Abstract
The contribution of abiotic and biotic processes to the oxidation of arsenite [As(III)] when anaerobic paddy soils were shifted to oxic conditions was investigated. Soil slurries were first incubated under reducing conditions to allow indigenous arsenic (As) to be dissolved into the liquid phase as As(III), and were then switched to oxic incubation conditions with shaking. One day after switching to oxic incubation, As(III) almost disappeared from the liquid phase without any increase of arsenate [As(V)], suggesting that dissolved As(III) was co-precipitated or adsorbed on the soil solid phase. X-ray adsorption near-edge structure (XANES) analysis revealed that the predominant species of As in the solid phase before the oxic incubation was As(III), ranging from 74 to 85% of total As. After 1 d of the oxic incubation, the proportion of As(III) decreased to 46–47%. This oxidation step was an abiotic process and 28–38% of As(III) was oxidized per day on average. However, the abiotic oxidation ceased within 24 h probably due to passivation of reactive sites on the mineral surface. Afterward, a second slow oxidation step (2.5–2.8% per day on average) became predominant. Interestingly, this step was microbiologically mediated, since it did not occur in sterilized soil slurries. Determination of putative arsenite oxidase gene (aioA) sequences suggested that arsenite-oxidizing bacteria are actually present in our soil slurries. Our results suggest that microbial As(III) oxidation accounts for more than 30% of total As(III) oxidation, and thus it is an important process especially after abiotic oxidation ceases.
INTRODUCTION
The predominant forms of arsenic (As) in soil and water are inorganic arsenite [As(III)] and arsenate [As(V)]. Under oxic conditions, As(V) is predominant and it strongly binds to soil minerals including iron (Fe), manganese (Mn) and aluminum (hydr)oxides at near neutral pH. In contrast, under reducing conditions, arsenic occurs mostly as As(III), which is more mobile and toxic than As(V) (Smith et al. Citation1998). There is widespread concern about elevated concentrations of arsenic in the aquifer of Bangladesh and West Bengal (Nickson et al. Citation1998). In this region, groundwater is used not only for drinking but also for irrigation of paddy fields. The use of contaminated groundwater for irrigation water has resulted in an elevated concentration of arsenic in rice (Oryza sativa) grain (Rahman and Hasegawa Citation2011). The transfer of arsenic from soil to rice would amplify the risk of arsenic poisoning among people living in this region. It is thus important to understand arsenic mobilization and changes in arsenic speciation in paddy fields during the cycle of flooded and non-flooded periods. An increased concentration of As(III) in soil solution is sometimes observed in flooded paddy fields (Takahashi et al. Citation2004), suggesting that arsenic is dissolved from paddy soil. Although microbial activity can affect arsenic mobilization indirectly via reductive dissolution of iron (hydr)oxides associated with arsenic (Cummings et al. Citation1999), numerous studies have revealed that As(V) reduction might be a key process controlling arsenic mobilization (Yamamura et al. Citation2008; Ohtsuka et al. Citation2013). Recently, Yamaguchi et al. (Citation2011) found that microbial activity was indispensable for reduction of As(V) to As(III), when two Japanese paddy soils were incubated under reducing conditions.
Not only reductive dissolution of As(V), but adsorption and oxidation of As(III) in soils are also important processes to determine the toxicity, mobility and bioavailability of arsenic in terrestrial environments. Although As(III) specifically adsorbs to iron (hydr)oxides (Dixit and Hering Citation2003), it is generally accepted that ferric iron [Fe(III)] in soils does not oxidize As(III) significantly. Manganese oxide minerals (Mn oxides) are another important minerals, since they readily oxidize As(III) to As(V) (Oscarson et al. Citation1983). Although Mn-oxides are effective oxidants, the rate of As(III) oxidation decreases with time due to blocking of surface reactive sites by reaction products such as As(V), Mn(II) and possibly Mn(III) (Lafferty et al. Citation2010a, Citation2010b). On the other hand, a large number of As(III)-oxidizing bacteria have been isolated from soils (Phillips and Taylor Citation1976; Santini et al. Citation2000). They involve heterotrophic or autotrophic As(III) oxidizers, but it is not clear if such bacteria play significant role in As(III)-oxidizing processes in these environments. Yamamura et al. (Citation2009) incubated five types of soil slurry with As(III), and found that As(III) was oxidized to As(V) microbiologically under aerobic conditions. In addition, Jones et al. (Citation2012) recently reported that As(III) oxidation by synthetic Mn oxides was enhanced in the presence of As(III)-oxidizing bacteria such as Pseudomonas fluorescens and Agrobacterium tumefaciens. However, in these studies, arsenic concentrations used (5.6–75 mg L−1 in the liquid phase) were significantly higher than those observed in natural environments.
