 productions, possibly suppressing the effect of moisture content. Application of chemical fertilizer adds only N to the soil while manure application adds both N and C to the soil. For this reason, more WEOC was produced in the manure-applied soil (). Therefore, more CO2 production was observed in MS in this study (). Steven et al. (Citation2006) found similar results. Zou et al. (Citation2005) defined soil labile organic C as the fraction of organic C with most rapid turnover which is degradable during microbial growth with its oxidation that drives the CO2 production. Therefore, the higher amount of MBC in larger aggregates shown in may induce higher labile C resulting in greater CO2 production. Semenov et al. (Citation2010) demonstrated that a higher content of potentially mineralized organic matter occurred in larger aggregates (3–1 mm, 1–0.25 mm) than in the smaller aggregates (<0.25 mm). Puget et al. (Citation1995) and Jastrow et al. (Citation1996) also observed increasing proportions of labile C with increasing aggregate size. These results indicate higher CO2 emission from larger aggregates as compared to emissions from smaller aggregates in our study ().
Several studies have implied that the amount of WEOC was a measure of a readily available resource for microbial growth and biological decomposition, often being considered as a good index of C availability (Paul and Beauchamp Citation1989; Liang et al. Citation1996; Jensen et al. Citation1997). Carbon dioxide is released largely from microbial decay (Janzen Citation2004; Smith Citation2004). Results from regression analysis showed a significant positive correlation between CO2 production and WEOC before incubation (). The slope value of the regression equation between CO2 production and MBC was higher in 60% of FWC (y = 0.189x + 105.5) than in 80% of FWC (y = 0.044x + 241.4), indicating that MBC can produce CO2 more efficiently in 60% of FWC than in 80% of FWC. On the other hand, WEOC consumption was significantly correlated to MBC regardless of soil moisture content (r = 0.906, P < 0.01). Comparing WEOC consumption (reduction of WEOC during incubation) and CO2 production, all samples in 80% of FWC produced less CO2 than WEOC consumption (). These results suggest that WEOC was consumed not only by decomposition but also by assimilation in the higher soil moisture condition, and the microbial activities are higher in larger aggregates. Larger aggregates can produce water saturated zones inside the aggregates (Khalil et al. Citation2005). This may contribute more effectively to the decomposition of WEOC in the soil desiccation process by supplying water from the saturated zone. Microbial biomass C and WEOC were considered to be important limiting factors for CO2 production. Soil aggregates control the microbial activities by producing the water saturated zones among aggregates.
Figure 5. Relationship between cumulative carbon dioxide (CO2) production and water extractable organic carbon (WEOC) consumption. Data of three replications were used. FWC, field water capacity.
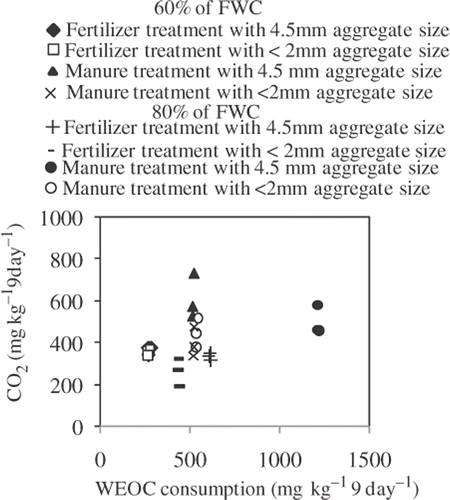
Results from this study also showed significant positive correlation between CO2 production and –N or
(). The availability of mineral N may influence the decomposition of organic matter and further CO2 production when N becomes a limiting factor for microbial activities (Hadas et al.
Citation1998). Soil pH was significant factor for CO2 production in all cases (). An incubation study showed significant positive effects of soil pH on soil respiration (Andersson and Nilsson Citation2001), particularly since microbial activity like denitrification increases with rising pH values (Ellis et al.
Citation1998).
Nitrous oxide production
This study showed a high initial peak of N2O production immediately after the addition of water (). An increase in N2O production after moistening of dry soil has also been reported by Kester et al. (Citation1997). They ascribed this to the presence of nitrifiers which can produce N2O in soil quickly after the rainfall event. Davidson et al. (Citation2004) observed N2O flush immediately after precipitation in a field study. They ascribed this to the change in the aeration status of soil which affects the microbial processes for the production of N2O. This suggests that the denitrification process may be a significant process for N2O production immediately after rainfall events.
