Abstract
Soil moisture condition is essential to regulate the release of soil carbon from a drained peatland since aerobic microbial activities can be encouraged through oxygen supply associated with dewatering the soil layer while they may be discouraged under too dry conditions. Aiming to characterize the soil moisture condition in a reclaimed tropical peatland, we monitored the volumetric water content at 5 cm depth (θ 5 cm), groundwater level (GWL) and rainfall for 20 months from March 2010 to November 2011 in an oil palm field in Nakhon-Si-Thammarat, Thailand. We also measured the soil water retention curve and the unsaturated hydraulic conductivity (k) for a series of matric potential (h) to simulate the moisture condition monitored in the field by using the Buckingham-Darcy's flux law. During the dry season in 2010, the θ 5 cm consistently stayed lower than 0.35 m3 m–3 with the GWL lower than a depth of 30 cm. In the transition from the dry season to the rainy season in 2010, the GWL rose to the land surface with peaks and dips across the time for about one month with the θ 5 cm increasing toward saturation. During the rainy season where the GWL stayed near or above the land surface, the θ 5 cm remained the field-saturated value of 0.58 m3 m–3 on average, less than the laboratory-saturated value of 0.63 m3 m–3, suggesting the development of a significant amount of entrapped air-phase. Hysteretic behavior in the measured θ 5 cm–GWL relation also supported that the top soil layer refuses to absorb water in wetting processes. The simulated θ 5 cm based on the measured k(h) and soil water retention curves demonstrated that the ease with which the top soil dries during a dry season was due mainly to the low k(h) value in the dried condition, while the slope of the θ(h) curve was so moderate that the soil layer could retain moisture for maintaining liquid water supply to the surface from the dropped GWL. Sensitivity analyses while varying the magnitude of both k(h) and evaporation rate (E) suggested that the k(h) function was more deterministic than the value of E in making the land surface easily dried. As the GWL stayed lower than 30 cm in depth for a total of 187 days out of the year monitored, while surface-ponding conditions took place for 120 days of the year, it was concluded that either the extremely dried condition or the saturated-moisture condition had dominantly occurred in the study site through a year and, thus, there may only be a limited time when soil organic matter near the land surface is in favorable moisture conditions for aerobic decomposition.
Introduction
Tropical peatlands may amount to 11% (441,025 km2) of the global peatland area, probably storing 88.6 Gt of carbon (C) (Page et al. Citation2011), and tropical peatland forests can perform as carbon sinks especially under high rainfall (Satrio et al. Citation2009). The reclamation of a peatland is associated with drainage, causing the release of soil C through the aeration of the peat soil layer. For instance, converting tropical peatland forests to palm production or other types of agricultural lands is likely to release about 15 t C ha−1 y−1 (Fargione et al. Citation2008). The soil C loss from tropical peatlands is unfavorable even for agricultural production itself since it inevitably causes so much land subsidence that additional labor and costs must be imposed on agricultural management. For example, Wosten et al. (Citation1997) estimated in Malaysian peatlands that the subsidence rate of 2 cm yr−1 can be equivalent to the soil C loss of 26.5 t carbon dioxide (CO2) ha−1 yr−1 and insisted on the importance of adopting a drainage system that combines drainage and water conservation.
Soil moisture condition can be one of the essential factors for controlling soil C storage. According to past studies dealing mainly with mineral soils, aerobic soil respiration is likely to have an optimum moisture condition around 50% of field water capacity or water holding capacity (Bowden et al. Citation1998; Gulledge and Schimel Citation1998; Ilstedt et al. Citation2000) and a threshold moisture content below 0.15 m3 m−3 (Xu et al. Citation2004) or 0.05 m3 m−3 (Liu et al. Citation2002). These observations suggest that soil moisture can affect soil organic matter decomposition by regulating the degree of water stress on aerobic microbial activities or by controlling the transport of gaseous oxygen or other substrates for the microbes.
In the case of a reclaimed peatland, its soil moisture condition should have drastically shifted to a drier one mainly through the artificial drainage. And some studies have suggested that, as well as in the case of mineral soils, there may be an optimum moisture condition for decomposing a peat layer. Makiranta et al. (Citation2009) studied the sensitivity of peat soil respiration to water level in afforested peatlands and showed that surface CO2 emission rates became highest with a groundwater level (GWL) of around 60 cm in depth and decreased through further drainage. They also showed that microbial biomass of the surface peat soils decreased with time-averaged GWL, suggesting an adverse effect of the dried condition on aerobic microbial activity, though they did not explicitly refer to specific soil moisture level in their experimental conditions. Berglund and Berglund (Citation2011) controlled GWL both in a laboratory and in lysimeters and estimated greenhouse-gas emissions from peatlands cultivated as grasslands, and they observed the highest CO2 emissions when the water table was positioned at 40 cm in depth with the volumetric water content near the soil surface ranging from 0.60 to 0.70 m3 m−3.
