ABSTRACT
In nature, iron (Fe) occurs in abundance and ranks fourth among all elements on Earth’s surface. Still, its availability to plants is reduced, once this element is in the form of hydrated oxides, which can limit plant productivity and biomass production. On the other hand, in high concentrations, this essential micronutrient for the plants can become a toxic agent, increasing the environmental contamination. Fe is necessary for the maintenance of essential processes like respiration and photosynthesis, participating in the electron transport chain and in the conversion between Fe2+ and Fe3+, being a key element for carbon dioxide (CO2) fixation and, therefore, important for crop production of cultivated or natural species. The balance of Fe should be strictly controlled, because both its deficiency and its toxicity affect the physiological process of plants. In aerated soils Fe is present in the form of Fe3+, which is the oxidized form and is less available to plants, so these organisms have developed different strategies for absorption, transport and storage of Fe. Deficiency and excess of Fe correlate with local soil conditions and with the care adopted in plant nutrition during the phenological phases and/or in the course of its cultivation. In situations of excessive accumulation of Fe in tissues, an enhancement of hydroxyl radical generation (OH•) occurs by Fenton reaction. Here, we review the nutritional, genetic and ecophysiological aspects of uptake, translocation and accumulation of Fe ions in plants growing under conditions of deficiency or toxicity of this metal.
1. Introduction
Iron (Fe) is an essential nutrient for plants, required for vital processes like respiration and photosynthesis, and playing a fundamental role in electron transfer and reversible redox reactions (Kim and Guerinot Citation2007; Balk and Schaedler Citation2014).
Although Fe is a constituent of approximately 5% of Earth’s crust (Mengel and Kirkby Citation1987), most of it is not readily available to plants, causing symptoms of Fe deficiency like internerval chlorosis and reduced plant productivity (Jeong and Guerinot Citation2009). In addition, its absorption depends on the species, genotype, environment, time of year and plant age (Briat et al. Citation2010). In anthropic ecosystems, the excess of Fe can alter plant metabolism and nutrient cycling, resulting in a significant reduction in growth, flowering and fruiting (Grantz et al. Citation2003; Grotz and Guerinot Citation2006). Understanding the Fe deficiency response in plants is necessary for improving both plant health and the human diet, which relies on Fe from plant sources (Welch and Graham Citation2004; Hindt and Guerinot Citation2012).
In the soil, Fe is found in the form of Fe3+ (ferric iron) and Fe2+ (ferrous iron) and is highly dependent on the redox state of the system. Chemically, organisms have developed specific mechanisms for Fe acquisition from the Fe-oxides (III): (i) protonation, (ii) chelation and (iii) reduction (Guerinot and Yi Citation1994). Non-exchangeable Fe is present in several primary minerals like biotite, hornblende, augite and olivine. The primary Fe oxides present in several soils contain hematite, magnetite and ilmenite, and in sedimentary rocks, there are primary forms like oxides and siderite. In the secondary minerals, Fe is present in several clay minerals and is also connected to organic complexes (Hansel et al. Citation2001).
According to the classification of plant mineral nutrients with biochemical function, Fe is in Group 4, which makes it part of the nutrients that are involved in redox reactions, with the constituent function of cytochromes and nonheme iron proteins involved in photosynthesis, nitrogen gas (N2) fixation and respiration (Ravet et al. Citation2009).
The balance of Fe should be strictly controlled, because both its deficiency and its toxicity affect the physiological processes of plants (Walker and Connolly Citation2008) with implications for their development and growth (Kuki et al. Citation2008; Briat et al. Citation2010). Fe is an essential chemical element for all living cells, because it is related to the constitutions of important macromolecules like those involved in respiration, photosynthesis and metabolism. However, an excess of free Fe is harmful to living cells due to the possibility of reaction with oxygen, producing harmful free radicals (Ravet et al. Citation2009). In cultivated soils, pH greatly influences the solubility of Fe compounds, where one pH unit can provide a 99% decrease in the availability of Fe for plants. To supply the need for Fe, roots use different mechanisms/strategies such as the formation of soluble chelate complexes (Zhao et al. Citation2011; Kobayashi et al. Citation2014).
2. Physiological basis for iron uptake by plants
Fe has great importance in iron-sulfur proteins and also as a catalyst in redox reactions, as occur in nitrogen (N) metabolism. Plants absorb Fe from the soil that is in the form of primary minerals like ferric iron (Fe3+) and the oxides: Fe(OH)2+, Fe(OH)3 and Fe(OH)4–. At pH = 7, ferric Fe is practically insoluble, so for plants to absorb Fe, the roots have developed mechanisms to increase its solubility and consequently its availability (Guerinot and Yi Citation1994).
The availability of Fe sources is affected by pH. In acidic pHs the soluble forms of Fe predominate and in alkaline pHs the insoluble forms predominate (). Fe can be absorbed as Fe2+, Fe3+ and as Fe-chelate, and its absorption is metabolically controlled by plants. At least two strategies or processes are involved (Kobayashi et al. Citation2014). In iron deficiency, strategy I is present in eudicotyledons and in non-monocot grasses. In this strategy, protons are pumped to the extraradicular environment (rhizosphere) by activation of the plasma membrane proton-adenosinetriphosphatases (P-ATPases), which promotes the acidification of the soil solution (Ramos et al. Citation2009b) and increased solubility of Fe3+ (, ). P-ATPases are a primary proton transport system found in bacteria, archaea and eukaryotes (Palmgren Citation2001; Yan et al. Citation2002; Colangelo and Guerinot Citation2006; Revathi and Venugopal Citation2013). These enzymes are considered eletrogenic pumps, because they transport positive charge in the form of H+, generating a difference in charges on either side of the membrane, called membrane potential (MP), and simultaneously generate a difference in the chemical potential of H+ or proton concentration gradient (ΔpH) (Yan et al. Citation2002; Ramos et al. Citation2009a). The electrochemical difference produced by this enzyme is used as a driving force in fundamental cellular processes, such as activation of secondary transporters, cellular signaling, nutrient uptake and proton extrusion (Palmgren Citation2001). The change of one unit of rhizosphere pH promoted by activation of P-ATPase can alter the solubility of Fe3+. It has been estimated that at pH 3.0, Fe is 1000 times more soluble than at pH 6.0. In these conditions dependents of P-ATPase, the ferric reductase enzyme, encoded by the Ferric Chelate Reductase (FRO) gene, reduces Fe3+ to Fe2+ in the surface of the plasma membrane of root cells. FRO proteins are localized in the epidermal cells and the FRO gene is expressed under Fe deficiency conditions. After reduction, Fe is then transported to the cytoplasm through an assembly of metal transporters from the Zinc-Iron Permease (ZIP) family like Iron Regulated Transporters (IRTs) (). Both genes are regulated by iron deficiency and activated by specific basic helix-loop-helix (bHLH) and transcription factors, which are also regulated by iron deficiency (Walker and Connolly Citation2008; Hindt and Guerinot Citation2012).
Figure 1. Speciation of different iron forms (A: Fe-citrate; B: FeSO4; C: FeCl3 and D: Fe-EDTA) depending on pH calculated by Visual Minteq Program v. 3.0 (Ramos et al. Citation2009a). This modelling take into account the dissociation, complexation and precipitation reactions occurring in solutions and the dynamic equilibrium. All these reactions take place at the same time and interact the iron form with medium pH demonstrating the conditions which affect the iron bioavailability.