In our study, cumulative N2O production in 60% of FWC revealed a significant correlation with –N both before and after incubation (), and the N2O production rate increased with the decrease in N2O–N/NO–N ratio from 100 to 1 (). These results indicate that nitrification was the main driver of N2O production, and
–N was the reactant in this nitrification process (Lipschultz et al.
Citation1981; Hadar et al. Citation2010). On the other hand, cumulative N2O production in 80% of FWC showed significant correlations with
(), and the N2O production rate increased with an increase of N2O–N/NO–N ratio from 1 to 100 (). These facts suggest that denitrification is the main process of N2O production, and
was the reactant in the denitrification process (Lipschultz et al.
Citation1981; Livesley et al. Citation2009; Toma and Hatano Citation2007). Actually, denitrification requires an anaerobic environment, while aerobic conditions are necessary for nitrification. Furthermore, high soil moisture content favors anaerobic conditions for denitrification and N2O appeared to be the main product of denitrification. Therefore, it is clear that the relative importance of each process to N2O production depends largely on soil water content and the aeration status of the soil. Granli and Bøckman (Citation1994) reported that when nitrification was the main process of N2O production, emission of N2O from soil tended to increase with an increase in soil pH. In our study, the significant positive relationship between N2O production and pH was found in 60% of FWC (). Sirwan et al. (Citation1997) demonstrated that N2O and NO fluxes decreased remarkably with an increase in soil acidity. Later on they concluded that the microbial community of the soil had adjusted to the low pH and this microbial community was responsible for the entire production of N2O from nitrification-denitrification processes and for much of the NO release. Our results also showed a significant positive correlation between NO and pH especially in 60% of FWC (). Production of N2O in each soil moisture condition showed a significant positive correlation with MBC (). The MBC was greater in the larger size of soil aggregates and in higher soil moisture content, and it also increased with manure application (). Furthermore, MBC was significantly correlated with apparent N mineralization (increase in NH4 + NO3 during the incubation process) regardless of soil moisture (r = 0.795, P < 0.01, n = 24). Also, as described above, MBC was significantly correlated with WEOC consumption regardless of soil moisture content. Moreover, there was a significant relationship between MBC and DEA (r = 0.796, P < 0.01, n = 24). This suggests that all samples used in this experiment were able to quickly develop the conditions suitable for denitrification, producing more N2O immediately after moistening. Therefore, these findings indicate that an increase in soil aggregate size increases N2O production due to the development of suitable conditions for microbial activity inside aggregates, which is enhanced by precipitation and manure application.
Most soil microorganisms get their energy from organic materials. Nitrogen mineralization and the following transformation, i.e., nitrification and denitrification whereby N2O is produced as an immediate product, are intimately linked to organic C decomposition (Wrage et al. Citation2001). A positive relationship between N2O and CO2 production was found in this study, especially in 60% of FWC. This suggests that N2O production is strongly affected by organic matter decomposition. Toma et al. (Citation2010) found a very strong significant relationship between N2O and CO2 emissions, which were stimulated more by application of crop residues with a lower C/N ratio. This could be a factor explaining why manure-applied soil with high organic matter might have been responsible for higher N2O production. Jin et al. (Citation2009) found higher N2O production from MS than the FS in a field experiment conducted for three years. Relationship between N2O and CO2 productions in 80% of FWC was not significant. In our study, CO2 production did not significantly vary with moisture content, but N2O production significantly varied with moisture content. In other words, higher moisture content resulted in extremely high N2O production. Linn and Doran (Citation1984) observed a linear relationship between CO2 and N2O productions at 60% WFPS, where water content below 60% WFPS limited microbial activities but water content above 60% WFPS decreased only aerobic microbial activities. They also found that the relative activity of anaerobic denitrification was negligible at 60% WFPS, but that it increased with increasing water and reached a maximum level at saturation.