On the other hand, only a limited number of studies have filled in the details of the moisture-dependency of peat soil decomposition and there seems to be no consistent understanding about volume fraction or potential of soil water favorable for decomposing peat soils. For example, Kechavarzi et al. (Citation2010) incubated peat soils and plotted CO2 emission rates from the samples against volumetric water contents. On their figures, however, it seems hard to find trends clear enough to give certain conclusions about moisture-dependency of peat soil decomposition.
One possible reason for the inconsistency among the past studies is that soil moisture conditions in actual fields may range more widely than those probed through laboratory studies. In particular, tropical peatlands in Monsoon Asia should experience a wide range of moisture conditions because there are alternating rainy and dry seasons. With these circumstances, some soil moisture conditions in a dry season might become insufficient for soil microbes to perform actively while another condition under flooding in a rainy season might suppress aerobic microbial activities. Thus, it is necessary to increase knowledge about the soil moisture conditions actually occurring in fields before probing the moisture-dependency of peat soil decomposition.
Another thing to be recognized is the way GWL can control the soil moisture condition near the land surface. Calculating this requires an understanding of soil physical properties, especially water conductivity and moisture characteristics of the peat soils which govern the flow and storage of soil water. Clearer understandings of the mechanism of soil water transport with these properties can give more comprehensive interpretations to the site-specific relationships between water level and peat soil decomposition observed in the past studies.
Considering these things to be known, the authors aimed to characterize soil moisture conditions near the surface of a reclaimed tropical peatland through (1) monitoring the seasonal behaviors of volumetric water content, GWL and rainfall, and (2) simulating the surface moisture condition with varying GWL using hydraulic conductivity and water retention characteristics obtained directly from the peatland. Two main characteristics the authors focused on were (i) what level of moisture content dominantly occurred near the surface of the study site and (ii) what the depths of the groundwater table were and how they can correspond to both the highest and the lowest moisture contents observed in the study field. Sensitivity of the simulated results to physical parameters was also discussed to help characterize the surface moisture condition in a reclaimed peatland.
Materials And Methods
Study sites and monitored terms
The study site is situated in Nakhon-Si-Thammarat (08° 00′ 55.4″N, 100° 04′ 09.6″E), located in the middle of the eastern coastline of the Malay Peninsula, 610 km south of Bangkok, Thailand. The location of this site, including a schematic map, was described in Iiyama and Osawa (Citation2010). Measurement devices were installed in an oil palm field that has been established on a tropical peatland since 2007. The peat layer in the study sites had experienced drainage through open ditches dug to a depth of more than 1.5 m and at intervals of about 14 m. According to our monitoring, every 30 minutes at the study site from 15 March 2010 to 14 March 2011, the mean annual temperature and the annual precipitation were 27.7°C and 2658.6 mm, respectively. The GWL fluctuated from more than 150 cm above the surface to lower than 110 cm below the surface in the monitoring period.
One moisture sensor (Hydra Probe II; Stevens Water Monitoring Systems, Inc.; Portland, Oregon, USA), with a cylindrical sensing region 5.7 cm in length and 3.0 cm in diameter, was horizontally installed between two ditches to evaluate the volumetric water content at 5 cm in depth (θ 5 cm). The sensor was calibrated both in laboratory column and in the study field by comparing its output-voltages with the moisture contents measured gravimetrically. In the calibration protocol, the sensor output responded to the amount of water within its sensing region with almost no regard to the bulk density of soil matrix. Rainfall events were monitored using a tipping bucket rain gauge. The GWL was measured by a submersible pressure transducer (CS455; Campbell Scientific; Logan, UT, USA) set in an observation well down to 112 cm in depth. All of these devices were regulated by a data logger (CR1000; Campbell Scientific; Logan, UT, USA).
The physical properties of the soils in the sites are listed in . The organic matter content was gravimetrically evaluated from the ignition loss of the soil sample using a simple muffle furnace (AMF-20N; Asahi Rika Seisakusho; Chiba, Japan) for burning the sample at 800°C for 24 h. The organic C content was measured using a total organic carbon analyzer (TOC-Vcsh; Shimadzu Corp.; Kyoto, Japan) combined with a solid-sample module (SSM-5000A; Shimadzu Corp.; Kyoto, Japan) to oxidize organic matter by catalytic combustion. The soil particle density was measured on the basis of Japan industrial standards (JIS) A 1202 using Gay-Lussac-type pycnometers. The dry bulk density was determined by the gravimetric method through drying the soil cores of 50 cm3 at 105°C for 24 h.
Table 1. Physical properties found in the soil layers in the study site. The top layer (0–60 cm in depth) was more brightly colored with smaller amount of organic matter content and higher bulk density
To measure the soil hydraulic conductivity (k) for a series of matric potential (h), the undisturbed soil cores were sampled using stainless steel tubes. The dimensions of a stainless steel tube were 11.3 cm in inner diameter, 5 cm in height and 0.1 cm in thickness, and three samples were taken from the top 60 cm in depth (). Stainless steel tubes of 50 cm3, 5 cm in diameter and 2.54 cm in height were used to take supplemental samples with three replicates for the measurements of the soil water retention curve [θ(h)]; the volumetric water content θ as a function of (h) of the top 60 cm layer.