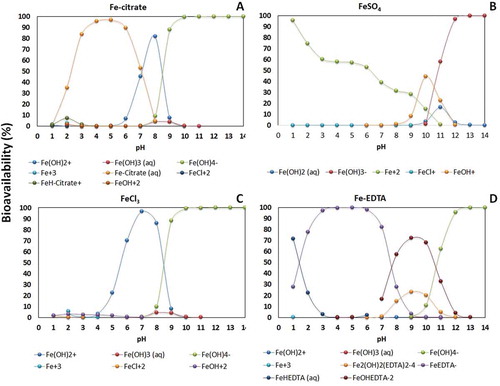
Figure 2. Strategies for iron (Fe) uptake in the soil by plants. A. Fe uptake in non-grasses plants (Strategy I). Acidification by plasma membrane H+-ATPase (P-ATPase) increases the solubility of Fe3+ (Fe III) in the rhizosphere and FRO2 reduces Fe3+ to Fe2+ increasing Fe2+ (Fe II) uptake by Iron Regulated Transporter - IRT1 transporter on the root epidermis. B. Fe uptake in grasses plants (Strategy II). A Phytosiderophore (PS) transporter of the major facilitator superfamily (MFS) was recently identified in barley (Hordeum vulgare L.) denominated HvTOM1 responsible for the efflux of the PS 2′-desoximugineic acid (DMA). C. Fe uptake in rice (Oryza sativa L.) plants (Strategy I/II). TOM1 transporter mediates the PS (DMA) secretion through the plasma membrane. The Fe (III)-DMA complex formed in the rhizosphere is then taken up into root cells via the OsYSL15 transporter. In addition to Fe (III)-DMA uptake, rice also possesses the components of Fe2+ uptake system.
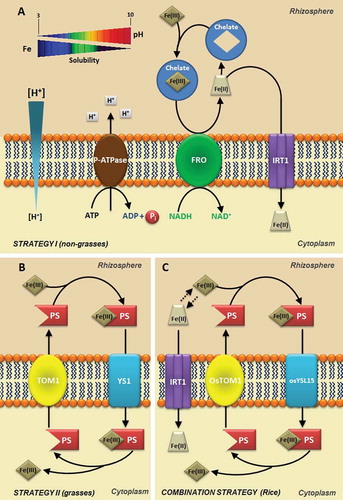
For several plants, the ability of roots to reduce Fe3+ to Fe2+ is essential for the absorption of this cation, since the reduction is required before its absorption (Chaney et al. Citation1998; Conte and Walker Citation2011). Two isoforms of P-ATPases (CsHA1 and CsHA2) were isolated from cucumber (Cucumis sativus L.) and their expressions were analyzed in terms of nutritional status of Fe (Santi et al. Citation2005). The authors showed that in roots with Fe deficiency, there was a greater expression of the CsHA1 gene, which was not detectable in the leaves, and concluded that the Fe supply to the deficient plants caused a decrease in the transcription level of CsHA1. In contrast, CsHA2 expression was down-regulated in leaves and roots by the availability of Fe (Santi et al. Citation2005).
Under certain environmental conditions, plant roots can enhance the release of exudates that increase the mobilization of essential mineral elements in the rhizosphere or inhibit the absorption of toxic metals in soil solution (Marschner et al. Citation2011). Additionally to the P-ATPases, plants roots can use, for example, citrate and other dicarboxylates during phosphorus (P) and Fe deficiency, so these elements can be mobilized from calcium (Ca), aluminum or iron phosphate, which are originally less soluble (Neumann and Römheld Citation1999).
Strategy II, characteristic of the family Poaceae, or grasses, occurs in Fe deficiency, where the roots release compounds called phytosiderophores (). These phytosiderophores are composed of amino acids not found in proteins, representatives of the family of mugineic acids (MA) that includes 2´-desoximugineic acid (DMA), 3–3-epihidroximugineic acid (epiHMA), and 3-epihydroxy-2ʹ-deoxymugineic acid (epiHDMA), which forms stable compounds with Fe3+ in soil (). In pathogenic interactions, siderophores are involved in iron acquisition from the host and are sometimes necessary for the expression of full virulence; however, in plants they can trigger immunity (Aznar and Dellagi Citation2015).
Studies have shown that tolerance to Fe deficiency is correlated with the amounts and types of phytosiderophores segregated, which is highly dependent on the plant species (Marschner et al. Citation2011). Ueno et al. (Citation2007) identified and characterized the phytosiderophores in root exudates from perennial grasses and found that Lolium perenne cv. Tove secreted both epiHDMA and DMA while Poa pratensis cv. Baron secreted DMA and avenic acid A (AVA). They also purified and identified two new types of phytosiderophores: 3-hydroxy-2ʹ-deoxymugineic acid (HDMA) from L. perenne, and 2ʹ-hydroxyavenic acid A (HAVA) from P. pratensis. AVA was previously reported as an amino acid derivative with an iron chelating activity from Avena sativa roots growing under iron-deficient conditions (Fushiya et al. Citation1980). For example, rice, wheat and maize (Oryza sativa L., Triticum aestivum L., Zea mays L.) secrete DMA only in relatively low amounts and, therefore, are sensitive to low Fe availability. In contrast, barley (Hordeum vulgare L.) secretes large quantities of several types of phytosiderophores including MA, HMA, and epi-HMA and it is, therefore, more tolerant to low Fe availability (Negishi et al. Citation2002; Bashir et al. Citation2006).
The phytosiderophores have high affinity for Fe3+, binding efficiently to this element in the rhizosphere. The siderophores are released and form complexes with Fe3+, which are specifically transported to the interior of root cells, without the reduction to Fe2+ in this second process (Curie and Briat Citation2003; Hindt and Guerinot Citation2012). This chelation strategy is more efficient than the reduction strategy and, thus, allows grasses to survive in more severe conditions of Fe deficiency.
The absorption of Fe3+ chelate by phytosiderophores is performed by the Yellow Stripe 1 (YS1) transport systems located at the plant root cells. It has been substantiated that the YS1 protein contains multiple putative transmembrane domains, which is consistent with this being a membrane protein that mediates ion uptake. The YS1 mRNA levels were increased in roots under iron-deficient conditions, and the expression of YS1 in an iron-uptake-deficient strain of Saccharomyces cerevisiae restored growth in the presence of complex Fe(III)-phytosiderophores (Curie et al. Citation2001). The YS1 gene from maize, which encodes a high-affinity Fe(III)- phytosiderophore transporter, has been isolated and characterized (Curie et al. Citation2001). Maize mutants defectives in the ys1 resulted in plants with Fe-deficiency and internerval chlorosis in young leaves (Von Wirén et al. Citation1994; Roberts et al. Citation2004).
Studies on graminaceous species have shown that the release of phytosiderophores was enhanced under copper (Cu) deficiency and even zinc (Zn) deficiency (Gries et al. Citation1998; Oburger et al. Citation2014). Indeed, Chaignon et al. (Citation2002) found that iron deficiency stimulates copper uptake, suggesting that increased copper accumulation may occur through uptake of Cu-Phytosiderophore (PS) complexes. Otherwise, one of the rice (Oryza sativa L.) YS1-like genes, OsYSL2, encodes a rice metal-nicotianamine transporter that may be responsible for the phloem transport of Fe and manganese (Mn; Koike et al. Citation2004). Nicotianamine is not only a key intermediate for the biosynthesis of MA for strategy II plants (Cheng et al. Citation2007), but also an important metal chelator that can facilitate the transport of Fe and other transition metals such as copper, zinc, manganese, cobalt and nickel inside plants (Kim and Guerinot Citation2007; Jeong and Guerinot Citation2009). Overexpression of the barley nicotianamine aminotransferase (NAAT) in rice increased the secretion of MA and consequently enhanced tolerance to low Fe availability (Takahashi et al. Citation2001). However, the mutation in the NAAT gene induced disruption of deoxymugineic acid biosynthesis and accumulation of nicotianamine in rice plants, which stimulated Fe2+ acquisition and increased the accumulation of iron (Cheng et al. Citation2007).