As described above, As(III) oxidation in soils may proceed both abiotically and microbiologically. However, neither their contributions to As(III)-oxidizing process nor the proportion of As(III) oxidized per day on average by each process has been determined separately in natural soils. The aim of this study was to quantify each As(III)-oxidizing reaction in paddy soils under ambient levels of arsenic.
MATERIALS AND METHODS
Soil samples
Soil samples were collected from the surface layer of a fallow paddy field (soil A) and from the plow layer of a paddy field (soil B) in April 2008. Both soils were classified as Aeric Epiaquents in US taxonomy (Soil Survey Staff Citation1994). Arsenic concentrations of soils A and B were 37.1 and 39.5 mg kg−1, respectively. Detailed soil properties of soils A and B were reported by Yamaguchi et al. (Citation2011). The soils were passed through a 2-mm sieve under field moist conditions and stored in a refrigerator at 7°C until use.
Incubation of soil slurry
For anaerobic incubation, moist soil (20 g after oven-drying at 105°C for 12 h) was mixed with 60 mL of deionized water in a 100-mL glass vial. After purging with Nitrogen (N) gas for 3 min, the vial was sealed with a butyl rubber stopper and an aluminum cap. The soil slurry was incubated statically at 30°C. The incubation periods were 60 d for soil A and 100 d for soil B. Soil B required a longer incubation period than soil A to reach a sufficiently low oxidation-reduction potential (Eh), probably due to its lower carbon content (Yamaguchi et al. Citation2011). Afterward, the incubation condition was then switched to oxic. The anaerobically-incubated slurry was transferred from the glass vial to a 300-mL Erlenmeyer flask with a silicone cap, and was incubated at 30°C with rotary shaking at 200 rpm for 1 week. At various time intervals, the soil slurry was removed to a centrifuge tube and then centrifuged at 5000 × g for 15 min. The supernatant was filtered using a centrifuge filter unit (Vivaspin 20, pore size 0.22 μm, Sartorius Stedim, Göttingen, Germany). To prevent precipitation of Fe (hydr)oxide, 1 mL of 10% nitric acid (HNO3) was added to 9 mL of the filtrate, and then was applied to analyses of arsenic speciation and the total concentration of arsenic in aqueous phase by a high performance liquid chromatography connected with a quadrupole inductively coupled plasma mass spectrometer (HPLC-ICPMS, see below). To investigate the effects of microbial activity on the As(III) oxidation, half of the soil slurries were irradiated with γ-ray at 30 kGy after the anaerobic incubation.
Analyses of solution phase and solid phase
HPLC (PU 712i, GL Science, Tokyo, Japan) together with ICPMS (ELAN DRC-e, PerkinElmer) was used to measure the speciation of arsenic in the liquid phase (Yamaguchi et al. Citation2011). Inertsil ODS-3 column (4.6 × 150 mm; GL Science) was used for the separation of As(III) and As(V). The eluent was 5% (volume/volume) methanol, 5 mM tetrabutylammonium hydroxide and 3 mM malonic acid at pH 5.6, and flow rate was 1 mL min−1. The retention times for As(III) and As(V) were 2 and 6 min, respectively. Total arsenic concentration was determined by ICPMS without passing the solution through the column. Fe concentration was determined by inductively coupled plasma optical emission spectrometer (ICP-OES, VistaPro, Varian, Palo Alto, CA, USA).
To determine the speciation of arsenic in the solid phase, arsenic K-edge (11,867 eV) XANES spectra were acquired at BL 12C of photon factory (PF, High-Energy Accelerator Research Organization, KEK, Tsukuba, Japan). The XANES spectra of soil samples were collected in the fluorescence detection mode using a 19-element Ge semiconductor detector, whereas those of the reference materials were collected in the transmission mode. Energy calibration was made by the white line peak of sodium arsenite (NaAsO2) assigned at 11,865 eV for As. The soil samples were analyzed as a wet paste at an ambient temperature. Compositions of As species in the soil solid phases were evaluated by linear combination fitting (LCF) of XANES spectra with model compounds, as proposed by Takahashi et al. (Citation2003). The model compounds for LCF included NaAsO2 [As(III)] and sodium arsenate (Na2HAsO4 [As(V)]) solids. The fitting range was 11,855–11,880 eV. The REX 2000 ver. 2.5 program packages (Rigaku, Tokyo, Japan) were used to subtract the pre-edge background and normalize the spectra and LCFs.