Measurements of k ( h ) and θ ( h ) to interpret the θ 5 cm–GWL relation monitored in the field
The k(h) function of an undisturbed sample was obtained using the transient water flow under a drying process with the wind and hot-air methods (Arya Citation2002). A sample was saturated for 24 h in a laboratory with a room temperature of 25°C and then exposed to evaporation. The sample was weighed repeatedly during the drying process and the evaporation rate from the soil sample was evaluated as the decrement of the mass of the sample per cross-sectional area during each time interval of weighing. The vertical profile of h in the sample was monitored by using five sets of porous cups 2 mm in diameter and 4 cm in length connected to pressure transducers (HTVN-100 kPa; Sensez Co. Ltd.; Kokubunji, Tokyo, Japan) regulated by a data logger (CR23X; Campbell Scientific; Logan, UT, USA), and hydraulic gradients were evaluated during the evaporation process. By assuming that the upward water flux is uniform in depth during each time interval of weighing, the k(h) for a specific time interval was evaluated by dividing the evaporation rate by the hydraulic gradient observed between any pair of the five tensiometers. Samples underwent shrinkage in the process of drying, causing gaps between the soil matrix and the wall of the stainless steel tube. When the gaps were found, they were filled with a sealing compound (NEO SEAL B-3, Nitto Chemical Industry Co., Ltd.; Osaka, Japan) to minimize the amount of water vapor circumventing the soil matrix during measurement. The measurements were continued as long as the outputs of the tensiometers indicated the hydraulic gradients as upward.
The θ(h) of the sample was obtained in parallel with the k(h) measurement. A weighing of the sample corresponded to the determination of the volumetric water content (θ) while the h of the sample at the time of weighing was evaluated as an average of the values from the five pressure transducers. To validate the plausibility of the θ(h) curve obtained in the k(h) measurement, we measured the θ(h) curves of the three sub-samples of 50 cm3 by implementing the hanging-water column (HWC) and the pressure-plate (PP) methods (Dane and Hopmans Citation2002).
Simulation of θ 5 cm–GWL relation based on k ( h ) and θ ( h )
To simulate the θ 5 cm–GWL relation observed in the study site, we assumed a quasi-steady-state upward capillary water flow with a given set of k(h) and θ(h) curves. The upward capillary water flow from a shallow groundwater table had been analyzed in classical studies theoretically (Gardner Citation1958), experimentally in laboratories (Gardner and Fireman Citation1958) and in fields (Wind Citation1959). Also, Gardner's theoretical work has been generalized to a broader range of k(h) functions (Warrick Citation1988) to give concise and effective analytical solutions for determining the depth profile of h or the possible maximum evaporation rate under a series of evaporative demands from the atmosphere. When a steady-state upward water flux exists on the shallow groundwater table without water uptake via plant roots or any other kinds of soil water sinks, Buckingham-Darcy's equation may give such a differential equation as follows:
One possible k(h) function that can give an analytical solution to Equation (Equation1) is
To evaluate h at the depth where the moisture sensor was installed, z = −5 cm should be substituted into Equation (Equation3). The resultant equation, h 5 cm, enables the simulation of a series of moisture conditions at z = −5 cm by varying the GWL. And for the GWL > −5 cm, as sometimes observed in the study site, we assumed h 5 cm= 0 to indicate the water-saturated condition. These concepts may be represented by the equation that follows:
Equation (Equation4) gives the h 5 cm–GWL relation with the parameters k s and a which were identified by fitting Equation (Equation2) to the measured k(h) data sets. Then, the h 5 cm–GWL relation was translated into the θ 5 cm–GWL relation by using the θ(h). The simulated θ 5 cm–GWL relation was compared with the measured θ 5 cm–GWL relation. Several values in E were tried in the simulations since we did not directly measure the evaporative demands at the study site.
Results
Seasonal behaviors of θ 5 cm, GWL and rainfalls
shows the seasonal behaviors of θ 5 cm, GWL and precipitation. From March to June in 2010, the GWL lowered from 50 cm to 110 cm in depth. Although sporadic rainfalls were observed during this dry season, their intensities were insufficient to remarkably increase the θ 5 cm. The θ 5 cm during this dry season stayed lower than 0.35 m3 m−3 and responded weakly or independently to the change in the GWL.
Figure 1. Seasonal behaviors of (a) precipitation, (b) volumetric water content at 5 cm in depth, and (c) groundwater level relative to the land surface from March 2010 to November 2011.
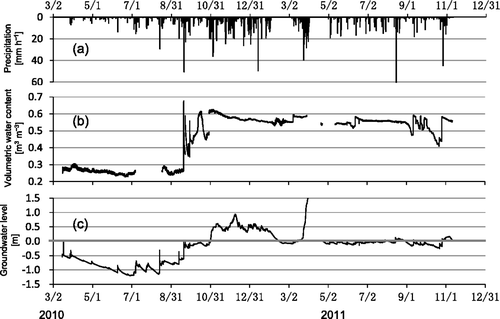
From the second half of September through the first half of October 2010, the GWL rose to within 10 cm of the land surface, and after these irregular fluctuations, the θ 5 cm increased to more than 0.60 m3 m−3, suggesting that the entire soil profile reached a field-saturated condition. From the beginning of November 2010 to March 2011, the θ 5 cm gradually decreased from 0.62 m3 m−3 to 0.55 m3 m−3. Since the GWL during this term had stayed above the land surface, this implied that air bubbles had developed in the top soil layer, presumably due to anaerobic microbial activities.