In this experiment, larger aggregates of both fertilizer and manure-applied soils showed higher N2O flux than smaller aggregates at both moisture contents because of the association of higher amounts of –N and NO3 -N with larger aggregates. Khalil et al. (Citation2005) used an incubation study and reported an increase in N2O production in aggregated soil. They suggested that the increase is dependent on aeration within the aggregate. Drury et al. (Citation2004) also revealed that larger aggregate size fractions resulted in greater N2O production from denitrification where soil aggregates were incubated anaerobically using a C2H2 inhibition technique. Lefelaar (Citation1993) used an experimental respirometer system and developed a theoretical simulation model to study the dynamics of water movement, oxygen supply and biological processes on the aggregate scale in unsaturated soil. It was suggested that larger aggregates contain more intra-(micro) and inter-aggregate (macro) pores, which determine the transport properties of water, solutes and gaseous substances through the soil. The transport properties are important in determining the biological activity of the soil. Macro pores are a major determinant in the transport of substances through the soil from and to the atmosphere. Micro pores are important in the transport of substances from the larger pores in the soil into and out of the aggregates, structural elements, or more generally, the denser structure in the soil. Moreover, larger aggregates of both fertilizer and manure-applied soil showed higher FWC, indicating that larger aggregates are able to hold more water inside the soil pores and decrease the O2 concentration in the soil. Larger aggregates have greater anoxic volumes for which the amount of denitrified N is higher within the larger aggregates, resulting in higher N2O production by larger soil aggregates.
Conclusions
There was a strong and rapid effect of soil wetness on N2O production, which can induce the flush of N2O emission immediately following precipitation. This was enhanced by increase in aggregate size and manure application. Moistening also increased the production of CO2 and NO, and induced a rapid change in –N,
, WEOC and MBC. These effects of moistening continued for a while (at least 9 days). Larger aggregates produced more gases, and induced more WEOC consumption and N mineralization, which was stimulated by manure application. In this study, the effect of soil moisture content on N2O production was stronger than that on CO2 and NO productions. Considerably larger N2O production was found in 80% of FWC than in 60 % of FWC. The result of N2O–N/NO–N ratio suggested denitrification in 80% of FWC, but nitrification in 60% of FWC is considered to be the main process of N2O production.
Acknowledgements
We would like to thank the technical staff of the Shizunai Livestock Farm for their help in collecting the soil samples. This study was partly supported by a research grant provided by the project entitled ‘Establishment of good practices to mitigate greenhouse gas emissions from Japanese grasslands’ funded by the Racing and Livestock Association.
References
- Andersson , S and Nilsson , S . 2001 . Influence of pH and temperature on microbial activity, substrate availability of soil-solution bacteria and leaching of dissolved organic carbon in a mor humus . Soil Biol. Biochem. , 33 : 1181 – 1191 .
- Angers , DA , Recous , S and Aita , C . 1997 . Fate of carbon and nitrogen in water-stable aggregates during decomposition of 13C 15N-labeled wheat straw in situ . Eur. J. Soil Sci. , 48 : 295 – 300 .
- Bremner , JM . 1997 . Source of nitrous oxide in soils . Nutr. Cycling Agroecosyst. , 49 : 7 – 16 .
- Crutzen , PJ . 1981 . “ Atmospheric chemical processes of the oxides of nitrogen including nitrous oxide ” . In Denitrification, nitrification and atmospheric nitrous oxide , Edited by: Delwiche , CC . 17 – 44 . New York : John Wiley .
- David , RS and Josep , P . 2005 . Short-term CO2 emissions from planted soil subject to elevated CO2 and simulated precipitation . Appl. Soil Ecol. , 28 : 247 – 257 .
- Davidson , EA , Ishida , FY and Nepstad , DC . 2004 . Effects of an experimental drought on soil emissions of carbon dioxide, methane, nitrous oxide, and nitric oxide in a moist tropical forest . Global Change Biol. , 10 : 718 – 730 .
- Davidson , EA and Schimel , JS . 1995 . “ Microbial processes of production and consumption of nitric oxide, nitrous oxide and methane ” . In Biogenic Trace Gases: Measuring Emissions from Soil and Water , Edited by: Matson , PA and Harriss , RC . 327 – 357 . Oxford : Blackwell Science .
- Davidson , EA and Swank , WT . 1990 . Nitrous oxide dissolved in soil solution: an insignificant pathway of nitrogen loss from a southeastern hardwood forest . Water Resour. Res. , 26 : 1687 – 1690 .