It has been shown that rice possesses not only a strategy II but also a strategy I-like system that may uptake Fe2+ via Fe2+ transporters OsIRT1 and OsIRT2 (Ishimaru et al. Citation2006). Unlike the typical strategy I of other plants, the expression and enzymatic activity of FROs were not detectable in Fe-deficient rice roots (Ishimaru et al. Citation2006), indicating that rice takes up Fe2+ without going through the step of Fe3+ reduction (Cheng et al. Citation2007). Like the IRT1 gene from Arabidopsis, OsIRT1 and OsIRT2 genes are expressed predominantly in roots and are induced in response to Fe deficiency. To adopt a strategy of Fe2+ acquisition can be especially advantageous for rice, since these plants are not very efficient in absorbing Fe3+ only via strategy II.
After being absorbed by roots through the different strategies described, Fe is carried into the xylem and translocated by the transpiration stream to the shoot. The Fe efflux from the symplast into the apoplastic space is the mechanism responsible for the release of Fe into the xylem vessels (Kim and Guerinot Citation2007). In Arabidopsis plants, AtIREG1 (an iron regulated transporter protein) is localized in the plasma membrane of stele cells, suggesting a possible role in the release of Fe into the xylem vessels (Kim and Guerinot Citation2007). In the xylem vessels, Fe is in the oxidized form and is transported in the form of complexes with nicotianamine or organic acids like citrate, which is considered the main metal chelator in the xylem where the pH is around 5.5–6.0 (Curie and Briat Citation2003). However, Ishimaru et al. (Citation2011) described a rice phenolic efflux transporter that controls the concentration of protocatechuic acid, a phenolic compound, in the xylem sap, which appears to be essential for the utilization of apoplasmic precipitated Fe in stele (Yoshino and Murakami Citation1998).
Ferric reductase defective 3 (FRD3), a multidrug and toxin efflux (MATE) family member, is localized in the plasma membrane of cells in the pericycle and vasculature and has a function in iron translocation from roots to shoots by carrying citrate into the xylem (Jeong and Guerinot Citation2009). Roschzttardtz et al. (2011) propose a model in which citrate efflux mediated by FRD3 allows iron solubilization in the apoplastic space separating two tissues without symplastic connections. Therefore, Arabidopsis frd3 nutant shows constitutive expression independent of the Fe supply and, as a consequence, accumulates more Fe in the roots (Rogers and Guerinot Citation2002). Expression analysis of FRD3 and rice FRDL1 genes indicated that the gene transcription is modulated by iron nutrition (Rogers and Guerinot Citation2002; Yokosho et al. Citation2009). For Fe absorption by the mesophyll cells it is also necessary to reduce the metal, which occurs after the release of ferric ion by the citrate molecule, which indicates the existence of a specific Fe2+ transporter located in the plasmalemma of leaf cells. In Fe reduction, the electron donor is the reduced form of Nicotinamide Adenine Dinucleotide Phosphate (NADPH) and this process is induced by the increase in the ratio NADPH/NADP+, as a result of the photochemical phase of photosynthesis (Bruggemann et al. Citation1993). After reduction in mesophyll, Fe is stored in vacuoles or immobilized by the protein ferritin (Briat et al. Citation1995; Briat and Lobréaux Citation1997; Briat et al. Citation2010), which occurs mainly in plastids (Zancani et al. Citation2004).
With the absorption of Fe or Fe-chelate by the roots, the oxidation to ferric form occurs and the metal is translocated into the leaves in the form of electrostatic complexes with citrate (Puig and Peñarrubia Citation2009; Balk and Schaedler Citation2014). In the leaves a reaction of Fe assimilation occurs, catalyzed by the enzyme iron-chelatase; then the Fe is inserted into the porphyrin, which is a precursor of the heme group, present in the cytochromes and located in chloroplasts and in mitochondria (Rutherford et al. Citation2005). In plants most of the Fe is found in the heme groups, although iron-sulfur proteins present in the electron transport chain contain non-heme iron that is covalently bonded to the sulfur (S) atom of the cysteine in the apoprotein. Fe can also be found in the center of Fe2 S2, composed of two Fe and two inorganic sulfides (Jones Citation1983; Hindt and Guerinot Citation2012; Balk and Schaedler Citation2014).
3. Physiology of iron deficiency
Fe deficiency causes chlorosis in plants, which is a nutritional disorder characterized by a significant decrease of chlorophyll in the leaves of plants grown in alkaline conditions and calcareous soils. Fe deficiency decreases the yield of cultures in quantitative and qualitative form, resulting in economic losses (Schenkeveld et al. Citation2008). Soil factors, including high humidity, poor ventilation, high temperatures and high levels of phosphate, may induce or increase chlorosis in the leaves due to a lack of Fe.
Factors such as high pH and high bicarbonate concentrations are generally considered critical to Fe deficiency (Shi et al. Citation1993; Kobayashi et al. Citation2014). The low solubility of Fe oxides and hydroxides in soils and the low bioavailability of Fe in the soil solution at high pH impairs Fe absorption (Marschner et al. Citation2011) or inactivates Fe in the apoplast of leaves. To correct the deficiency of Fe in leaves, chelates of synthetic Fe, like FeEDDHA (ethylene diamine di [o-hydroxyphenylacetic] acid), can be applied, once they increase the solubility of Fe and act as a carrier through a solution inside the plant (Lucena et al. Citation1992).
However, it is known that iron-deficient leaves may have concentrations of iron similar to or even greater than leaves with regular levels of iron, which has been called the “paradox of Fe chlorosis” (Morales et al. Citation1998; Römheld Citation2000).
Fe deficiency in plants stimulates exudation of protons by roots that acidify the soil around them. The release of organic substances like malic and citric acids occurs through exudation of protons in the process of cations absorption, mainly ammonium, which decreases the pH of the solution in the rhizosphere region by increasing the availability of Fe and phosphate. In this process, the enzyme iron-chelating reductase present in the plasma membrane of roots, reduces ferric iron (Fe3+) to its ferrous form (Fe2+), whereas Nicotinamide Adenine Dinucleotide (NADH) or NADPH donates electrons in the process. Thus, several compounds secreted by roots form stable Fe chelates such as malic acid, citric acid, phenolic acid and piscidic acid (Guerinot and Yi Citation1994; Marschner et al. Citation2011).
4. Iron and physiological process
The physiological processes in which Fe has greater importance are photosynthesis and respiration. Although intensively studied, the mechanisms of action of Fe and its ultimate goals in chloroplasts and mitochondria, as well as its equilibrium processes, are not yet well understood as those of other metals like Cu (Puig and Peñarrubia Citation2009). In the chloroplast, there is an indication that the reduction of Fe3+ via FRO family (ferric reductase oxidase) may occur and, in fact, FRO7 is required for incorporation into chloroplasts (Jeong et al. Citation2008). In these organelles, the Fe transport can be mediated by PIC1 permease (permease in chloroplasts 1), which is located inside the chloroplast and is critical to plant development (Duy et al. Citation2007). In mitochondria, the MIT1 importer (mitochondrial iron transporter 1) is essential for the growth and development of plants (Bashir et al. Citation2011).
Although most of the Fe is located in chloroplasts, the vacuole is important for the redistribution of Fe in the early stages of plant development. Fe is imported into the vacuole by vacuolar iron transporter 1 (VIT1; Kim et al. Citation2006) and iron regulated transporter protein-2 (IREG2) transporters (Schaaf et al. Citation2006), and exported to the cytoplasm by natural resistance-associated macrophage proteins NRAMP3 and NRAMP4 (Lanquar et al. Citation2005). The Arabidospis VIT1 was recently identified as a Fe2+ transporter that functions in vacuolar Fe storage (Kim et al. Citation2006), and 62% of VIT1 is similar to its yeast ortholog CCC1p, a transporter that can mediate Fe and Mn efflux into vacuoles (Li et al. Citation2001). On the other hand, IREG2 is involved in Fe-dependent nickel detoxification.
