Abstract
Aggregate slaking is linked with rapid pressure buildup within aggregates. Soil water repellency may help hamper the pressure buildup within aggregates by reducing their wetting rates. We examined the effects of animal manure in improving aggregate stability, the hydrophobic effects of green manure, and the possibility of using organic manure mixtures to increase the aggregate stability for Sri Lankan red yellow podzolic soils using model aggregates. Almost all the cow dung (CD) added samples showed extremely low percentages of water stable aggregates (%WSA) demonstrating rapid destruction of aggregates. Although the addition of ≥ 10% goat dung (GD) improved the %WSA, aggregate floating occurred, showing the risk of aggregate floating with runoff water. Addition of 5% GD would be an acceptable solution if the %WSA can be improved. Casuarina equisetifolia L. leaves (CE) was found to be a hydrophobic green manure. Although addition of ≥ 5% CE increased the %WSA up to about 90%, aggregate floating occurred. The possibility of improving %WSA using 1–2% hydrophobic green manure in organic manures mixtures was tested. Samples with 5% GD + 2% CE manure mixture showed the highest and the most stable %WSA without showing aggregate floating. Additions of compost and poultry litter were found not to be effective in improving aggregate stability with or without CE. Strong or higher water repellency was not observed in any of the samples with manure mixtures, showing that the addition of 1–2% hydrophobic CE would not induce detrimental effects of water repellency. There was no clear correlation between %WSA and the hydrophobicity of soils. However, the %WSA can be considered to show a tendency to increase with increasing hydrophobicity, because the %WSA was very high in samples with hydrophobic CE, the %WSA increased when mixed with 1–2% CE, and samples with highest water drop penetration time (WDPT) among all the manure mixtures showed the highest %WSA.
1. INTRODUCTION
Aggregate stability refers to the ability of soil aggregates to resist disruption when outside forces, which are usually associated with water, are applied (Bossuyt et al. Citation2001; Díaz-Zorita et al. Citation2002; Rillig and Mummey Citation2006). Soil aggregation in natural conditions is related to soil organic matter levels and affects soil biological activity, water infiltration into soils, water holding-capacity and nutrient availability of soils (Six et al. Citation2004; Pirmoradian et al. Citation2005; Nichols and Toro Citation2011). Aggregate stability of soil is generally strongly correlated with soil organic matter (Chaney and Swift Citation1984; Piccolo and Mbagwu Citation1999). Stable aggregates strongly resist slaking and thereby reduce soil erosion.
The slaking process after rapid wetting of aggregates is a key factor controlling the soil structural stability in dry soils. Erosion of highly wettable surface soils linked with slaking and mechanical breakdown of aggregates is a major problem in the low country wet zone (WL) of Sri Lanka. The area is characterized by hot humid climate with rapid decomposition of organic matter, where the predicted increases in temperature and climate instability associated with global change scenarios will intensify the problems related with aggregate disruption and erosion (Özgül et al. Citation2011).
Slaking of aggregates is linked with rapid pressure buildup within aggregates. In general, there are four key processes involved in aggregate deterioration: (1) slaking when dry aggregates are suddenly rehydrated; (2) mechanical breakdown by rain drop impact; (3) mellowing after wetting-drying cycles, and (4) differential swelling/dispersion when the soil is in contact with free water for a prolonged period of time (Le Bissonnais Citation1996; Zaher and Caron Citation2008).
Water-repellent soils, which are also called hydrophobic soils, resist wetting, or demonstrate reduced wetting rates, when water is applied on the surface. Soil hydrophobicity adversely affects infiltration, evaporation, erosion and the hydrologic balance of soils (Wallis et al. Citation1991; Wallis and Horne Citation1992; Feng et al. Citation2001). It reduces the affinity for water and the infiltration capacity of soil aggregates and might be expected to intervene with slaking, which is a key process of aggregate deterioration. Slaking occurs as a result of internal stresses such as trapped and escaping air in soil pores, and rapid release of heat during wetting. By reducing the rate of infiltration into aggregates, hydrophobicity might help hamper the pressure buildup within aggregates, which can be expected to reduce the aggregate disruption by slaking.