After April 2011, rainfalls occurred frequently and the GWL resided near or above the land surface even after the dry season began. Consequently, the θ 5 cm stayed between 0.53 m3 m−3 and 0.57 m3 m−3 by the end of August 2011. The data sets from March to May 2011 were unavailable because an extreme flood invaded this region and the measurement devices were temporarily evacuated. If floods like the observed one had been taking place yearly, the top soil layer might have been exposed to alluvial matter. The exposure to alluvial matter can be a more likely cause of the relatively low C content in the topsoil () than aerobic decomposition of soil organic matter because the oil palm plantation has been there since 2007 and, thus, the three-year-old plantation was unlikely to cause the soil C content in the entire top layer to drop from more than 80% to less than 10%. The continuous monitoring of the GWL with other weather parameters will make it clear whether large floods are generally happening in the study site.
In summation of the seasonal monitoring, a clear distinction between dry and rainy seasons made the moisture condition around the land surface either severely dry or water-saturated through the year, and the intermediate of the two extreme moisture conditions appeared for about one month in the transition between the two seasons. In fact, the GWL stayed lower than 30 cm in depth for 187 d in total for one year from March 2010. On the other hand, the moisture sensor was under field-saturated conditions for 120 d of the year.
Modeling the k ( h ) and the θ ( h ) functions
shows the results of the k(h) measurements while the plots in draw the θ(h) obtained through the k(h) measurements (△) and the HWC and the PP methods (▪). In , the dotted line and the chain line are drawn for discussing the sensitivity of the soil moisture condition to the modeled k(h) curve in the later section. These two examples of ks were manually selected so that most of the measured k(h) plots were included in the two modeled curves, specifically so that the simulation with ks = 3 × 10−2 cm s−1 represented the possible maximum water conductivity condition and that with 3 × 10−5 cm s−1 was regarded as the possible minimum one.
Figure 2. (a) Shows the plots from the triplicate k(h) measurements for the soil samples taken from the top 60-cm layer while the solid line, the dotted line, and the chain line denote the modeled curves of k(h) = ks /(1 + h/a) with ks = 6 × 10−4, 3 × 10−2 and 3 × 10−5 cm s−1, respectively. The parameter a = −0.04 is consistently used. (b) Soil water retention curves obtained from the k(h) measurements (△) and from the hanging-water-column (HWC) and the pressure-plate (PP) methods (▪). Horizontal bars attached to the plots of △ denote the one standard error below and above the data plots among the h values recorded by the five tensiometers. Vertical bars drawn on the plots of ▪ indicate one standard error below and above the data plots for the triplicates of the HWC and the PP methods. The dotted line is the van Genuchten model θ = θr + (θ s−θr )(1 + α|h| n )−1+1/ n (van Genuchten Citation1980) with the parameters of θ s = 0.63 m3 m−3, θr = 0.26 m3 m−3, α = 0.01, and n = 1.4.
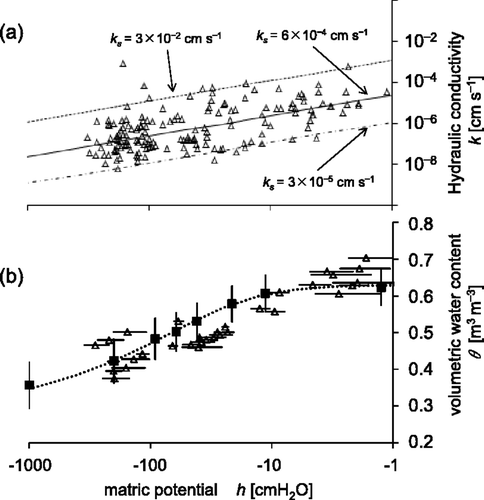
In , a single curve of Equation (Equation2) with the parameters k s = 6 × 10−4 cm s−1 and a = −0.04 cm−1 represented all the plots (the solid line in ) and, thus, this single curve was used to identify h 5 cm for a series of GWL by using Equation (Equation4). In , the θ(h) plots obtained through the k(h) measurements formed an almost identical domain to those obtained from the HWC and the PP measurements except for the near-saturated condition in which the latter group had the saturated volumetric water content of 0.63 m3 m−3 while the former seemed to have slightly higher values in θ with the maximum of 0.70 m3 m−3 among the observed values. Thus, as a first approximation, the mean θ(h) curve for the HWC and the PP measurements was used for translating the simulated h 5 cm–GWL relation in Equation (Equation4) into the θ 5 cm–GWL relation. For this simulation procedure, the θ(h) curve was expressed by the van Genuchten equation (van Genuchten Citation1980):
Measured and simulated θ 5 cm–GWL relation
describes the θ 5 cm plotted against the GWL. The measured plots were differentiated into two groups represented by circle (○) and plus (+) symbols. The former group denotes the plots obtained while the GWL was going downward while the latter represents those observed while the GWL was rising. includes only the data points observed when the GWL continuously moved in one specific direction for at least one hour straight, and therefore excluded any momentarily-recorded peaks of either the θ 5 cm () or the GWL (). The four solid lines indicate the simulated curves based on Equation (Equation4) with the parameters specified in . The upward water flux E was varied from 2 to 4, 6, and 8 mm d−1. The simulated curves were extrapolated as the dotted lines into the matric potential domain of h < −1000 cm where neither the k(h) nor the θ(h) was measured, and were ended at GWL = −60 cm because the k(h) measurements made sense only for the top 60 cm layer in the study site.