Fe ions also act as cofactors in many enzymatic reactions and are important for several cellular activities (Briat et al. Citation1995). The photosynthetic apparatus of plants is very rich in Fe atoms (21 or 22; Behrenfeld et al. Citation1996), containing two or three atoms of Fe per Photosystem II (PSII), 12 atoms of Fe per Photosystem I (PSI), five Fe atoms by cytochrome complex b6 – f (cyt b6 – f) and two Fe atoms per ferredoxin molecule. In addition, Fe is important as a cofactor for enzymes of thylakoids that eliminate reactive oxygen species. Therefore, photosynthetic organisms are highly sensitive to changes in the availability of Fe, and that is manifested as a marked decrease in photosynthetic activity when exposed to Fe limitation (Molassiotis et al. Citation2006; Briat et al. Citation2007).
During photosynthesis, photoinhibition mechanisms of PSI may occur, because the electrons that are supplied from PSII reduce the oxygen molecules on the receiver side of PSI, which results in the formation of superoxide radicals (O2–), as well as reduction of Fe-S centers, which serve as PSI electron acceptors. Hydrogen peroxide (H2O2) is produced by dismutation of superoxide anion radicals and reacts with reduced Fe in the Fe-S cluster centers to form hydroxyl radicals (•OH-) (Sonoike et al. Citation1997), which destroy the Fe-S centers (Sonoike et al. Citation1995). Therefore, the electron flow is essential for PSI photoinhibition in photosynthetic organisms. However, the low mobility of Fe is probably due to its precipitation in the older leaves in the form of insoluble oxides or phosphates, or to the formation of complexes with phytoferritin, an iron-binding protein found in leaves and other parts of plants (Oh et al. Citation1996).
Fe participates in the detoxification process of reactive oxygen species (ROS) as a constituent of the heme portion of antioxidant enzymes like catalase (CAT), nonspecific peroxidases (POD) and ascorbate peroxidase (APX), and as a co-factor of Fe-superoxide dismutase (Briat and Lobréaux Citation1997). In cases of iron deficiency, the most evident effect in leaves is a generalized chlorosis, caused by reduced chlorophyll that can cause a decrease in CO2 assimilation rate. Also, carotenoids such as β-carotene and neoxanthin suffer reductions in availability. However, lutein and three xanthophylls (violaxanthin, zeaxanthin and anteraxantina) that are part of the xanthophyll cycle are less affected (Donnini et al. Citation2003, Citation2009). A decrease in photosynthetic activity induced by Fe deficiency is associated with decreased light harvesting complexes (LHCs) and electron transport (Pérez et al. Citation1995; Donnini et al. Citation2009).
In chlorotic leaves there is a decrease of thylakoid proteins, and as result PSI is more affected than cytochrome b6–f, which in turn is more sensitive than PSII (Soldatini et al. Citation2000). In contrast, a reduction of chlorophyll decreases the number of photosynthetic units per unit of leaf area. When Fe is added in a medium containing Fe-deficient plants, recovery occurs gradually and both photosynthetic pigments and components of the photosystem are gradually resynthesized (Hecht-Buchholz and Ortmann Citation1986; Pushnik and Miller Citation1989).
The determination of chlorophyll fluorescence has been widely used to examine the photosynthetic performance in different types of plants with iron deficiency, like sugar beet (Beta vulgaris L.) (Belkhodja et al. Citation1998), peach (Prunus persica L.) (Molassiotis et al. Citation2006) and pear (Pyrus communis L.) (Morales et al. Citation2000). Analysis of chlorophyll fluorescence in pear (Morales et al. Citation2000), tomato (Solanum lycopersicum) (Donnini et al. Citation2003) and peach plants (Molassiotis et al. Citation2006) showed a general increase in non-photochemical quenching (NPq) in leaves of Fe-deficient plants, indicating that there is an activation of photoprotective mechanisms, which are not sufficient to avoid photoinhibition process, indicated by a significant reduction in maximum photochemical efficiency of PSII (Fv/Fm) and photochemical quenching (Pq). The quantum efficiency of PSII (ФPSII) and the intrinsic efficiency of PSII (Фexc) showed a similar behavior to the Pq and Fv/Fm rates. Leaves with extreme Fe deficiency show a decrease in PSII efficiency after adaptation to dark, while leaves with moderate chlorosis were less affected (Morales et al. Citation2000). Chlorophyll fluorescence images have been intensively used to study the response of plants to biotic and abiotic stresses (Perez-Bueno et al. Citation2006). This is a noninvasive method that can quantify and analyze the changes induced by stress in the fluorescence emission at the initial stage of stress, as well as detect localized changes in the photosynthetic process.
5. Ecophysiological responses to iron toxicity
Damages caused by Fe toxicity can be direct, through the absorption and excessive accumulation of the element, or indirect, when high levels of Fe in the soil solution result in its precipitation over the roots, forming a crust of ferric oxide, that alters the absorption of other nutrients like P, potassium (K) and Zn (Hansel et al. Citation2001). In situations of excessive accumulation of Fe in tissues, the enhancement of OH• generation occurs by Fenton reaction. Plants have several enzymatic and non-enzymatic mechanisms to protect against oxidative stress caused by excess of Fe (Sinha et al. Citation1997; Vansuyt et al. Citation1997). The enzymatic systems are represented by the enzymes superoxide dismutase (SOD), catalase (CAT) and peroxidase (PO). The non-enzymatic systems consist of organic molecules like glutathione, carotenoids, ascorbate, tocopherol, ubiquinol, uric acid and lipoic acid, which can react directly with the reactive oxygen intermediates (ROIs), neutralizing them (Mitller Citation2002).
Several steps of the metabolism of photosynthetic pigments depend on Fe (Briat et al. Citation2007). However, every time that this metal is absorbed in excess, changes in photosynthetic mechanisms are promoted (Souza-Santos et al. Citation2001; Briat et al. Citation2007), which can affect crop production, as occurs mainly with the rice grown under flood (Fageria et al. Citation2008). When Fe is found free, it can connect with oxygen to form superoxide anions (O2–), which is harmful, because it can damage the membranes by degradation of unsaturated lipidic components. However, plant cells can decrease the damage, by storing the excess of Fe in iron complexes called phytoferritin (Bienfait and Van der Mark Citation1983; Oh et al. Citation1996; Zancani et al. Citation2004; Briat et al. Citation2007, Citation2010). This phytoferritin is composed of a proteic structure with 24 subunits, similar to the form of a hollow sphere, with a molecular weight of about 480 kilodaltons (kDa), and inside the sphere there is a core of 5400–6200 atoms of Fe present as a complex phosphate-ferric oxide.
Fe toxicity in leaves causes necrotic spots, dark roots, inhibition of plant growth (Dobermann and Fairhurst Citation2000) and cationic unbalance, which promotes nutritional imbalances. In rice, excess of Fe has resulted in a reduction in the absorption of P, K, Ca and magnesium (Mg) (Silveira et al. Citation2007). In Beta vulgaris under the effects of Fe toxicity, changes in chloroplast structure were observed, with reduced numbers of grana and stromal lamellae per chloroplast, and of thylakoids per grana, and also reduced numbers of P700 molecules and cytochrome f per unit of area. This pigment disruption of photosynthetic complexes promotes changes in electron transport, which causes reduction in the net assimilation rate of CO2 and a decrease of carbon fixation in chloroplasts (Mishra and Dubey Citation2005).