Recent studies have demonstrated that water repellency has some beneficial effects towards stabilizing soil aggregates (Cosentino et al. Citation2010), and subcritical or mild water repellency (Tillman et al. Citation1989) in soils can improve the resistance of aggregates against slaking (Eynard et al. Citation2004). According to Goebel et al. (Citation2005), hydrophobic conditions on aggregate surfaces reduce the rate of mineralization of inside soil organic matter and simultaneously enhance the water stability of aggregates. However, in contrast to the significant amount of research that has been carried out on the common detrimental effects of hydrophobicity on soils, the impact of hydrophobic components on improving aggregate stability has been much less investigated. In fact, soil water repellency is a completely new theme in Sri Lanka and no published data is available on hydrophobicity in Sri Lankan soils, or its relation to aggregate stability.
Organic matter has direct impacts on reducing wetting rates or inducing water repellency in soils (Doerr and Thomas Citation2000; Mataix-Solera and Doerr Citation2004; Leelamanie and Karube Citation2007), where the hydrophobic organic matter has greater effect over hydrophilic organic matter. Improving aggregate stability using hydrophobic organic matter is not as simple a process as it appears to be. If aggregates are too hydrophobic, the accumulation of water on the soil surface will make them float, consequently increasing topsoil erosion by aggregate floating with runoff water (Poulenard et al. Citation2001). This is, in fact, in addition to the problems that a highly water-repellent soil would create. Therefore, the addition of hydrophobic organic matter has to be minimized to the greatest extent possible.
A major reason for soil fertility decline in Sri Lanka is the depletion of soil organic matter. Since ancient times, manuring has been considered one of the most important techniques to increase as well as to maintain soil fertility. Organic manure in the form of animal manure, green manure and compost has long been used for agriculture in Sri Lanka without considering their effects in reducing wetting rates, or improving aggregate stability of soils. A unique feature observed in crop cultivation in the up-country of Sri Lanka, where topsoil erosion is a crucial problem, is the use of animal manure at rates of 10–30 t ha–1. Animal manure has become popular among Sri Lankan rural farmers because of its high nutritive value and low cost. Most often, animal manure is available for rural farmers at no cost. Cow dung, goat dung and poultry litter are some of the most common animal manures that are presently in use.
The objectives of this study were to find the effects of animal manure in improving aggregate stability, to identify a hydrophobic green manure that would improve aggregate stability, and to examine the possibility of using hydrophobic green manure in organic manure mixtures to improve the aggregate stability in Sri Lankan red yellow podzolic soils.
2. METHODOLOGY
2.1. Sampling location
Soil was taken from Wilpita natural forest located in the low country wet zone of Sri Lanka. The agro-ecological region (AER) of the area is WL2a, according to the National Atlas of Sri Lanka (Citation2007). The expected annual rainfall of the area ranges from 2400 to 2800 mm. Average maximum temperature ranges from 32 to 35°C, which is recorded during the period of late February to early May. The average minimum temperature ranges from 22 to 24°C, which is usually observed during the period between December and February. This temperature variation in the given periods of the year is a common phenomenon for the entire island. The relative humidity during the daytime ranges from 60 to 75%, and the nighttime values may often reach up to 90% at any time of the year (National Atlas of Sri Lanka Citation2007).
The soil is locally known to be red yellow podzolic soils (Rhodudults). The basic properties of the soil are presented in . Red yellow podzolic soils are the most widespread great soil group in the wet zone of Sri Lanka. Soil reaction is acidic and the cation exchange capacity may vary from 2–15 cmolc kg–1 in surface soils. The texture of the surface soils is sandy or loamy. The texture and the organic matter content (1–2%) in the surface soils are almost the same as reddish brown earth soils (Haplustalfs), the most common soil in the dry zone of Sri Lanka. Compared with reddish brown earth soils which have a slightly acidic soil reaction (pH 5.8–6.5) and a cation exchange capacity of 15–20 cmolc kg–1, red yellow podzolic soils are less fertile with usually weak or moderate structure (crumb or granular).