Figure 3. The θ 5cm–GWL relation based on the measured data sets on . Symbols of circle (○) and plus (+) denote the plots obtained while the groundwater table was going downward and upward, respectively. Solid lines show the simulated curves based on Equation (Equation4) with the parameters specified in . The upward water flux E in Equation (Equation4) was varied from 2 to 4, 6 and 8 mm d−1. The simulated curves were extrapolated into the matric potential domain of h < −1000 cm where the k(h) was not measured (dotted lines), and were terminated at GWL = −60 cm since both the k(h) and the θ(h) were evaluated only for the top 60 cm layer.
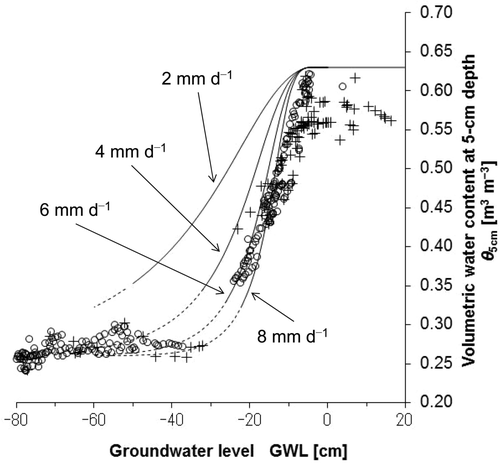
Among the simulated θ 5 cm–GWL relations, the curve with E = 6 and 8 mm d−1 were seemingly promising to reproduce the measured θ 5 cm–GWL relation while the curves with E = 2 and 4 mm d−1 overestimated the measured plots. In addition, since E = 8 mm d−1 may be equivalent to the annual evaporation of 2920 mm that obviously exceeds the observed rainfall of 2658.6 mm, and the gradient of the curve with E = 8 mm d−1 in seemed too steep for the GWL > −30 cm to reproduce the measured plots, we adopted the curve with E = 6 mm d−1 as the reference model to interpret the measured θ 5 cm–GWL relation in the following discussion.
The measured θ 5 cm–GWL relation was roughly divided into three GWL domains. For the GWL shallower than −25 cm, the measured θ 5 cm decreased from around 0.6 m3 m−3 under field-saturated conditions to around 0.35 m3 m−3 with the lowering of GWL. Then, only a small number of the measured plots on the θ 5 cm–GWL relation was found for the GWL around −30 cm, because there were few chances that the GWL stayed in this depth range (). When the GWL lowered its position to deeper than 30 cm in depth, the measured θ 5 cm reached below 0.30 m3 m−3 and the change in θ 5 cm was almost negligible for the further lowering of the GWL.
Discussion
Incomplete saturation and moisture hysteresis in the rainy season
suggested that, in the rainy season, the top soil layer rarely became completely saturated even under surface-ponding conditions because the θ 5 cm observed under field-saturated conditions was 0.58 m3 m−3 on average and was lower than the saturated volumetric water content of 0.63 m3 m−3 obtained by the HWC and the PP methods. The simulated curve with E = 6 mm d−1 also tended to deviate to the higher side in water content from the measured plots in the range of GWL≧ −15 cm. Since organic soils tend to repel water more remarkably than other types of soils when wetting (Lachacz et al. Citation2009; Rasa et al. Citation2007), the water-repellent properties may explain the θ 5 cm lower than the laboratory-saturated water content under field-saturated conditions. Additional evidence of difficulty in saturating the soil was found as the moisture hysteresis in wetting and drying of the soil. shows that, particularly for the GWL shallower than 10 cm in depth, the observed θ 5 cm for the GWL values going downward (○) tended to be larger than those measured under wetting processes (+).