- Davidson , EA , Verchot , LV and Cattânio , JH . 2000 . Effects of soil water content on soil respiration in forests and cattle pastures of eastern Amazonia . Biogeochem. , 48 : 53 – 69 .
- Dobbie , K and Smith , KA . 2003 . Nitrous oxide emission factors for agricultural soils in Great Britain: The impact of soil water-filled pore space and other controlling variables . J. Geophys. Res. , 104 : 26891 – 26899 .
- Drury , CF , Yang , XM , Reynolds , WD and Tan , CS . 2004 . Influence of crop rotation and aggregate size on carbon dioxide production and denitrification . Soil Till. Res. , 79 : 87 – 100 .
- Ellis , S , Howe , MT , Goulding , KWT , Mugglestone , MA and Dendooven , L . 1998 . Carbon and nitrogen dynamics in a grassland soil with varying pH: Effect of pH on the denitrification potential and dynamics of the reduction enzymes . Soil Biol. Biochem. , 30 : 359 – 367 .
- Granli , T and Bøckman , OC . 1994 . Nitrous oxide from agriculture . Norwegian J. Agri. Sci. , Suppl. 12 : 1 – 128 .
- Gregorich , EG , Drury , CF , Ellert , BH and Liang , BC . 1997 . Fertilization effects on physically protected light fraction organic matter . Soil Sci. Soc. Am. J. , 61 : 482 – 484 .
- Gupta , VVSR and Germida , JJ . 1988 . Distribution of microbial biomass and its activity in different aggregate size classes as affected by cultivation . Soil Biol. Biochem. , 20 : 777 – 786 .
- Hadar , H , Asher , BT and Guy , T . 2010 . Effect of manure and cultivation on CO2 and N2O emission from a corn field under Mediterranean conditions . Soil Sci. Soc. Am. J. , 39 : 437 – 448 .
- Hadas , A , Parkin , TB and Stahl , PD . 1998 . Reduced CO2 release from decomposing wheat straw under N-limiting conditions: Simulation of carbon turnover . Eur. J. Soil Sci. , 49 : 487 – 494 .
- Hattori , T . 1988 . Soil aggregates as microhabitats of microorganisms . Rep. Inst. Agric. Res. Tohoku Univ. , 37 : 23 – 36 .
- Intergovernmental Panel on Climate Change (IPCC) . 2007 . Fourth Assessment Report (AR4), Working Group I: The physical Science Basis , Cambridge : Cambridge University Press .
- IUSS Working Group WRB, 2006: World Reference Base for Soil Resources 2006.World Soil Resources Reports 103, FAO, Rome
- Janzen , HH . 2004 . Carbon cycling in earth systems-a soil science perspective . Agric. Ecosyst. Environ. , 104 : 399 – 417 .
- Jastrow , JD , Boutton , TW and Miller , RM . 1996 . Carbon dynamics of aggregate-associated organic matter estimated by carbon-13 natural abundance . Soil Sci. Soc. Am. J. , 60 : 801 – 807 .
- Jenkinson , DS . 1990 . The turn over of the organic carbon and nitrogen in soil . Phil. Trans. R. Soc. Lond. B , 329 : 361 – 368 .
- Jensen , LS , Mueller , T , Magid , J and Nielsen , NE . 1997 . Temporal variation of C and N mineralisation, microbial biomass, and extractable organic pools in soils after oilseed rape straw incorporation in the field . Soil Biol. Biochem. , 29 : 1043 – 1055 .
- Jin , T , Shimizu , M , Marutani , S , Desyatkin , AR , Iizuka , N , Hata , H and Hatano , R . 2009 . Effect of chemical fertilizer and manure application on N2O emission from reed canary grassland in Hokkaido, Japan . Soil Sci. Plant Nutr. , 56 : 53 – 65 .
- Jones , SK , Rees , RM , Skiba , UM and Ball , BC . 2007 . Influence of organic and mineral N fertiliser on N2O fluxes from a temperate grassland . Agr. Ecosyst. Environ. , 121 : 74 – 83 .