Table 1 Basic properties of soil
2.2. Sample preparation
Soil was thoroughly air-dried before use and the natural aggregates were broken by gently pressing them with a spatula during drying. Organic manures (animal manure, green manure, and compost) were thoroughly air-dried and ground using a mechanical grinder. Soil and organic manures were sieved separately with a 1-mm sieve.
To find the effects of animal manure in improving aggregate stability, air-dried and sieved cow dung (CD) and goat dung (GD) (research farm, Faculty of Agriculture, University of Ruhuna, Sri Lanka) were mixed with the sieved soil separately at rates of 0, 5, 10, 25, and 50% ().
Table 2 Organic manure percentage and the average bulk density of prepared aggregates ± standard deviation
Casuarina equsetifolia L. (CE) and Gliricidia maculata L. (GM) leaves (Faculty of Agriculture, University of Ruhuna, Sri Lanka) were used to identify a hydrophobic green manure that would improve aggregate stability. Air-dried and sieved green manures were mixed with soil separately at rates of 0, 5, 10, 25, and 50% (). Both CE and GM samples without mixed-in soil (100% CE or GM) were prepared to test the hydrophobicity of the unadulterated materials.
To assess the possibility of using hydrophobic green manure in organic manure mixtures to improve the aggregate stability, goat dung (GD), poultry litter (PL) (research farm, Faculty of Agriculture, University of Ruhuna, Sri Lanka) and compost (CP) (Ruhunu Compost Fertilizer, Ruhunurata Farm, Ranna, Sri Lanka; Ingredients: cattle manure, paddy straw, green manure, poultry litter, coconut coir dust) were used together with CE. Soils with organic manure mixtures were prepared by mixing 1–2% of CE and 5% of either GD, PL or CP with soil (). The organic carbon content, pH and the air-dried moisture contents of the materials are given in .
Table 3 Organic manure percentages in manure mixtures and the average bulk density of prepared aggregates ± standard deviation
Table 4 Basic properties of organic manures
2.3. Preparation of model aggregates
Air-dried soil and organic materials were mixed together to obtain different mixing ratios. The mixtures were uniformly wetted with distilled water and thoroughly mixed with a spatula to achieve a similar consistency. The water contents of the samples varied in a range of 0.20–0.35 kg kg–1, where the highest water content was required for the sample with 50% CE. The prepared soil cakes were carefully filled into plastic cylindrical blocks 23 mm in diameter and 25 mm in height to create model soil aggregates. The blocks were tapped gently to improve the homogeneity of soil mixtures during packing, and care was taken to fill approximately the same amount from each sample into the cylindrical blocks to maintain the homogeneity of the bulk density of aggregates. The bulk density of the aggregates was measured using 10 aggregates for each sample ( and ). The aggregates were removed from the blocks by gently pressing out from one side. To find the effects of animal manure and green manure in improving aggregate stability, measurements were taken 3, 7, 14 and 30 d after the preparation of aggregates. To find the effect of hydrophobic CE in manure mixtures, measurements were taken 3 and 7 d after the preparation.
2.4. Estimation of the percentage of water stable aggregates
Air-dried aggregates, in three replicates, were placed in a 2-mm sieve and the sieve was oscillated up and down in water through a vertical distance of 3 cm at a rate of 20 oscillations per min for a period of 2 min. The percentage of water stable aggregates larger than 2 mm in diameter (%WSA) was measured to represent the stability of aggregates. The aggregates on the sieve (water stable aggregates) and the destroyed aggregates were oven-dried separately and the %WSA was calculated.
2.5. Floating time
Once the sieves prepared for measuring %WSA are immersed in water, aggregates leap up to the water surface as a result of the entrapped air in intra-aggregate pores when the water entry into aggregates is slow enough. The total floating time of each aggregate, which demonstrates floating, was recorded. The total floating time was considered as the time that aggregates remain on water freely without support of the sieve.
2.6. Estimation of hydrophobicity
The water drop penetration time (WDPT) test was used to measure the hydrophobicity or water repellency of the samples. The samples, which were uniformly wetted with distilled water as explained in the preparation of model aggregates, were allowed to air-dry in large petri dishes. About 5 g of air-dried samples, in three replicates, were placed in weighing bottles 30 mm in diameter and height. The bottle was tapped gently to provide homogeneous packing of the sample inside the weighing bottle and to obtain evenly flat surface conditions. The dry bulk density of the sample varied with the height of the sample inside the bottle, which changed corresponding to the amount of added organic matter in the sample (1.4 Mg m–3 in samples without organic matter; 0.5 Mg m–3 in samples with 50% CE).