Determination of As(III) oxidase genes (aioA) in soil slurries
DNA was extracted from paddy soil slurries after the oxic incubation for 7 d using a FastDNA Spin kit (MP Biomedicals, Morgan Irvine, CA, USA). The aioA gene was amplified by polymerase chain reaction (PCR) protocols as described by Hamamura et al. (Citation2009) using degenerate primers aroA95f (5′-TGYCABTWCTGCAIYGYIGG-3′) and aroA599r (5′-TCDGARTTGTASGCIGGICKRTT-3′). The PCR products were cloned into pMD20-T vectors using a Mighty TA-Cloning kit (Takara Bio, Otsu, Japan) and transformed into DH5α chemically competent Escherichia coli (Takara Bio) according to the manufacturer’s instructions. Fifteen clones were randomly chosen for sequencing from soils A and B. These clones were sequenced using BigDye Terminator v3.1 Cycle Sequencing kit (Applied Biosystems, Foster City, CA, USA) and an ABI Prism 3100 Genetic Analyzer (Applied Biosystems). The obtained gene sequences were translated to amino acid sequences, and were subjected to Basic Local Alignment Search Tool (BLAST) search (http://www.ncbi.nlm.nih.gov/BLAST/) to determine sequence identity. The retrieved sequences were aligned using the Clustal X program, version 2.0. The phylogenetic tree was constructed using the neighbor-joining method (Saito and Nei Citation1987). Bootstrap values were obtained for 1000 replicates to estimate the confidence of tree topologies. The aioA gene sequences identified in this study have been deposited in the DDBJ/EMBL/GenBank databases under accession numbers AB897741 to AB897755, and AB905499 to AB905513.
RESULTS AND DISCUSSION
Liquid phase analysis
Slurries prepared from soils A and B were first incubated anaerobically under reducing condition. In slurries of soils A and B, 3.61 and 2.92 μmol L−1 of indigenous arsenic dissolved into the liquid phase mainly as As(III). The slurries were then incubated under oxic conditions with shaking. shows changes in the concentration of arsenic in the liquid phase of the slurries. After oxic incubation for 1 d, more than 92% of dissolved As(III) disappeared from the liquid phase of the slurries ( and ). When the slurries were irradiated with γ-ray at 30 kGy before the oxic incubation, changes in arsenic concentration on the first day were similar to those observed in the unsterilized slurries ( and ). This result suggests that an abiotic process was responsible for the As(III) removal from the liquid phase.
Figure 1 Concentration of arsenite [As(III)] and arsenate [As(V)] in the liquid phase of soil slurries incubated under oxic condition. Data from soil slurries prepared from (a) unsterilized soil A, (b) γ-ray irradiated soil A, (c) unsterilized soil B and (d) γ-ray irradiated soil B are shown. Symbols represent the mean values obtained for duplicate determinations, and bars indicate range of values. The absence of bars indicates that the error is smaller than the symbol. As, arsenic.
![Figure 1 Concentration of arsenite [As(III)] and arsenate [As(V)] in the liquid phase of soil slurries incubated under oxic condition. Data from soil slurries prepared from (a) unsterilized soil A, (b) γ-ray irradiated soil A, (c) unsterilized soil B and (d) γ-ray irradiated soil B are shown. Symbols represent the mean values obtained for duplicate determinations, and bars indicate range of values. The absence of bars indicates that the error is smaller than the symbol. As, arsenic.](/cms/asset/f68b9aee-39bb-42fe-95d5-87c7ee11d6ae/tssp_a_897924_f0001_b.gif)
In our soil slurries, 1.61–1.79 mmol L−1 of iron dissolved into the liquid phase after the anaerobic incubation, which was determined by ICP-OES (data not shown). The co-precipitation of As(III) with dissolved ferrous iron [Fe(II)] has been considered an effective mechanism to immobilize As(III) from the liquid phase (Roberts et al. Citation2004; Ona-Nguema et al. Citation2009). Alternatively, dissolved Fe(II) may be oxidized during aeration and form poorly crystalline iron (hydr)oxides, which are known as superior As(III) adsorbents at neutral pH (Hering et al. Citation1997; Dixit and Hering Citation2003). Thus, it is also possible that dissolved As(III) was adsorbed on the surface of iron (hydr)oxides during the oxic incubation. At present, however, it is uncertain which process occurred predominantly in our experiment. It seems unlikely that As(III) was first oxidized in the liquid phase and As(V) was then adsorbed on the soil solid phase, since As(III) oxidation by oxygen proceeds very slowly in solution (Tallman and Shaikh Citation1980).