Rice cultivation is performed mainly in lowlands and in damp and poorly drained soils, which allows direct contact of the root system with the wetland soil. In these culture conditions, Fe toxicity is one of the most important abiotic stresses in crop limitation (Dobermann and Fairhurst Citation2000). During the period of flooding, the diffusion of atmospheric oxygen into the soil is limited, promoting a hypoxic environment. In these cases, the molecular oxygen dissolved in the water is consumed by aerobic microorganisms and, over time, these microorganisms are replaced by anaerobes, resulting in an environment with an excess of CO2 (Grotz and Guerinot Citation2006). These factors lead to a decrease in the pH of the soil solution, which favors the reduction of oxidized metals, like iron, that reduces from Fe3+ to Fe2+, becoming available in high concentration for plants, increasing its potential toxicity condition (Audebert and Fofana Citation2009).
6. Responses to Fe by plants with associate microorganisms
Unlike other plants that have only one strategy to absorb iron (strategy I or strategy II), except in the case of rice, fungi have developed three distinct mechanisms for solubilization and iron uptake: (i) acidification of the medium; (ii) reduction of iron (Fe3+ to Fe2+); and (iii) secretion of soluble iron-chelating molecules. Each fungal species uses these strategies to different degrees and in different ways (Yun et al. Citation2000; Kosman Citation2003; Arantes and Milagres Citation2007; Machuca et al. Citation2007; Adeleke et al. Citation2012). Studies with Saccharomyces cerevisiae Meyen ex E.C. Hancen (1883) show that fungi express two genetic systems for iron absorption, called the reducer system and the non-reducing system (Haas Citation2004). The absorption of Fe3+ in the plasma membrane of yeasts is initiated by the reduction of iron (Fe3+/Fe2+), catalyzed by the enzyme Fre1p. The Fe2+ formed is a substrate for Fet3p, which is part of the high-affinity iron uptake complex. Fe+3 is generated by Fet3p, transported by Fe-permease (Ftr1p) into the cell.
The non-reducing system exclusively recognizes siderophores (De Luca and Wood Citation2000; Kornitzer Citation2009). In low-oxygen conditions, the cells will express a low-affinity ferrous ion transporter Fet4p, which functions as an independent oxygen system for iron transport into the cell. This low-affinity mechanism (which is not regulated by the presence of iron) also transports cobalt, cadmium and nickel (Lemanceau et al. Citation2009; Marschner et al. Citation2011; Shi et al. Citation2011; Nagata et al. Citation2013). Mycorrhizal fungi have different mechanisms to tolerate Fe toxicity, such as the production of melanin, release of siderophores to the external environment, Fe complexation in the cytosol and Fe storage in vacuoles. However, siderophore production is the mechanism used by both mycorrhizal fungi and bacteria for Fe homeostasis (Hall Citation2002; Hall and Williams Citation2003).
7. Conclusions
Environmental contamination by mining industries has affected native species; however, few studies address their impacts on agriculture. Plants can adopt different strategies to absorb Fe from soil solution. Several studies have described the effect of Fe deficiency in physiology and plant nutrition, but with a more ecosystem-oriented view. Therefore, we need more researchers devoted to the understanding of how the iron concentration changes the plant ionome and its correlation with proton and oxygen fluxes in plant roots. The use of biotechnology to generate mutants with over-expression of certain genes involved in iron homeostasis, from plants and microorganisms, could be important in generating more resistant materials to the conditions of lowlands, in which iron availability under anoxic conditions is a real problem, as an alternative production in these environments.
Acknowledgements
We would like to acknowledge Dr. Carlos Eduardo Tadokoro (Universidade Vila Velha, Brazil) for the critical review and helpful suggestions. This work was supported by FAPES and CAPES PhD fellowships conceded to FJE and AAB, respectively, and a CNPq Researcher fellowship conceded to ACR. ACR’s laboratory is supported by FAPERJ (#E-26 /110.081/2014; #E-26 /111.428/2014; #E-26 /111.458/2014) and Conselho Nacional de Desenvolvimento Científico e Tecnólogico (CNPq) (#475436/2010-5; #312399/2013-8) grants. We are grateful to Fundação para a Ciência e Tecnologia (FCT) through the Postdoctoral grant SFRH/BPD/85419/2012 to Teresa Dias.
ORCID
Frederico Jacob Eutrópio http://orcid.org/0000-0001-6393-4976
Amanda Azevedo Bertolazi http://orcid.org/0000-0001-8082-5501
Leonardo Barros Dobbss http://orcid.org/0000-0003-0782-2405
Eliemar Campostrini http://orcid.org/0000-0002-1329-1084
Teresa Dias http://orcid.org/0000-0002-5421-4763
Alessandro Coutinho Ramos http://orcid.org/0000-0002-4082-0076
Additional information
Funding
References
- Adeleke RA, Cloete TE, Bertrand A, Khasa DP 2012: Iron ore weathering potentials of ectomycorrhizal plants. Mycorrhiza, 22, 535–544. doi:10.1007/s00572-012-0431-5
- Arantes V, Milagres AMF 2007: The synergistic action of ligninolytic enzymes (MnP and Laccase) and Fe3+-reducing activity from white-rot fungi for degradation of Azure B. Enzyme. Microb. Tech., 42, 17–22. doi:10.1016/j.enzmictec.2007.07.017
- Audebert A, Fofana M 2009: Rice yield gap due to iron toxicity in west Africa. J. Agron. Crop Sci., 195, 66–76. doi:10.1111/jac.2009.195.issue-1
- Aznar A, Dellagi A 2015: New insights into the role of siderophores as triggers of plant immunity: what can we learn from animals? J. Exp. Bot., 66, 3001–3010. doi:10.1093/jxb/erv155
- Balk J, Schaedler TA 2014: Iron cofactor assembly in plants. Annu. Rev. Plant Biol., 65, 125–153. doi:10.1146/annurev-arplant-050213-035759
- Bashir K, Inoue H, Nagasaka S, Takahashi M, Nakanishi H, Mori S, Nishizawa NK 2006: Cloning and characterization of deoxymugineic acid synthase genes from graminaceous plants. J. Biol. Chem., 281, 32395–32402.
- Bashir K, Ishimaru Y, Shimo H, Nagasaka S, Fujimoto M, Takanashi H, Tsutsumi N, An G, Nakanishi H, Nishizawa NK 2011: The rice mitochondrial iron transporter is essential for plant growth. Nature, 2, 1–7.
- Behrenfeld MJ, Bale AJ, Kolber ZS, Aiken J, Falkowski PG 1996: Confirmation of iron limitation of phytoplankton photosynthesis in the equatorial Pacific Ocean. Nature, 383, 508–511. doi:10.1038/383508a0
- Belkhodja R, Morales F, Quilez R, Lopez-Millan AF, Abadia A, Abadia J 1998: Iron deficiency causes changes in chlorophyll fluorescence due to the reduction in the dark of the Photosystem II acceptor side. Photosynth. Res., 56, 265–276. doi:10.1023/A:1006039917599
- Bienfait HF, Van der Mark F 1983: Phytoferritin and its role in iron metabolism. In D.A. Robb and W.S. Pierpoint (eds.), Metals and Micronutrients: Upake and Utilization by Plants, pp. 111–123. Academic Press, New York.