A drop of distilled water was placed on the surface of each sample using a burette at about 10 mm height. The time taken for the complete penetration of the water drop was recorded using a stopwatch up to a maximum limit of 1 h. Samples with WDPT ≤ 1 s were considered non-repellent, 1 < WDPT < 60 s slightly repellent, 60 < WDPT < 600 s strongly repellent, 600 < WDPT < 3600 s severely repellent, and WDPT ≥ 3600 s extremely repellent (King Citation1981; Chenu et al. Citation2000; Leelamanie et al. Citation2008).
2.7. Experimental conditions
All the experiments were conducted in laboratory conditions at 28 ± 1°C with 75 ± 5% relative humidity.
3. RESULTS AND DISCUSSION
3.1. Effect of animal manure
3.1.1. Percentage of water stable aggregates (%WSA)
The %WSA of CD- and GD-added soils are presented in . In general, the %WSA of soil mixed with CD was lower compared with that of soils mixed with GD. Almost all the CD-added soils indicated low %WSA, showing that the addition of CD did not improve aggregate stability in a considerable manner.
Figure 1 Percentage of water stable aggregates (%WSA) of animal manure-added soils. CD, cow dung; GD, goat dung. Error bars indicate ± standard deviation.
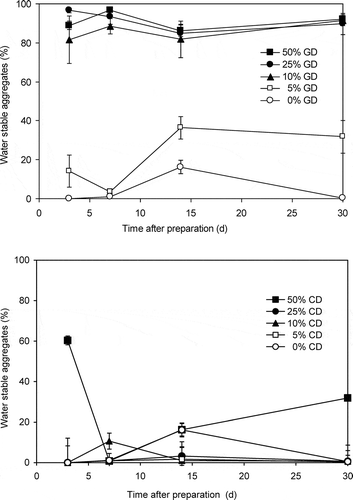
Samples with 10, 25, and 50% GD maintained more than 80% of WSA, without showing a considerable alteration with time. Samples with 5% GD showed low %WSA. As the %WSA of samples with 10, 25, and 50% GD-added soils were almost comparable, addition of more than 10% GD was considered to not be effective in further improving %WSA. Samples with 5% GD showed comparatively low %WSA.
Soil organic carbon content, which is reported to be directly correlated with wet aggregate stability, cannot be considered as the cause of high %WSA in GD-added samples because both CD and GD contain similar organic carbon contents (). Two types of manures show a major difference in carbon:nitrogen (C:N) ratio. According to Tennakoon and Bandara (Citation2003), the C:N ratios of GD and CD fall in a range of 5–9 and 12–20, respectively. Higher aggregate stabilization is reported to be related to low C:N ratio of residues (Peng et al. Citation2003; Bugg et al Citation2011). Aggregate stability in clay loam soils is reported to be high for manures with high decomposition intensity (Wakindiki and Yegon Citation2011), which can be related to low C:N ratio. Accordingly, high %WSA in GD-added soils might be considered to be a result of low C:N ratio. However, further experiment is needed to determine the exact clarifications, especially in field conditions.
3.1.2. Floating time of aggregates
Aggregates placed in water float as a result of entrapped air when water entry into air-dried aggregates is slow. Aggregate floating was observed when the aggregates were immersed in water during the measurement of %WSA. shows the aggregate floating times of CD- and GD-added soils. The floating time increased with increasing manure content. Aggregate floating times of CD-added soils were short compared with those of GD-added soils. Aggregates with 5% animal manure showed low floating times. The floating times of all the samples increased with time after the preparation of aggregates, which can be considered a result of gradual drying of aggregates.
Figure 2 Aggregate floating times of animal manure-added soils. CD, cow dung; GD, goat dung. Error bars indicate ± standard deviation.