Solid phase analysis
Distribution of As(III) and As(V) in the solid phase was determined by XANES (Fig. S1). Changes in energy of the white line peak centroid were observed, suggesting that As(III) was oxidized to As(V) in the soil solid phase. The proportion of As(III) in the solid phase was estimated from LCF (). After the anaerobic incubation, 74–85% of total arsenic was As(III) in unsterilized soil slurries. However, it decreased to 46–47% after oxic incubation for 1 d, indicating that 28–38% of As(III) was oxidized per day. Since similar rates of As(III) oxidation were observed in γ-ray irradiated soil slurries, this process was considered to be an abiotic one. Mn oxides are common soil minerals with a high capacity to oxidize heavy metals and metalloids including As(III) (Post Citation1999). The effective oxidation of As(III) by synthetic Mn oxides such as birnessite (δ-MnO2) has been reported (Oscarson et al. Citation1981, Citation1983). However, Lafferty et al. (Citation2010a, Citation2010b) reported that reaction products of As(III) oxidation by Mn oxides, i.e., As(V), Mn(II) and possibly Mn(III), immediately block reactive sites on the mineral surface. In our study, the abiotic oxidation was found not to proceed further, since As(III) oxidation did not occur in the sterilized slurries after 1 day (). Thus, it is possible that Mn oxides played a role in the abiotic oxidation of As(III) in our soil slurries.
Figure 2 Proportion of arsenite [As(III)] in the solid phase of (a) soil A and (b) soil B incubated under oxic conditions. Results from unsterilized and γ-ray irradiated soil slurries are shown.
![Figure 2 Proportion of arsenite [As(III)] in the solid phase of (a) soil A and (b) soil B incubated under oxic conditions. Results from unsterilized and γ-ray irradiated soil slurries are shown.](/cms/asset/19958f3b-ff08-4082-bb80-03c5312904c0/tssp_a_897924_f0002_b.gif)
In unsterilized soil slurries, As(III) was further oxidized linearly after 1 d, although the proportion of As(III) oxidized per day on average was more than 10 times lower (2.5–2.8% per day on average) than that observed on the first day. However, this second oxidation step did not occur in the γ-ray irradiated soils (), indicating that it was catalyzed microbiologically. We also analyzed As(III) oxidase genes (aioA) in our soil slurries, which had been incubated aerobically for 7 d. As shown in , a total of 30 clones were determined, and their amino acid sequences showed close relatedness with putative AioA of Alpha-, Beta- and Gammaproteobacteria. These results suggest that As(III)-oxidizing bacteria are actually present in our soil slurries.
Figure 3 Neighbor-joining phylogenetic tree of putative arsenite oxidase (AioA) amino acid sequences found in two types of soil slurries (soil A and soil B). Circles and triangles at the branch nodes represent bootstrap percentages (1000 replicates): filled circles, 90–100%; open circles, 70–89%; open triangles, 50–69%. Values < 50% are not shown. The scale bar represents the estimated number of substitutions per site.
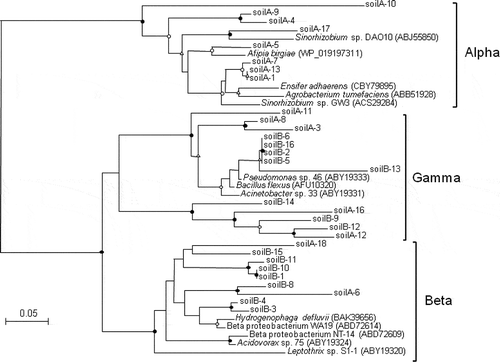
It should be noted that microbial oxidation accounts for more than 30% of total As(III) oxidation, and thus it is an important process especially once the abiotic oxidation ceases after 1 d. As shown in and , approximately 0.27 μmol L−1 of As(III) remained in the liquid phase in sterilized soil slurries. Thus, it may be possible that soil bacteria do not oxidize As(III) directly in the soil solid phase, but oxidize dissolved As(III) partitioned into the liquid phase. Recently, Jones et al. (Citation2012) determined the time dependency of As(III) oxidation by birnessite and As(III)-oxidizing soil bacteria in batch experiments. They found that the apparent rate of dissolved As(V) formation was highest when bacteria and birnessite were mixed, while much slower rates were observed when the experiments were performed by either component alone. Our results showed a good agreement with the study by Jones et al. (Citation2012), since unsterilized soils including both soil bacteria and minerals showed much higher oxidation rates than sterilized soils including only soil minerals.