- Briat JF, Curie C, Gaymard F 2007: Iron utilization and metabolism in plants. Curr. Op. Plant Biol., 10, 276–282. doi:10.1016/j.pbi.2007.04.003
- Briat JF, Duc C, Ravet K, Gaymard F 2010: Ferritins and iron storage in plants. Biochim. Biophys. Acta, 1800, 806–814. doi:10.1016/j.bbagen.2009.12.003
- Briat JF, Fobisloisy I, Grignon N, Lobreaux S, Pascal N, Savino G, Thoiron S, Vonwiren N, Vanwuytswinkel O 1995: Cellular and molecular aspects of iron metabolism in plants. Biol. Cell., 84, 69–81. doi:10.1016/0248-4900(96)81320-7
- Briat JF, Lobréaux S 1997: Iron transport and storage in plants. Trends Plant Sci., 2, 187–193. doi:10.1016/S1360-1385(97)85225-9
- Bruggemann W, Maass-kantel K, Moog PR 1993: Iron uptake by leaf mesophyll cells: the role of the plasma membrane bound ferric-chelate reductase. Planta, 190, 151–155. doi:10.1007/BF00196606
- Chaignon V, Di Malta D, Hinsiger P 2002: Fe-deficiency increases Cu acquisition by wheat cropped in a Cu-Contaminated vineyard soil. New Phyotlogist, 154, 121–130. doi:10.1046/j.1469-8137.2002.00349.x
- Chaney RL, Maillet J, Richarte J, Herrmann P, Remy JC 1998: Relationships between extractable copper, soil properties and copper uptake by wild plants in vineyard soils. Environ. Pollut., 102, 151–161. doi:10.1016/S0269-7491(98)00120-1
- Cheng L, Wang F, Shou H et al. 2007: Mutation in nicotianamine aminotransferase stimulated the Fe(II) acquisition system and led to iron accumulation in Rice1. Plant Physiol., 145, 1647–1657. doi:10.1104/pp.107.107912
- Colangelo EP, Guerinot ML 2006: Put the metal to the petal: metal uptake and transport throughout plants. Curr. Opin. Plant Biol., 9, 322–330. doi:10.1016/j.pbi.2006.03.015
- Conte SS, Walker EL 2011: Transporters contributing to iron trafficking in plants. Mol. Plant., 4, 464–476. doi:10.1093/mp/ssr015
- Curie C, Briat JF 2003: Iron transport and signaling in plants. Annu. Rev. Plant Biol., 54, 183–206. doi:10.1146/annurev.arplant.54.031902.135018
- Curie C, Panaviene Z, Loulergue C, Dellaporta SL, Briat JF, Walker EL 2001: Maize yellow stripe1 encodes a membrane protein directly involved in Fe(III) uptake. Nature, 409, 346–349. doi:10.1038/35053080
- De Luca N, Wood P 2000: Iron uptake by fungi: contrasted mechanisms with internal or external reduction. Adv. Microb. Physiol., 43, 39–74.
- Dobermann A, Fairhurst T 2000: Mineral toxicities. In A. Dobermann and A. Fairhurst (eds.), Rice: Nutrient Disorders & Nutrient Management, pp. 121–125. Potash & Phosphate Institute (PPI), Potash & Phosphate Institute of Canada (PPIC) and International Rice Research Institute.
- Donnini S, Castagna A, Guidi L, Zocchi G, Ranieri A 2003: Leaf responses to reduced iron availability in two tomato genotypes: T3238FER (iron efficient) and T3238fer (iron inefficient). J. Plant Nutr., 26, 2137–2148. doi:10.1081/PLN-120024270
- Donnini S, Castagna A, Ranieri A, Zocchi G 2009: Differential responses in pear and quince genotypes induced by Fe deficiency and bicarbonate. J. Plant Physiol., 166, 1181–1193. doi:10.1016/j.jplph.2009.01.007
- Duy D, Wanner G, Meda A, Von Wirén N, Soll J, Philippar K 2007: PIC1, an ancient permease in Arabidopsis chloroplasts, mediates iron transport. Plant Cell, 19, 986–1006. doi:10.1105/tpc.106.047407
- Fageria NK, Santos AB, Barbosa Filho MP, Guimarães CM 2008: Iron toxicity in lowland rice. J. Plant Nutr., 31, 1676–1697. doi:10.1080/01904160802244902
- Fushiya S, Sato Y, Shigeo N, Nomoto K, Takemoto T 1980: Avenic acid, a new aminoacid possessing na iron chelating activity. Tetrahedron Lett., 21, 3071–3072. doi:10.1016/S0040-4039(00)77410-7
- Grantz DA, Garner JHB, Johnson DW 2003: Ecological effects of particulate matter. Environ. Int., 29, 213–239. doi:10.1016/S0160-4120(02)00181-2
- Gries D, Klatt S, Runge M 1998: Copper-deficiency-induced phytosiderophore release in in the calcicole grass Hordelymus europaeus. New Phytologist, 140, 95–101. doi:10.1046/j.1469-8137.1998.00250.x
- Grotz N, Guerinot ML 2006: Molecular aspects of Cu, Fe and Zn homeostasis in plants. Biochim. Biophys. Acta, 1763, 595–608. doi:10.1016/j.bbamcr.2006.05.014
- Guerinot ML, Yi Y 1994: Iron: nutritious, noxious, and not readily available. Plant Physiol., 104, 815–820.
- Haas H 2004: Molecular genetics of iron uptake and homeostasis in fungi. Biochem. Mol. Biol. Mycota, 3, 3–31.
- Hall JL 2002: Cellular mechanisms for heavy metal detoxification and tolerance. J. Exp. Bot., 53, 1–11. doi:10.1093/jexbot/53.366.1
- Hall JL, Williams LE 2003: Transition metal transporters in plants. J. Exp. Bot., 54, 2601–2613. doi:10.1093/jxb/erg303
- Hansel CM, Fendorf S, Sutton S, Newville M 2001: Characterization of Fe plaque and associated metals on the roots of mine-waste impact aquatic plants. Environ. Sci. Technol., 35, 3863–3868. doi:10.1021/es0105459
- Hecht-Buchholz CH, Ortmann U 1986: Effect of foliar iron application on regreening and chloroplast development in iron chlorotic soybean. J. Plant Nutr., 9, 647–659. doi:10.1080/01904168609363471
- Hindt MN, Guerinot ML 2012: Getting a sense for signals: regulation of the plant iron deficiency response. Biochim. Biophys. Acta, 1823, 1521–1530. doi:10.1016/j.bbamcr.2012.03.010
- Ishimaru Y, Kakei Y, Shimo H, Bashir K, Sato Y, Sato Y, Uozumi N, Nakanishi H, Nishizawa NK 2011: A rice phenolic efflux transporter is essential for solubilizing precipitated apoplasmic iron in the plant stele. J. Biol. Chem., 286, 24649–24655. doi:10.1074/jbc.M111.221168
- Ishimaru Y, Suzuki M, Tsukamoto T et al. 2006: Rice plants take up iron as an Fe3+-phytosiderophore and as Fe2+. Plant J., 45, 335–346. doi:10.1111/j.1365-313X.2005.02624.x
- Jeong J, Cohu C, Kerkeb L, Pilon M, Connolly EL, Guerinot ML 2008: Chloroplast Fe(III) chelate reductase activity is essential for seedling viability under iron limiting conditions. Proc. Natl. Acad. Sci. USA, 105, 10619–10624. doi:10.1073/pnas.0708367105
- Jeong J, Guerinot ML 2009: Homing in on iron homeostasis in plants. Trends Plant Sci., 14, 280–285. doi:10.1016/j.tplants.2009.02.006
- Jones OTG 1983: Ferrochelatase. In D.A. Robb and W.S. Pierpoint (eds.), Metals and Micronutrients: Upake and Utilization by Plants, pp. 125–144. Academic Press, New York.