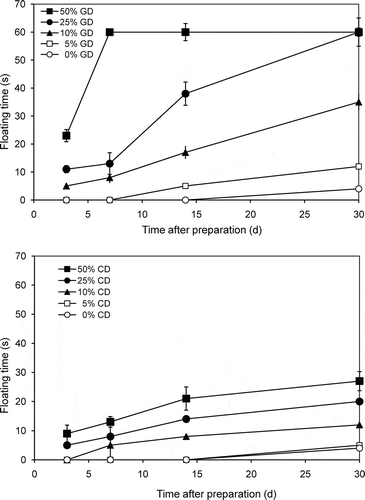
Results revealed that although the addition of about 10% GD improved the water stability of aggregates, it might have the risk of aggregate floating, which would consequently result in topsoil erosion.
3.1.3. Hydrophobicity of soils
The WDPTs of CD- and GD-added soils are presented in . Soils mixed with 25 and 50% animal manure were slightly water repellent (WDPT = 1–60 s) and those with 5 and 10% were non-repellent (WDPT ≤ 1 s).
Figure 3 Water drop penetration times (WDPT, s) of animal manure-added soils. CD, cow dung; GD, goat dung. Error bars indicate ± standard deviation.
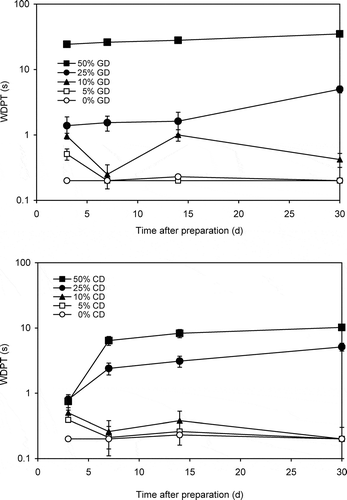
The WDPT increased with increasing manure content, and was high in GD-added soils, revealing that the WDPT and floating time of aggregates () show a positive correlation. Accordingly, it can be suggested that the floating time increases with increasing hydrophobicity.
Although the wettability was not considerably affected by the type of animal manure, aggregate stability was higher in GD-added soils (). The relations between WDPT and %WSA in GD-added soils are shown in . In GD-added soils, the %WSA was high (> 80%) when the WDPT was longer than 1 s. Such a relation could not be observed in CD-added soils.
3.2. Hydrophobic effect of green manure
The WDPT of 5, 10, 25, and 50% CE-added samples were initially (3 d after preparation) 50, 130, 200, and 2000 s, respectively. Samples with 50% CE, which were severely repellent, gradually altered with time to be strongly repellent after 30 d, whereas other samples did not show a considerable alteration. The GM-added soils showed instant wetting with WDPT < 1 s. The WDPT of 100% GM was about 1 s, whereas that of 100% CE was > 3600 s. Therefore, CE was considered hydrophobic, whereas GM was hydrophilic.
The %WSA of all the GM-added soils were approximately zero. In contrast, hydrophobic CE-added samples showed very high %WSA (). The %WSA of almost all the CE-added soils was about 90% and did not show a considerable alteration with time. However, aggregate floating occurred in all the samples. As shown in , floating times increased with increasing CE content. Samples with 5, 10, 25, and 50% CE respectively demonstrated 15, 75, 120, and > 120 s floating times 3 d after preparation, showing a positive relation with initial WDPT. These results further confirm that floating time increases with increasing hydrophobicity.
3.3. Effect of manure mixtures
The effect of manure mixtures on aggregate stability was tested 3 and 7 d after the preparation of aggregates. The possibility of using of 1–2% of hydrophobic CE with 5% organic manure as manure mixtures to improve the aggregate stability, while preventing or minimizing aggregate floating, was tested. The organic manure percentage in manure mixtures was fixed to 5% because 5% manure-added samples did not show the risk of aggregate floating and detrimental effects of water repellency. Although the aggregate stabilities of tested samples with 5% animal manure (GD and CD) were low, CE was expected to improve the %WSA.
shows the %WSA of soils with organic manure mixtures. Samples with only CE, and GD mixed with CE, showed > 50% of WSA 3 d after the preparation. Samples with 5% GD + 2% CE manure mixture showed the highest and the most stable %WSA. Additions of CP and PL were found not to be effective in improving aggregate stability with or without CE. Aggregate floating was not observed in any of the samples.