In conclusion, we demonstrated that a rapid abiotic oxidation was accompanied by a much slower bacterial oxidation in Japanese paddy soils. Interestingly, bacterial As(III) oxidation is an important process especially after abiotic As(III) oxidation ceases. Bacteria might oxidize the residual dissolved As(III) in the liquid phase. Therefore, bacterial contribution to As(III) oxidation becomes more significant if dissolved As(III) level in soils is much higher than those observed in this study (3–4 μmol L−1). Further study is needed to better understand the role of soil bacteria in soils of high arsenic level.
Figure S1 Arsenic K-edge XANES spectra of reference materials and soil slurries prepared from unsterilized soil B (a), and ?-ray irradiated soil B (b).
Download MS Word (135.7 KB)ACKNOWLEDGMENTS
We are grateful to Prof. Y. Takahashi (Hiroshima University) for XANES measurements. This work was supported by grants from the Ministry of Agriculture, Forestry and Fisheries of Japan (Research Project for Ensuring Food Safety from Farm to Table AC-1122) and from JSPS KAKENHI (Grant No. 23580103). The XANES measurements were performed under the approval of the High-Energy Accelerator Research Organization, KEK (Proposal No. 2011G016 and 2012G578).
References
- Cummings DE, Caccavo F, Fendorf S, Rosenzweig RF 1999: Arsenic mobilization by the dissimilatory Fe(III)-reducing bacterium Shewanella alga BrY. Environ. Sci. Technol., 33, 723–729. doi:10.1021/es980541c
- Dixit S, Hering JG 2003: Comparison of arsenic(V) and arsenic(III) sorption onto iron oxide minerals: Implications for arsenic mobility. Environ. Sci. Technol., 37, 4182–4189. doi:10.1021/es030309t
- Hamamura N, Macur RE, Korf S, Ackerman G, Taylor WP, Kozubal M, Reysenbach AL, Inskeep WP 2009: Linking microbial oxidation of arsenic with detection and phylogenetic analysis of arsenite oxidase genes in diverse geothermal environments. Environ. Microbiol., 11, 421–431. doi:10.1111/j.1462-2920.2008.01781.x
- Hering JG, Chen P, Wilkie JA, Elimelech M 1997: Arsenic removal from drinking water during coagulation. J. Environ. Eng., 123, 800–807. doi:10.1061/(ASCE)0733-9372(1997)123:8(800)
- Jones LC, Lafferty BJ, Sparks DL 2012: Additive and competitive effects of bacteria and Mn oxides on arsenite oxidation kinetics. Environ. Sci. Technol., 46, 6548–6555. doi:10.1021/es204252f
- Lafferty BJ, Ginder-Vogel M, Sparks D 2010a: Arsenite oxidation by a poorly crystalline manganese-oxide 1. Stirred-flow experiments. Environ. Sci. Technol., 44, 8460–8466. doi:10.1021/es102013p
- Lafferty BJ, Ginder-Vogel M, Zhu M, Livi JT, Sparks D 2010b: Arsenite oxidation by a poorly crystalline manganese-oxide. 2. Results from X-ray absorption spectroscopy and X-ray diffraction. Environ. Sci. Technol., 44, 8467–8472. doi:10.1021/es102016c
- Nickson RT, McArthur JM, Burgess WG, Ahmed KM, Ravenscroft P, Rahman M 1998: Arsenic poisoning of Bangladesh groundwater. Nature, 395, 338. doi:10.1038/26387
- Ohtsuka T, Yamaguchi N, Makino T, Sakurai K, Kimura K, Kudo K, Homma E, Dong DT, Amachi S 2013: Arsenic dissolution from Japanese paddy soil by a dissimilatory arsenate–reducing bacterium Geobacter sp. OR–1. Environ. Sci. Technol., 47, 6263–6271.