Another possible reason for the field-saturated θ 5 cm being lower than the laboratory-saturated one was the anaerobic microbial activities producing some gas species under field-saturated conditions. As shown in , the moisture sensor indicated a gradual decrease in the θ 5 cm even when the water table stayed above the land surface from November 2010 to March 2011, suggesting the growth of the air-phase under the field-saturated condition. One of the gas species possibly produced under anaerobic conditions is methane (CH4). CO2 may also exist under the anaerobic condition. However, part of the CO2 would be consumed as a substrate to anaerobically produce CH4. In addition, the solubility of CH4 is one-twentieth at 40°C and one-thirtieth at 0°C of that of CO2. Therefore, CH4 is likely to be more important than CO2 as the source of air bubbles. Certainly, the concentration of CH4 in the soil gaseous phase can become as high as 1000 ppm in reclaimed tropical peatlands as Melling et al. (Citation2005) observed under an oil palm plantation. On the other hand, however, presumably due to the substrate quality of tropical peats which are rich in polyphenols like lignin and are not easily decomposable, CH4 emissions from tropical peatlands may not be as remarkable as those from boreal or temperate peatlands (Couwenberg et al. Citation2010). Thus, the evidence of the possible gas accumulation in the study site and the types of gas species should be confirmed through capturing the burst of gas from the land surface at the moment when ponding-water recedes and the soil layer are becoming unsaturated again.
The inhibiting factors for saturating soil may explain the reason why the simulated curves in overestimated the measured θ 5 cm as the GWL got close to the land surface. Thus, further studies will require the models of k(h) and θ(h) to be based on both wetting and drying processes of peat soil samples to take into account the effect of the hysteresis in soil moisture characteristics. In addition, the growth of air bubbles in a saturated peat layer may become another prominent factor to define the ratio of soil air-phase under a field-saturated condition, though it is difficult to specify which of these factors can be dominant to make the models of the physical properties more effective to explain actual soil moisture behaviors.
Surface soil subject to severe drying in a dry season
During most of the dry season, the soil near the land surface was in severely dried conditions as the measured θ 5 cm stayed below 0.35 m3 m−3 (). This dried condition near the land surface appeared as soon as the GWL dropped below 30 cm in depth. The main reason why the surface soil was subject to drying even with minor intensity of drainage was likely that the slope of the θ(h) curve was quite moderate in the dried condition while the k(h) value decreased three to four orders lower than that under the saturated condition. We discuss this point in the next paragraph using the k(h) and the θ(h) in , concurrently confirming the assumption adopted when applying Equation (Equation1) that a steady-state upward water flux exists on the shallow groundwater table.
indicates that the measured θ 5 cm dropped to 0.35 m3 m−3 while the GWL decreased to 25 cm in depth. When the θ decreases to 0.35 m3 m−3, the h can reach −1000 cm according to the simulated θ 5 cm–GWL relation with E = 6 mm d−1. The k(h) curve modeled in yields the k(h) values of 2.4 × 10−8 cm s−1 and 1.2 × 10−7 cm s−1 for h = −1000 cm and −203 cm, respectively. Thus, the hydraulic gradient that should appear under the steady evaporation with E = 6 mm d−1 = 6.9 × 10−6 cm s−1 in this h-domain must be E/k = 58 ∼ 290. On the other hand, the measured θ(h) curve in gives θ of 0.356 and 0.420 for h = −1000 cm and −203 cm, respectively, meaning that dθ/dh is as moderate as 8.0 × 10−5 in this h-domain. Therefore, a hydraulic gradient of 58 ∼ 290 can be achieved with the moisture gradient in the range of 0.0046 ≦ dθ/dz ≦ 0.023 m3 m−3 cm−1. This moisture characteristic suggests that, in the dried condition, even a faint moisture gradient can cause as large a hydraulic gradient as that realized the steady upward water flux under the evaporative demand of E = 6 mm d−1, giving a reason for supporting the assumption that Equation (Equation1) holds true. Consequently, the water conductivity of the study field can reduce three to four orders lower than that in the saturated condition with even a minor intensity of drainage, while the moisture condition allows the soil layer to maintain the upward liquid water flow.
Combining the above discussion and the monitored fact that the GWL stayed lower than −30 cm for 187 d out of one year from March 2010, we concluded that the severely dried condition can easily occur in the topsoil of the study site for the major part of a dry season, owing to the moderate slope of the θ(h) curve and the low k(h) value in the dried condition associated with the dropped GWL.
Sensitivity of the h 5 cm–GWL relation to soil water conductivity and evaporative demand
illustrates the results of the sensitivity of the h 5 cm–GWL relation to the k(h) curves by applying the three k(h) curves modeled in . For the three simulations, the upward water flux E = 6 mm d−1 was equally assigned. suggested that when a soil layer has a k(h) 50 times larger than the representative one in , it can keep its land surface wetted with h > −100 cm even for a GWL lower than −80 cm. On the other hand, a soil layer with a k(h) one-twentieth of the representative one can become rapidly dried to h < −106 cm before the GWL goes down by a few centimeters. Thus, it was suggested that the shape of the k(h) curve can have such a strong impact on defining the moisture condition in the topsoil that the possible maximum and minimum k(h) curves can give two extreme cases in the h 5 cm–GWL relations and, thus, it seemed better to replicate as many directly measured properties as possible in each study field to allow estimating the extent to which the model prediction can deviate from an actual solution.