- Kim S, Punshon T, Lanzirotti A, Li L, Alonso JM, Ecker JR, Kaplan J, Guerinot ML 2006: Localization of iron in Arabidopsis seed requires the vacuolar membrane transporter VIT1. Science, 314, 1295–1298. doi:10.1126/science.1132563
- Kim SA, Guerinot ML 2007: Mining iron: iron uptake and transport in plants. FEBS Lett., 581, 2273–2280. doi:10.1016/j.febslet.2007.04.043
- Kobayashi T, Itai RN, Nishizawa NK 2014: Iron deficiency responses in rice roots. Rice, 7, 27–38. doi:10.1186/s12284-014-0027-0
- Koike S, Inohue H, Mizuno D, Takahashi M, Nakanishi H, Mori S, Nishizawa NK 2004: OsYSL2 is a rice metal-nicotianamine transporter that is regulated by iron and expressed in the phloem. Plant J., 39, 415–424. doi:10.1111/j.1365-313X.2004.02146.x
- Kornitzer D 2009: Fungal mechanisms for host iron acquisition. Curr. Opin. Microbiol., 12, 377–383. doi:10.1016/j.mib.2009.05.005
- Kosman DJ 2003: Molecular mechanisms of iron uptake in fungi. Mol. Microbiol., 47, 1185-1197.2003.
- Kuki KN, Cano MAO, Pereira EG, Costa AC, Cambraia J 2008: Effects of simulated deposition of acid mist and iron ore particulate matter on photosynthesis and the generation of oxidative stress in Schinus terebinthifolius Radii and Sophora tomentosa L. Sci. Total Environ., 403, 207–214. doi:10.1016/j.scitotenv.2008.05.004
- Lanquar V, Lelievre F, Bolte S et al. 2005: Mobilization of vacuolar iron by AtNRAMP3 and AtNRAMP4 is essential for seed germination on low iron. EMBO J., 24, 4041–4051. doi:10.1038/sj.emboj.7600864
- Lemanceau P, Bauer P, Kraemer S, Briat JF 2009: Iron dynamics in the rhizosphere as a case study for analyzing interactions between soils, plants and microbes. Plant Soil, 321, 513–535. doi:10.1007/s11104-009-0039-5
- Li L, Chen OS, Ward DM, Kaplan J 2001: CCC1 is a transporter that mediates vacuolar iron storage in yeast. J. Biol. Chem., 276, 29515–29519. doi:10.1074/jbc.M103944200
- Lucena JJ, Manzanares M, Gárate A 1992: Comparative study of the efficacy of commercial Fe-chelates using a new test. J. Plant Nutr., 15, 1995–2006. doi:10.1080/01904169209364453
- Machuca A, Pereira G, Aguiar A, Milagres AMF 2007: Metal-chelating compounds produced by ectomycorrhizal fungi collected from pine plantations. Lett. Appl. Microbiol., 44, 7–12. doi:10.1111/lam.2007.44.issue-1
- Marschner P, Crowley D, Rengel Z 2011: Rhizosphere interactions between microorganisms and plants govern iron and phosphorus acquisition along the root axis-model and research methods. Soil Biol. Biochem., 43, 883–894. doi:10.1016/j.soilbio.2011.01.005
- Mengel K, Kirkby EA 1987: Principles of Plant Nutrition, pp. 687. International Potash Institute, Worblaufen-Bern, Switzerland.
- Mishra S, Dubey RS 2005: Heavy metal toxicity induced alterations in photosynthetic metabolism in plants. In M. Pessarakli (ed.), Handbook of Photosynthesis, p. 862.
- Mitller R 2002: Oxidative stress, antioxidants and stress tolerance. Trends Plant Sci., 7, 405–408. doi:10.1016/S1360-1385(02)02312-9
- Molassiotis A, Tanou G, Diamantidis G, Patakas A, Therios I 2006: Effects of 4-month Fe deficiency exposure on Fe reduction mechanism, photosynthetic gas exchange, chlorophyll fluorescence and antioxidant defense in two peach rootstocks differing in Fe deficiency tolerance. J. Plant Physiol., 163, 176–185. doi:10.1016/j.jplph.2004.11.016
- Morales F, Belkhodja R, Abadia A, Abadia J 2000: Photosystem II efficiency and mechanisms of energy dissipation in irondeficient, field grown pear trees (Pyrus communis L.). Photosynth. Res., 63, 9–21. doi:10.1023/A:1006389915424
- Morales F, Grasa R, Abadía A, Abadía J 1998: Iron chlorosis paradox in fruit trees. J. Plant Nutr., 21, 815–825. doi:10.1080/01904169809365444
- Nagata T, Oobo T, Aozasa O 2013: Efficacy of a bacterial siderophore, pyoverdine, to supply iron to Solanum lycopersicum plants. J. Biosci. Bioeng., 115, 686–690. doi:10.1016/j.jbiosc.2012.12.018
- Negishi T, Nakanishi H, Yazaki J et al. 2002: cDNA microarray analysis of gene expression during Fe-deficiency stress in barley suggests that polar transport of vesicles is implicated in phytosiderophore secretion in Fe-deficient barley roots. Plant J., 30, 83–94. doi:10.1046/j.1365-313X.2002.01270.x
- Neumann G, Römheld V 1999: Root excretion of carboxylic acids and protons in phosphorus-deficient plants. Plant Soil, 211, 121–130. doi:10.1023/A:1004380832118
- Oburger E, Gruber B, Schindlegger Y, Schenkeveld WDC, Hann S, Kraemer SM, Wenzel WW, Puschenreiter M 2014: Root exudation of phytosiderophores from soil-grown wheat. New Phyotlogist, 203, 1161–1174. doi:10.1111/nph.12868
- Oh SH, Cho SW, Know TH, Yang MS 1996: Purification and characterization of phytoferritina. J. Biochem. Mol. Biol., 29, 415–431.
- Palmgren MG 2001: Plant plasma membrane H+-ATPases: powerhouses for nutrient uptake. Annu. Rev. Plant Physiol. Plant Mol. Biol., 52, 817–845. doi:10.1146/annurev.arplant.52.1.817
- Pérez C, Val J, Monge E 1995: Effects of iron deficiency on photosynthetic structures in peach (Prunus persica) leaves. In J. Abadía (ed.) Iron Nutrition in Soil and Plants, pp. 183–189. Kluwer Academic Publishers, Dordrecht, The Netherlands.
- Perez-Bueno ML, Ciscato M, Vandeven M, Garcia-Luque I, Valcke R, Baron M 2006: Imaging viral infection studies on Nicotiana benthamiana plants infected with the pepper mild mottle tobamovirus. Photosynth. Res., 90, 111–123. doi:10.1007/s11120-006-9098-0
- Puig S, Peñarrubia L 2009: Placing metal micronutrients in context: transport and distribution in plants. Curr. Opin. Plant Biol., 12, 299–306. doi:10.1016/j.pbi.2009.04.008
- Pushnik JC, Miller GW 1989: Iron regulation of chloroplasts photosynthetic function: mediation of PS I development. J. Plant Nutr., 12, 407–421. doi:10.1080/01904168909363962
- Ramos AC, Lima PT, Dias PN, Kasuya MCM, Feijó JA 2009a: A pH signaling mechanism involved in the spatial distribution of calcium and anion fluxes in ectomycorrhizal roots. New Phytologist, 181, 448–462. doi:10.1111/j.1469-8137.2008.02656.x
- Ramos AC, Martins MA, Okorokova-Façanha AL, Olivares FL, Okorokov LA, Sepúlveda N, Feijó JÁ, Façanha AR 2009b: Arbuscular mycorrhizal fungi induce differential activation of the plasma membrane and vacuolar H+ pumps in maize roots. Mycorrhiza, 19, 69–80. doi:10.1007/s00572-008-0204-3
- Ravet K, Touraine B, Boucherez J, Briat JF, Gaymard F, Cellier F 2009: Ferritins control interaction between iorn homeostasis and oxidative stress in Arabidopsis. Plant J., 57, 400–412. doi:10.1111/j.1365-313X.2008.03698.x
- Revathi S, Venugopal S 2013: Physiological and biochemical mechanisms of heavy metal tolerance. Int. J. Environ. Sci., 3, 1339–1354.