- Ona-Nguema G, Morin G, Wang Y, Menguy N, Juillot F, Olivi L, Aquilanti G, Abdelmoula M, Ruby C, Bargar JR, Guyot F, Calas G, Brown GE 2009: Arsenite sequestration at the surface of nano-Fe(OH)2, ferrous-carbonate hydroxide, and green-rust after bioreduction of arsenic-sorbed lepidocrocite by Shewanella putrefaciens. Geochim. Cosmochim. Acta, 73, 1359–1381. doi:10.1016/j.gca.2008.12.005
- Oscarson DW, Huang PM, Liaw WK 1981: Role of manganese in the oxidation of arsenite by freshwater lake sediments. Clays Clay Miner., 29, 219–225. doi:10.1346/CCMN.1981.0290308
- Oscarson DW, Huang PM, Liaw WK, Hammer UT 1983: Kinetics of oxidation of arsenite by various manganese oxides. Soil Sci. Soc. Am. J., 47, 644–648. doi:10.2136/sssaj1983.03615995004700040007x
- Phillips SE, Taylor ML 1976: Oxidation of arsenite to arsenate by Alcaligenes faecalis. Appl. Environ. Microbiol., 32, 392–399.
- Post JE 1999: Manganese oxide minerals: Crystal structures and economic and environmental significance. Proc. Natl. Acad. Sci. USA, 96, 3447–3454. doi:10.1073/pnas.96.7.3447
- Rahman MA, Hasegawa H 2011: High levels of inorganic arsenic in rice in areas where arsenic-contaminated water is used for irrigation and cooking. Sci. Total Environ., 409, 4645–4655. doi:10.1016/j.scitotenv.2011.07.068
- Roberts LC, Hug SJ, Ruettimann T, Billah MM, Khan AW, Rahman MT 2004: Arsenic removal with iron(II) and iron(III) in waters with high silicate and phosphate concentrations. Environ. Sci. Technol., 38, 307–315. doi:10.1021/es0343205
- Saito N, Nei M 1987: The neighbor-joining method: A new method for reconstructing phylogenetic trees. Mol. Biol. Evol., 4, 406–425.
- Santini JM, Sly LI, Schnagl RD, Macy JM 2000: A new chemolithoautotrophic arsenite-oxidizing bacterium isolated from a gold mine: Phylogenetic, physiological, and preliminary biochemical studies. Appl. Environ. Microbiol., 66, 92–97. doi:10.1128/AEM.66.1.92-97.2000
- Smith E, Naidu R, Alston AM 1998: Arsenic in the soil environment: A review. Adv. Agron., 64, 149–195. doi:10.1016/S0065-2113(08)60504-0
- Soil Survey Staff (Ed) 1994: Keys to Soil Taxonomy. Pocahontas Press, Blacksburg, VA, USA.
- Takahashi Y, Minamikawa R, Hattori KH, Kurishima K, Kihou N, Yuita K 2004: Arsenic behavior in paddy fields during the cycle of flooded and non-flooded periods. Environ. Sci. Technol., 38, 1038–1044. doi:10.1021/es034383n
- Takahashi Y, Ohtaku N, Mitsunobu S, Yuita K, Nomura M 2003: Determination of the As(III)/As(V) ratio in soil by X-ray absorption near-edge structure (XANES) and its application to the arsenic distribution between soil and water. Anal. Sci., 19, 891–896. doi:10.2116/analsci.19.891
- Tallman DE, Shaikh AU 1980: Redox stability of inorganic arsenic(III) and arsenic(V) in aqueous solution. Anal. Chem., 52, 196–199. doi:10.1021/ac50051a047
- Yamaguchi N, Nakamura T, Dong D, Takahashi Y, Amachi S, Makino T 2011: Arsenic release from flooded paddy soils is influenced by speciation, Eh, pH, and iron dissolution. Chemosphere, 83, 925–932. doi:10.1016/j.chemosphere.2011.02.044
- Yamamura S, Watanabe M, Kanzaki M, Soda S, Ike M 2008: Removal of arsenic from contaminated soils by microbial reduction of arsenate and quinone. Environ. Sci. Technol., 42, 6154–6159. doi:10.1021/es703146f
- Yamamura S, Watanabe M, Yamamoto N, Sei K, Ike M 2009: Potential for microbially mediated redox transformations and mobilization of arsenic in uncontaminated soils. Chemosphere, 77, 169–174. doi:10.1016/j.chemosphere.2009.07.071