ABSTRACT
To evaluate the influence of reducible iron (Fe) on the availability of silicon (Si) by rice (Oryza sativa L.), (1) relationships between the available Si content in soil (successive water elution method), the Si content in rice shoots and ears, and the levels of oxalate-extractable Si (Sio), Fe (Feo), and aluminum (Alo) in 13 paddy soils were analyzed; and (2) the Si concentration in soil solution following sequential flooding/drainage was traced in soil microcosm experiments. Both the available Si and Feo contents in soil were positively correlated with the Si content in rice shoots, while a similar correlation was not observed between the available Si, Sio and Feo. These results suggest that the Sio adsorbed to Feo is not necessary to determine the pool size of the Si available to plants. In microcosm experiments, intermittent drainages decreased Fe concentration in soil solutions by 83–96% compared to that in the continuous flooding treatment. The oxidation of Fe2+ resulted in the co-precipitation of Si with Fe. However, the decrease of Si in a soil solution following drainage was 22% or less, which were smaller than the values observed when solutions containing various ratios of metasilicic acid and Fe2+ were mixed under oxic conditions (24–97%). In addition, as the Si concentrations in soil solutions in the intermittent flooding treatment increased to the levels similar to those in the continuous flooding treatment after re-flooding before Fe was re-reduced, the effect of reduction/oxidation of Fe on the dissolved Si concentrations was small. Thus, the reducible Fe is not a major factor in rice-available Si in paddy soil.
1. Introduction
Silicon (Si) is the second most abundant element on the Earth, accounting for 28.8% of the Earth’s crust on a weight basis (Wedepohl Citation1995), and is recognized to have various beneficial effects on plant growth (Epstein Citation1999; Ma and Takahashi Citation2002). Si accumulation enhances plant resistance to abiotic stress, such as salinity, metal toxicity, and ultraviolet (UV) irradiation (Ma Citation2004; Liang et al. Citation2007; Seyfferth and Fendorf Citation2012), and biotic stress caused by pests and plant pathogens (Ma Citation2004; Cai et al. Citation2009). It has also been reported that Si reduces lodging and improves leaf and stalk erectness, which can increase the rate of photosynthesis, for rice (Oryza sativa L.) and sugarcane (Saccharum officinarum) (Savant et al. Citation1999; Kyuma Citation2004). The amount of soil Si that is absorbed by irrigated rice is estimated to be 0.2–0.5 t Si ha−1 y−1 (Savant et al. Citation1997), which is 10 times larger than nitrogen (N) absorption and 20 times larger than phosphorus adsorption (Epstein Citation1994).
Table 1. Physicochemical properties of the plow layer soil from paddy fields in Chiba.
Table 2. Si contents in rice shoots and ears as well as Si uptake by rice per unit area in Chiba paddy fields.
Figure 2. Relationships between (a) soil available Si content and Si concentration in rice plants (a) or (b) Feo content in soil.
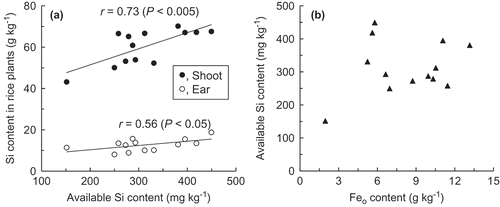
Figure 3. Variations in soil Eh (a, d, g) and concentrations of Fe (b, e, h) and Si (c, f, i) in soil solution in the Kitasuga, Kitahatori and Naganuma-1 soils, respectively. Closed circles indicate intermittent flooding treatment and open circles indicate continuous flooding treatment. Green background indicates the drained period in the intermittent flooding treatment. Vertical bars indicate standard errors.