Figure 7 Percentage of water stable aggregates (% WSA) in soils with manure mixtures. GD, goat dung; PL, poultry litter; CP, compost; CE, Casuarina equisetifolia L. leaves. Error bars indicate ± standard deviation.
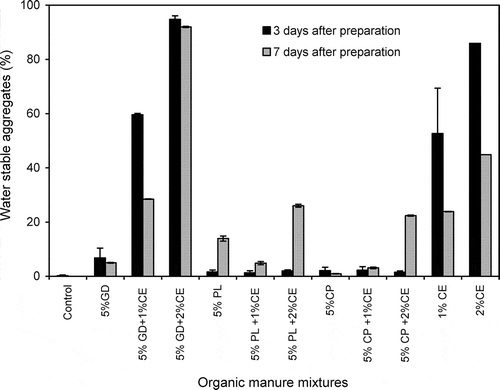
All the samples showed slight water repellency (1 < WDPT < 60 s). The WDPT of samples with 1% and 2% CE, which were 24 and 30 s respectively, decreased to below 10 s when mixed with other organic manures, except the samples with 5% GD + 2% CE. The WDPT of 5% CP and PL samples very slightly increased (1–2 s increment) when mixed with 1–2% CE, whereas that of 5% GD (WDPT=3 s) increased markedly when mixed with 2% CE (WDPT = 21 s). Strong, or higher water repellency (WDPT > 600 s) was not observed in any of the samples, showing that the addition of hydrophobic organic matter (CE) in suggested amounts (1–2%) would not induce detrimental effects of water repellency. Since aggregate floating did not occur in slightly repellent samples, it is clear that aggregates allow escaping air to move out.
There was no clear correlation between %WSA and the hydrophobicity of soils. However, results revealed several relations between hydrophobicity and %WSA; first, the %WSA was very high in samples with hydrophobic CE (); second, the %WSA increased when the 5% manure samples were mixed with 1–2% CE; third, samples with 5% GD + 2% CE, which had the highest WDPT among all the manure mixtures, showed the highest %WSA (). Accordingly, the %WSA was considered to have a tendency to increase with increasing hydrophobicity.
A commonly used manure application rate in Sri Lanka for poultry manure (chicken dung) is 10–15 t ha–1 (Wijewardena Citation1995), whereas that for cattle manure is 20–30 t ha–1 (Maraikar et al. Citation1996). Soils in Sri Lanka are low in organic matter, and no increase of organic matter in soil was observed by application of organic manure at a rate of 10 t ha–1 (Wijewardena Citation1995). Addition of the most succeeded amount of GD and CE, 5% GD + 2% CE, would mean the addition of about 50 and 20 t ha–1 (40 and 17 t ha–1 dry basis), respectively, in field conditions if the plow depth and the bulk density of topsoil are 10 cm and 1.0 Mg m–3, respectively. Although this could be regarded as a high rate compared with the usual rates of manure addition (20–30 t ha–1), it is necessary to acquire considerable aggregate stability as well as to increase the organic matter levels in soils. As a solution to this problem, the generation of organic manure in the field itself or in its near vicinity, which can easily be practiced in coconut and rubber plantations, can be recommended.
4. CONCLUSIONS
Goat dung improved the aggregate stability more strongly compared with CD. As the %WSA of 10, 25, and 50% GD-added soils were almost comparable, addition of more than 10% GD was considered not be effective in further improving aggregate stability. The %WSA did not show a considerable alteration with time. Addition of ≥ 10% GD would have a risk of aggregate floating, which would consequently result in topsoil erosion. The CD-added aggregates did not float; however, their stability against water was low.
Aggregates with 5–50% CE showed high and almost comparable stability of around 90% without showing an alteration with time, though aggregate floating would lead to extra soil loss. Addition of 2% of CE with 5% GD increased the %WSA. Addition of CP and PL were found not to be effective in improving aggregate stability with or without CE. Aggregate floating was not observed in any of the samples with manure mixtures. Strong or higher water repellency was not observed in any of the samples, showing that the addition of hydrophobic organic matter (CE) in suggested amounts (1–2%) would not induce detrimental effects of water repellency.