- Roberts LA, Pierson AJ, Panaviene Z, Walker EL 2004: Yellow stripe 1. Expanded roles for the maize iron-phytosiderophore transporter. Plant Physiol., 135, 112–120. doi:10.1104/pp.103.037572
- Rogers EE, Guerinot ML 2002: FRD3, a member of the multidrug and toxin efflux family, controls iron deficiency responses in Arabdopsis. Plant Cell, 14, 1787–1799. doi:10.1105/tpc.001495
- Römheld V 2000: The chlorosis paradox: Fe inactivation as a secondary event in chlorotic leaves of grapevine. J. Plant Nutr., 23, 1629–1643. doi:10.1080/01904160009382129
- Roschzttardtz H, Séguéla-Arnaud M, Briat J-F, Vert G, Curie C 2009: The FRD3 citrate effluxer promotes iron nutrition between symplastically disconnected tissues throughout Arabidopsis development. Plant Cell., 23, 2725–2737. doi:10.1105/tpc.111.088088
- Rutherford JC, Ojeda L, Balk J, Mulenhoff R, Lill DRW 2005: Activation of the iron regulon by yeast Aft1/Aft2 transcription factors depends on mitochondrial but not cytosolic iron–sulfur protein biogenesis. J. Biol. Chem., 280, 10135–10140. doi:10.1074/jbc.M413731200
- Santi S, Cesco S, Varanini Z 2005: Two plasma membrane H(+)-ATPase genes are differentially in iorn-deficient cucumber plants. Plant Physiol. Biochem., 43, 287–292. doi:10.1016/j.plaphy.2005.02.007
- Schaaf G, Honsbein A, Meda A, Kirchner S, Wipf D, Von Wiren N 2006: AtIREG2 encodes a tonoplast transport protein involved in iron-dependent nickel detoxification in Arabidopsis thaliana roots. J. Biol. Chem., 281, 25532–25540. doi:10.1074/jbc.M601062200
- Schenkeveld WDC, Dijcker R, Reichwein AM, Temminghoff EJM, Van Riemsdijk WH 2008: The effectiveness of soil-applied FeEDDHA treatments in preventing iron chlorosis in soybean as a function of the o,o-FeEDDHA content. Plant Soil, 303, 161–176. doi:10.1007/s11104-007-9496-x
- Shi S, Richardson AE, O’Callaghan M 2011: Effects of selected root exudate components on soil bacterial communities. FEMS Microbiol. Ecol., 77, 600–610. doi:10.1111/j.1574-6941.2011.01150.x
- Shi Y, Byrne DH, Reed DW, Loeppert RH 1993: Influence of bicarbonate level on iron-chlorosis development and nutrient uptake of the peach rootstock Montclar. J Plant Nutr., 16, 1675–1689. doi:10.1080/01904169309364642
- Silveira VC, Oliveira AP, Sperotto RA, Amaral L, Dias JF, Cunha JB, Fett JP 2007: Influence of iron on mineral status of two Rice (Oryza sativa L.) cultivars. Braz. J. Plant Physiol., 19, 127–139. doi:10.1590/S1677-04202007000200005
- Sinha S, Gupta M, Chandra P 1997: Stress induced by iron in Hydrella verticillata, (I. F.) Royele: response of antioxidants. Ecotoxicol. Environ. Saf., 38, 286–291. doi:10.1006/eesa.1997.1598
- Soldatini GF, Tagliavini M, Baldan B, Castagna A, Ranieri A 2000: Alterations in thylakoid membrane composition induced by iron starvation in sunflower plants. J. Plant Nutr., 23, 1717–1732. doi:10.1080/01904160009382136
- Sonoike K, Kamo M, Hihara Y, Hiyama T, Enami I 1997: The mechanism of the degradation of psaB gene product, one of the photosynthetic reaction center subunits of photosystem I, upon photoinhibition. Photosynth Res., 53, 55–63. doi:10.1023/A:1005852330671
- Sonoike K, Terashima I, Iwaki M, Itoh S 1995: Destruction of photosystem I iron–sulfur centers in leaves of Cucumis sativus L. by weak illumination at chilling temperatures. FEBS Lett., 362, 235–238. doi:10.1016/0014-5793(95)00254-7
- Souza-Santos P, Ramos RS, Ferreira ST, Carvalho-Alves PC 2001: Iron induced oxidative damage of corn root plasma membrane H+-ATPase. Biochim. Biophys. Acta, 1512, 357–366. doi:10.1016/S0005-2736(01)00341-8
- Takahashi M, Nakanishi H, Kawasaki S, Nishizawa NK, Mori S 2001: Enhanced tolerance of rice to low iron availability in alkaline soils using barley nicotianamine aminotransferase genes. Nat. Biotechnol., 19, 466–469. doi:10.1038/88143
- Ueno D, Rombolà AD, Iwashita T, Nomoto K, Ma JF 2007: Identification of two novel phytosiderophores secreted by perennial grasses. New Phytologist, 174, 304–310. doi:10.1111/nph.2007.174.issue-2
- Vansuyt G, Lopez F, Inzé D, Briat J, Fourcroy P 1997: Iron triggers a rapid induction of ascorbate peroxidase gene expression in Brassica napus. FEBS Lett., 410, 195–200. doi:10.1016/S0014-5793(97)00587-5
- Von Wirén N, Mori S, Marschner H, Romheld V 1994: Iron inefficiency in maize mutant ysl (Zea mays L. cv Yellow-Stripe) is caused by a defect in uptake of iron phytosiderophores. Plant Physiol., 106, 71–77.
- Walker EL, Connolly EL 2008: Time to pump iron: iron-deficiencysignaling mechanisms of higher plants. Curr. Opin. Plant Biol., 11, 530–535. doi:10.1016/j.pbi.2008.06.013
- Welch RM, Graham RD 2004: Breeding for micronutrients in staple food crops from a human nutrition perpective. J. Exp. Bot., 55, 353–364. doi:10.1093/jxb/erh064
- Yan F, Zhu Y, Mu C, Zo C, Schubert S 2002: Adaptation of H -pumping and plasma membrane H ATPase activity in proteoid roots of white lupin under phosphate deficiency 1. Plant Physiol., 129, 50–63. doi:10.1104/pp.010869
- Yokosho K, Yamaji N, Ueno D, Mitani N, Ma JF 2009: OsFRDL1 is a citrate transporter required for efficient translocation of iron in rice. Plant Physiol., 149, 297–305. doi:10.1104/pp.108.128132
- Yoshino M, Murakami K 1998: Interaction of iron with polyphenolic compounds: application to antioxidant characterization. Anal. Biochem., 257, 40–44. doi:10.1006/abio.1997.2522
- Yun CW, Tiedeman JS, Moore RE, Philpott CC 2000: Siderophore-iron uptake in Saccharomyces cerevisiae: identification of ferrichrome and fusarinine transporters. J. Biol. Chem., 275, 16354–16359. doi:10.1074/jbc.M001456200
- Zancani M, Peresson C, Biroccio A, Federici G, Urbani A, Murgia I, Soave C, Micali F, Vianello A, Macri F 2004: Evidence for the presence of ferritin in plant mitochondria. Eur. J. Biochem., 271, 3657–3664. doi:10.1111/j.1432-1033.2004.04300.x
- Zhao J, Dong Y, Xie X, Li X, Zhang X, Shen X 2011: Effect of annual variation in soil pH on available soil nutrients in pear orchards. Acta Ecol. Sin., 31, 212–216. doi:10.1016/j.chnaes.2011.04.001