Figure 4. (a) The sensitivity of the h 5cm–GWL relation to the modeled k(h) curve. The three modeled k(h) curves displayed in were used. The upward water flux E of 6 mm d−1 was consistently adopted in all three simulations. (b) The transition of the h 5cm–GWL relation with varying the upward water flux E. All three curves share the same k(h) = ks /(1 + h/a) with ks = 6 × 10−4 cm s–1 and a = –0.04, and the chain line denotes E = 10 mm d–1 while the two-dot chain line represents E = 2 mm d–1.
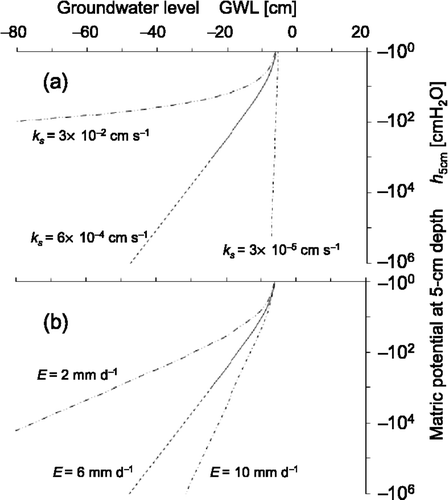
shows the sensitivity of the h 5 cm–GWL relation to the evaporative demand on the land surface. All the simulated curves consistently used Equation (Equation2) with ks = 6 × 10−4 cm s−1 and a = −0.04. The two curves simulating the steady-state upward water fluxes of E = 10 mm d−1 (chain line) and 2 mm d−1 (two-dot chain line) were compared with the curve for E = 6 mm d−1 so that the two additional curves include most cases of E that may occur in the field. The three curves indicate that the GWLs corresponding to the h 5 cm = −1000 cm are −17.6, −24.3, and −51.8 cm for E = 10, 6, and 2 mm d−1, respectively, suggesting that the top soil layer in the study site is subjected to drying for the GWL ordinarily found in a dry season regardless of the level of E. A comparison of the curves in with those in also implies that E seems to have less impact on the h 5 cm–GWL relation than k(h) and, thus, the low water conductivity of the soil layer is a more essential factor for the top soil layer to be prone to drying.
Conclusions
In order to characterize the soil moisture condition near the surface of a reclaimed tropical peatland, the seasonal behaviors of θ 5 cm, GWL and rainfall were monitored.
The seasonal monitoring showed that either severely dried or water-saturated conditions had dominantly occurred through alternating dry and rainy seasons in the study field. The intermediate moisture conditions appeared only for about one month between the two extreme conditions. Correspondingly, the GWL stayed lower than 30 cm in depth for a total of 187 d out of the year monitored, while the surface ponding condition had occurred for 120 d of the year.
During the surface ponding condition in the rainy season, the time-averaged θ 5 cm under field-saturated conditions was 0.58 m3 m−3. This value was lower than the saturated volumetric water content of 0.63 m3 m−3 obtained in the laboratory. The hysteretic behavior on the θ 5 cm–GWL relation, probably due to water repellency of the highly organic soil, and the growth of air-phase volume were observed, both of which also suggested that the soil layer in the study field tends to entrap a substantial volume of air bubbles while the GWL is rising or stays above the land surface.
The θ 5 cm–GWL relation was simulated using the modeled k(h) and θ(h) functions to characterize the surface moisture condition, especially to explain that the top soil layer is prone to drying, with varying GWL. We concluded that the ease of drying of the top soil layer was attributable to the low k(h) value in the dried condition while the moderate slope of the θ(h) curve allowed the soil layer to keep liquid water supply to the surface from the dropped GWL.
Sensitivity analyses of the h 5 cm–GWL relation to k(h) demonstrated that the shape of the k(h) curve was a strong factor for predicting the moisture condition in the topsoil. Thus, in addition to the pursuit of precision in determining k(h), the degree to which the application of the evaluated k(h) can let a predicted moisture condition deviate from an actual field condition should be prescribed as clearly as possible.
The sensitivity of the h 5 cm–GWL relation to the E suggested that the top soil layer in the study site is subjected to drying for the GWL ordinarily found in a dry season regardless of the level of E. The comparison between the sensitivity on k(h) and that on E implied that E seems to have less impact on the h 5 cm–GWL relation than k(h).
Because the severely dried and the near-saturated moisture conditions had dominantly occurred in the study site through a year, there may be only a limited length of time when soil organic matter near the land surface is in a favorable moisture condition for aerobic decomposition. So far, however, the nature of the soil moisture-aerobic decomposition relationship is largely unknown among tropical peat soils. Therefore, in parallel with accumulating knowledge about actual and/or predicted soil moisture conditions in fields, further studies should be encouraged to clarify the features and the ranges of optimum moisture level for aerobic decomposition of tropical peat soils.
Acknowledgments
This study was partly supported by the Japan Science and Technology Agency (JST) under the research funding program “CREST (Core Research of Evolutional Science & Technology)”.
References
- Arya , LM . 2002: Wind and hot-air methods. In Methods of Soil Analysis: Part 4, Physical Methods. SSSA Book Ser. 5, Eds. Dane JH, Topp GC, pp. 916–926. Soil Science Society of America, Inc., Madison, WI
- Berglund , O and Berglund , K . 2011 . Influence of water table level and soil properties on emissions of greenhouse gases from cultivated peat soil . Soil Biol. Biochem. , 43 : 923 – 931 .