There was no clear correlation between %WSA and the hydrophobicity of soils. This is understandable because the water stability of aggregates is not solely a function of hydrophobic organic matter. However, the %WSA can be considered to show a tendency to increase with increasing hydrophobicity, owing to several findings. First, it was obvious that aggregates with high amounts of hydrophobic organic matter demonstrated high aggregate stabilities. Second, the %WSA increased when the 5% manure samples were mixed with 1–2% CE. Third, samples with the highest WDPT among all the manure mixtures showed the highest %WSA. Therefore, locally available hydrophobic organic matter might be effective in improving aggregate stability in Sri Lankan soils.
However, animal manure is becoming expensive due to high demand by vegetable and potato growers in the country, and the addition of high rates is required to obtain considerable aggregate stability. These considerations suggest that organic manure should be generated in the field itself or in its near vicinity if the practice is to be accepted by farmers. Increase of organic matter in soils is a difficult task due to prevailing high temperature in many parts of the country. In this regard, seasonal applications of organic materials would be necessary.
Further research is required on other effects, such as declining of infiltration rates, effects on seedling and plant growth, and effects of hydrophobic materials on soil and plants. Moreover, the possible accumulation of cadmium (Cd), zinc (Zn), and copper (Cu), which enter the soil as ions or chelates following decomposition of organic manure, and its subsequent effects on crops in the context of Sri Lanka need to be further investigated.
ACKNOWLEDGMENTS
This work is financially supported by the annual research fund of the Faculty of Agriculture, University of Ruhuna, Sri Lanka. Japan Society for the Promotion of Science is gratefully acknowledged for affording an Invitation Fellowship for Research in Japan (Long-term), which provided facilities for further analysis.
REFERENCES
- Bossuyt H, Denef K, Six J, Frey SD, Merckx R, Paustian K 2001: Influence of microbial populations and residue quality on aggregate stability. Appl. Soil Ecol., 16, 195–208.
- Bugg RL, Horwath W, Six J 2011: Agricultural soil ecology. In Cover Cropping for Vegetable Production: A Grower’s Handbook. Eds. R Smith, RL Bugg, M Gaskell, O Daugovish, MV Horn. p. 25. Agriculture & Natural Resources E-book, University of California, Davis, CA.
- Chaney K, Swift RS 1984: The influence of organic matter on the stability of some British soils. J. Soil Sci., 35, 223–230.
- Chenu C, Le Bissonnais Y, Arrouays D 2000: Organic matter influence on clay wettability and soil aggregate stability. Soil Sci. Soc. Am. J., 64, 1479–1486.
- Cosentino D, Hallett PD, Michel JC, Chenu C 2010: Do different methods for measuring the hydrophobicity of soil aggregates give the same trends in soil amended with residue? Geoderma, 159, 221–227.
- Díaz-Zorita M, Perfect E, Grove JH 2002: Disruptive methods for assessing soil structure. Soil Till. Res., 64, 3–22.
- Doerr SH, Thomas AD 2000: The role of soil moisture in controlling water repellency: New evidence from forest soils in Portugal. J. Hydrol., 231–232, 134–147.
- Eynard A, Schumacher TE, Lindstrom MJ, Malo DD, Kohl RA 2004: Wettability of soil aggregates from cultivated and uncultivated Ustolls and Usterts. Austr. J. Soil Res., 42: 163–170.
- Feng GL, Letey J, Wu L 2001: Water ponding depths affect temporal infiltration rates in a water repellent sand. Soil Sci. Soc. Am. J., 65, 315–320.
- Goebel MO, Bachmann J, Woche SK, Fischer WR 2005: Soil wettability, aggregate stability, and the decomposition of soil organic matter. Geoderma, 128, 80–93.
- King PM 1981: Comparison of methods for measuring severity of water repellence of sandy soils and assessment of some factors that affect its measurement. Aust. J. Soil Res., 19, 275–285.
- Le Bissonnais Y 1996: Aggregate stability and assessment of soil crustability and erodibility. I. Theory and methodology. Eur. J. Soil Sci., 47, 425–437.