- Kester , RA , Deboer , W and Laanbroek , HJ . 1997 . Production of NO and N2O by pure cultures of nitrifying and denitrifying bacteria during changes in aeration . Appl. Environ. Microbiol. , 63 : 3872 – 3877 .
- Khalil , K , Renaulta , P and Mary , B . 2005 . Effects of transient anaerobic conditions in the presence of acetylene on subsequent aerobic respiration and N2O emission by soil aggregates . Soil Biol. Biochem. , 37 : 1333 – 1342 .
- Lefelaar , PA . 1993 . Water movement, oxygen supply and biological processes at the aggregate scale . Geoderma. , 57 : 143 – 165 .
- Liang , BC , Gregorich , EG , Schnitzer , M and Voroney , RP . 1996 . Carbon mineralisation in soils of different textures as affected by water-soluble organic carbon extracted from composted dairy manure . Biol. Fertil. Soil. , 21 : 10 – 16 .
- Linn , DM and Doran , JW . 1984 . Effect of water-filled pore space on carbon dioxide and nitrous oxide production in tilled and non-tilled soils . Soil Sci. Soc. Am. J. , 48 : 1267 – 1272 .
- Linquist , BA , Singleton , PW , Yost , RS and Cassman , KG . 1997 . Aggregate size effects on the sorption and release of phosphorus in an Ultisol . Soil Sci. Soc. Am. J. , 61 : 160 – 166 .
- Lipschultz , F , Zafiriou , OC , Wofsy , SC , McElroy , MB , Valois , FW and Watson , SW . 1981 . Production of NO and N2O by soil nitrifying bacteria . Nature. , 294 : 641 – 643 .
- Livesley , SJ , Kiese , R , Miechle , P , Weston , CJ , Butterbach-Bahl , K and Arndt , SK . 2009 . Soil atmosphere change of greenhouse gases in a Eucalyptus marginata woodland, a clover grass pasture, and pinus radiata and Eucalyptus globules plantation . Global Change Biol. , 15 : 425 – 440 .
- Martens , R . 1995 . Current methods for measuring microbial biomass C in soil: Potentials and limitations . Biol. Fertil. Soil. , 19 : 87 – 99 .
- Martin , J and David , MB . 1996 . Temperature and moisture effects on the production of dissolved organic carbon in a Spodosol . Soil Biol. Biochem. , 28 : 1191 – 1199 .
- Mori , T , Ohta , S , Ishizuka , S , Konda , R , Wicaksono , A , Heriyanto , J and Hardjono , A . 2010 . Effects of phosphorus addition on N2O and NO emissions from soils of an Acacia mangium plantation . Soil Sci. Plant Nutr. , 56 : 782 – 788 .
- Nishina , K , Takenaka , C and Ishizuka , S . 2009 . Relationship between N2O and NO emission potentials and soil properties in Japanese forest soils . Soil Sci. Plant. Nutr. , 55 : 203 – 214 .
- Paul , JA and Beauchamp , EG . 1989 . “ Biochemical changes in soil beneath cattle slurry layer: the effect of volatile fatty acid oxidation on denitrification and soil pH ” . In Nitrogen on Organic Wastes Applied to Soils , Edited by: Hansens , JA and Henriksen , K . 261 – 270 . London : Academic Press .
- Prove , BG , Loch , RJ , Foley , JL , Anderson , VJ and Younger , DR . 1990 . Improvements in aggregation and infiltration characteristics of a krasnozem under maize with direct drill and stubble retention . Aust. J. Soil Res. , 28 : 577 – 590 .
- Puget , P , Angers , DA and Chenu , C . 1995 . Total and young organic matter distributions in aggregates of silty cultivated soils . Eur. J. Soil Sci. , 46 : 449 – 459 .
- Renault , P and Stengel , P . 1994 . Modeling oxygen diffusion in aggregated soils: I. anaerobiosis inside the aggregate . Soil Sci. Soc. Am. J. , 58 : 1017 – 1023 .
- Scott , A , Ball , BC , Crichton , IJ and Aitken , MN . 2000 . Nitrous oxide and carbon dioxide emissions from grassland amended with sewage sludge . Soil Use Manage. , 16 : 36 – 41 .
- Semenov , VM , Ivannikova , LA , Semenova , NA , Khodzhaeva , AK and Udal , SN . 2010 . Organic matter mineralization in different soil aggregate fractions . Euro. Soil Chem. , 43 : 141 – 148 .