- Bowden , RD , Newkirk , KM and Rullo , GM . 1998 . Carbon dioxide and methane fluxes by a forest soil under laboratory-controlled moisture and temperature conditions . Soil Biol. Biochem. , 30 : 1591 – 1597 .
- Couwenberg , J , Dommain , R and Joosten , H . 2010 . Greenhouse gas fluxes from tropical peatlands in south-east Asia . Global Change Biol. , 16 : 1715 – 1732 .
- Dane , JH and Hopmans , JW . 2002: Water retention and storage. In Methods of Soil Analysis: Part 4, Physical Methods. SSSA Book Ser. 5, Eds. Dane JH, Topp GC, pp. 680–683, 688–690. Soil Science Society of America, Inc., Madison, WI
- Fargione , J , Hill , J , Tilman , D , Polasky , S and Hawthorne , P . 2008 . Land clearing and the biofuel carbon debt . Science , 319 : 1235 – 1238 .
- Gardner , WR . 1958 . Some steady-state solutions of the unsaturated moisture flow equation with application to evaporation from a water table . Soil Sci. , 85 : 228 – 232 .
- Gardner , WR and Fireman , M . 1958 . Laboratory studies of evaporation from soil columns in the presence of a water table . Soil Sci. , 85 : 244 – 249 .
- Gulledge , J and Schimel , JP . 1998 . Moisture control over atmospheric CH4 consumption and CO2 production in diverse Alaskan soils . Soil Biol. Biochem. , 30 : 1127 – 1132 .
- Iiyama , I and Osawa , K . 2010 . Surface O2 influx related to soil O2 profile in a drained tropical peatland . Soil Sci. Plant Nutr. , 56 : 517 – 520 .
- Ilstedt , U , Nordgren , A and Malmer , A . 2000 . Optimum soil water for soil respiration before and after amendment with glucose in humid tropical acrisols and a boreal mor layer . Soil Biol. Biochem. , 32 : 1591 – 1599 .
- Kechavarzi , C , Dawson , Q , Bartlett , M and Leeds-Harrison , PB . 2010 . The role of soil moisture, temperature and nutrient amendment on CO2 efflux from agricultural peat soil microcosms . Geoderma , 154 : 203 – 210 .
- Lachacz , A , Nitkiewicz , M and Kalisz , B . 2009 . Water repellency of post-boggy soils with a various content of organic matter . Biologia , 64 : 634 – 638 .
- Liu , X , Wan , S , Su , B , Hui , D and Luo , Y . 2002 . Response of soil CO2 efflux to water manipulation in a tallgrass prairie ecosystem . Plant Soil , 240 : 213 – 223 .
- Makiranta , P , Laiho , R , Fritze , H , Hytonen , J , Laine , v and Minkkinen , K . 2009 . Indirect regulation of heterotrophic peat soil respiration by water level via microbial community structure and temperature sensitivity . Soil Biol. Biochem. , 41 : 695 – 703 .
- Melling , L , Hatano , R and Goh , KJ . 2005 . Methane fluxes from three ecosystems in tropical peatland of Sarawak, Malaysia . Soil Biol. Biochem. , 37 : 1445 – 1453 .
- Page , S , Rieley , JO and Banks , CJ . 2011 . Global and regional importance of the tropical peatland carbon pool . Global Change Biol. , 17 : 798 – 818 .
- Rasa , K , Horn , R , Raty , M , Yli-Halla , M and Pietola , L . 2007 . Water repellency of clay, sand and organic soils in Finland . Agric. Food Sci. , 16 : 267 – 277 .
- Satrio , AE , Gandaseca , S , Ahmed , OH and Majid , NMA . 2009 . Effect of precipitation fluctuation on soil carbon storage of a tropical peat swamp forest . Am. J. Appl. Sci. , 6 : 1484 – 1488 .
- Shokri , N and Salvucci , GD . 2011 . Evaporation from porous media in the presence of a water table . Vadose Zone J. , 10 : 1309 – 1318 .
- van Genuchten , MT . 1980 . A closed-form equation for predicting the hydraulic conductivity of unsaturated soils . Soil Sci. Soc. Am. J. , 44 : 892 – 898 .
- Warrick , AW . 1988 . Additional solutions for steady state evaporation from a shallow water table . Soil Sci. , 146 : 63 – 66 .
- Wind , GP . 1959 . A field experiment concerning capillary rise of moisture in a heavy clay soil . Neth. J. Agric. Sci. , 3 : 60 – 69 .
- Wosten , JHM , Ismail , AB and van Wijk , ALM . 1997 . Peat subsidence and its practical implications: a case study in Malaysia . Geoderma , 78 : 25 – 36 .
- Xu , L , Baldocchi , DD and Tang , J . 2004 . How soil moisture, rain pulses, and growth alter the response of ecosystem respiration to temperature . Glob. Biogeochem. Cycles , 18 : 1 – 10 .