- Leelamanie DAL, Karube J 2007. Effects of organic compounds, water content, and clay on water repellency of a model sandy soil. Soil Sci. Plant Nutr., 53, 711–719.
- Leelamanie DAL, Karube J, Yoshida A 2008: Characterizing water repellency indices: Contact angle and water drop penetration time of hydrophobized sand. Soil Sci. Plant Nutr., 54, 179–187.
- Maraikar S, Amarasiri SL, Wijewardena JDH 1996: Improving productivity of vegetable cultivation in Ultisols of Sri Lanka. In Proceedings of International Conference on Managing Soil Fertility for Intensive Vegetable Production Systems in Asia (AVRDC), Ed. Morris RA. pp. 270–282. Asian Vegetable Research and Development Centre, Tainan, Taiwan.
- Mataix-Solera J, Doerr SH 2004: Hydrophobicity and aggregate stability in calcareous top soils from fire-affected pine forests in southeastern Spain. Geoderma, 118, 77–88.
- National Atlas of Sri Lanka 2007: National Atlas of Sri Lanka, 2nd ed., Survey Department of Sri Lanka, Colombo, Sri Lanka.
- Nichols KA, Toro M 2011: A whole soil stability index (WSSI) for evaluating soil aggregation. Soil Till. Res., 111, 99–104.
- Özgül M, Aksakal Ekrem L, Günes A, Angn I, Turan M, Öztaş T 2011: Influence of global warming on aggregate stability and hydraulic conductivity under highland soil order in Turkey. Soil Sci., 176, 559–566.
- Peng XH, Zhang B, Zhao QG 2003: Effect of soil organic carbon on aggregate stability after vegetative restoration on severely eroded red soil. Acta Ecol. Sin., 23, 2176–2183 (In Chinese with English summary).
- Piccolo A, Mbagwu JSC 1999: Role of hydrophobic components of soil organic matter in soil aggregate stability. Soil Sci. Soc. Am. J., 63, 1801–1810.
- Pirmoradian N, Sepaskhah AR, Hajabbasi MA 2005. Application of fractal theory to quantify soil aggregate stability as influenced by tillage treatments. Biosyst. Eng., 90, 227–234.
- Poulenard J, Podwojewski P, Janeau JL, Collinet J 2001: Runoff and soil erosion under rainfall simulation of Andisols from the Ecuadorian Páramo: Effect of tillage and burning. Catena, 45, 185–207.
- Rillig MC, Mummey DL 2006: Mycorrhizas and soil structure. New Phytol., 171, 41–53.
- Six J, Bossuyt H, Degryze S, Denef K 2004: A history of research on the link between (micro) aggregates, soil biota, and soil organic matter dynamics. Soil Till. Res., 79, 7–31.
- Tennakoon NA, Bandara SDH 2003: Nutrient content of some locally available organic materials and their potential as alternative sources of nutrient for coconut. COCOS, 15, 1523–1530.
- Tillman RW, Scotter DR, Wallis MG, Clothier BE 1989: Water-repellency and its measurement by using intrinsic sorptivity. Austr. J. Soil Res., 27, 637–644.
- Wakindiki IIC and Yegon R 2011: Effect of decomposition intensity of incorporated chickpea manure on stability and saturated hydraulic conductivity of a clay loam and clay soil. Afr. J. Agric. Res., 6, 1120–1127.
- Wallis MG, Horne DJ 1992: Soil water repellency. Adv. Soil Sci., 20, 91–146.
- Wallis MG, Scotter DR, Horne DJ 1991: An evaluation of the intrinsic sorptivity water repellency index on a range of New Zealand soils. Aust. J. Soil Res., 29, 353–362.
- Wijewardena JDH 1995: Nutrient management under intensive cropping systems. The experience of the vegetable growing systems of the up-country of Sri Lanka. Proceedings of Workshop on Rural Credit for Crop Production in Sri Lanka. pp. 38–49. The Experience of the Block Demonstrations, Colombo, Sri Lanka.
- Zaher H, Caron J 2008: Aggregate slaking during rapid wetting: Hydrophobicity and pore occlusion. Can. J Soil Sci., 88, 85–97.