- Sexstone , AJ , Revsbech , NP , Parkin , TB and Tiedje , JM . 1985 . Direct measurement of oxygen profiles and denitrification rates in soil aggregates . Soil Sci. Soc. Am. J. , 49 : 645 – 651 .
- Shimizu , M , Marutani , S , Desyatkin , AR , Jin , T , Hata , H and Hatano , R . 2009 . The effect of manure application on carbon dynamics and budgets in a managed grassland of Southern Hokkaido, Japan . Agric. Ecosyst. Environ. , 130 : 31 – 40 .
- Shimizu , M , Marutani , S , Desyatkin , AR , Jin , T , Nakano , K , Hata , H and Hatano , R . 2010 . Nitrous oxide emissions and nitrogen cycling in managed grassland in Southern Hokkaido, Japan . Soil Sci. Plant Nutr. , 56 : 676 – 688 .
- Sirwan , Y , Roy , MH , Goulding , KWT and Webster , CP . 1997 . N2O, NO And NO2 fluxes from a grassland: Effect of soil pH . Soil Biol. Biochem. , 29 : 1199 – 1208 .
- Smith P 2004: Engineered biological sinks on land. In The Global Carbon Cycle: Integrating Humans, Climate, and the Natural World. Eds. Field CB, Raupach MR, pp 479–491. Island Press, Washington, DC
- Smith , MS and Tiedje , JM . 1979 . Phases of denitrification following oxygen depletion in soil . Soil Biol. Biochem. , 11 : 261 – 267 .
- Soil Survey Staff, 2006: Keys to Soil Taxonomy, 10th ed. USDA-Natural Resources Conservation Service, Washington, D.C
- Steven , S , Stefaan , DN , Tamas , N , Tibor , T and Georges , H . 2006 . Effect of fertilizer and manure application on the distribution of organic carbon in different soil fractions in long-term field experiments . Europ. J. Agron. , 25 : 280 – 288 .
- Tiedje , JM . 1994 . “ Denitrifiers ” . In Methods of Soil Analysis, Part 2. Microbiological and Biochemical Properties , Edited by: Weaver , RW . 245 – 267 . Madison, , WI : Soil Science Society of America .
- Tisdall , JM and Oades , JM . 1982 . Organic matter and water-stable aggregates in soils . J. Soil Sci. , 33 : 141 – 163 .
- Toma , Y and Hatano , R . 2007 . Effect of crop residue C: N ration N2O emission from gray lowland soil in Mikasa, Hokkaido, Japan . Soil. Sci. Plant Nutr. , 53 : 198 – 205 .
- Toma , Y , Kimura , SD , Yamada , H , Hirose , Y , Fujiwara , K , Kusa , K and Hatano , R . 2010 . Effect of environmental factors on temporal variation in annual carbon dioxide and nitrous oxide emission from an unfertilized bare field on gray lowland in Mikasa, Hokkaido, Japan . Soil Sci. Plant Nutr. , 56 : 663 – 675 .
- Wang , LF and Cai , ZC . 2008 . Nitrous oxide production at different soil moisture contents in an arable soil in China . Soil Sci. Plant Nutr. , 54 : 786 – 793 .
- Wang , X , Yost , RS and Linquist , BA . 2001 . Soil aggregate size affects phosphorus desorption from highly weathered soils and plant growth . Soil Sci. Soc. Am. J. , 65 : 139 – 146 .
- Wrage , N , Velthof , GL , van Beusichem , ML and Ocnema , O . 2001 . Role of nitrifier denitrification in the production of nitrous oxide . Soil Biol. Biochem. , 33 : 1723 – 1732 .
- Zhang , R and Wienhold , BJ . 2002 . The effect of soil moisture on mineral nitrogen, soil electrical conductivity and pH . Nutr. Cycl. Agroecosys. , 63 : 251 – 254 .
- Zou , XM , Ruan , HH , Fu , Y , Yang , XD and Sha , LQ . 2005 . Estimation of soil labile carbon and potential turnover rates using a sequential fumigation-incubation procedure . Soil. Biol. Biochem. , 37 : 1023 – 1928 .