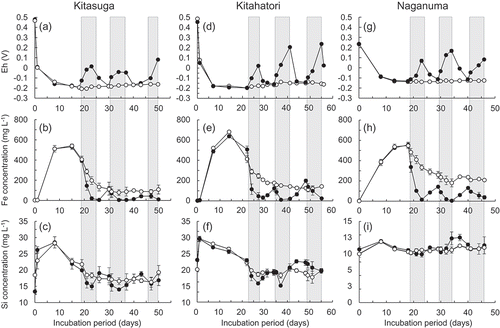
Figure 4. Relationships between (a) the initial Fe concentration and the amount of Si precipitated or (b) the Si in aqueous phase and that in solid phase after solutions containing various ratios of metasilicic acid and Fe2+ were gently stirred for 8 d. Closed squares, open circles, and open triangles in (a) indicate the solutions with Si concentration of 9.1, 17.9 and 35.8 mg L−1, respectively.
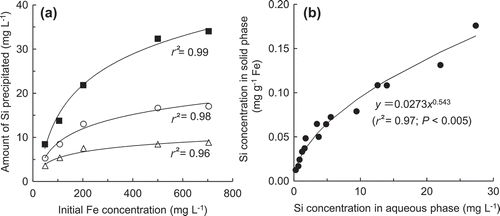
Silicates forming primary and secondary soil minerals are eluted with an advance in chemical weathering. The weathering rate of soil minerals depends on the structural properties, such as crystallinity, size and elemental composition. The dissolution kinetics of silicates is also affected by environmental factors, such as soil temperature, pH and redox potential (Eh) (Savant et al. Citation1997; Icenhower and Dove Citation2000; Gérard et al. Citation2002; Sommer et al. Citation2006). In paddy soil, Si adsorbed to poorly crystalline iron (Fe) or aluminum (Al) oxide/hydroxide has been thought to be the major Si eluted into soil solution (Imaizumi and Yoshida Citation1958; Makabe et al. Citation2009). Dissolved Si is adsorbed preferentially to poorly crystalline Fe rather than crystalline Fe (Delstanche et al. Citation2009), and a part of the poorly crystalline Fe is reducible in a flooded soil (Wahid and Kamalam Citation1993). When the soil Eh decreases to a sufficient level to permit Fe-reducing bacteria to be active, its associated Si becomes available to rice plants (Schwertmann Citation1991; Savant et al. Citation1997; Sauer et al. Citation2006). On the contrary, the oxidation of Fe2+ following the drainage of flood water may induce the co-precipitation of Si in soil solution. Thus, the reducible Fe may play a role as a regulator of Si concentration in soil solution, and the water regime that changes soil Eh may be important for the availability of Si to rice plants.
Because the dissolution rate of Si and the stability of dissolved Si are affected by the form of Si and environmental factors, it is difficult to estimate the amount of Si that is or becomes available to plants during a crop period. In fact, many methods have been introduced (Haynes Citation2014), but none of them is currently accepted as a standard method. Classical acetate buffer (pH 4) extraction (Imaizumi and Yoshida Citation1958) has been considered to overestimate (solubilize some unavailable Si; Takahashi Citation1981; Savant et al. Citation1997), while phosphate buffer (pH 6.2) extraction (Shigezumi et al. Citation2002) likely misses the Si that is dissolved following the development of reducing conditions in the case of rice paddy soils (Kato et al. Citation2002). While Makabe et al. (Citation2009) reported a positive correlation between the available Si content, as estimated by the phosphate buffer extraction method, and the oxalate-extractable Si (Sio) and Fe (Feo) contents for rice paddy soils, Klotzbücher et al. (Citation2015) indicated that none of acetate-, oxalate- or carbonate-extractable Si was related to the amount of Si absorbed by rice. Thus, it is still unclear what is the major existing form of plant available Si in a paddy soil. Although incubation methods such as successive leaching with water (Kitada et al. Citation1992) and a combination of pre-incubation and phosphate buffer extraction (Kato et al. Citation2002) require more time than simple extraction methods do, these methods seem to more accurately reflect the amount of soil Si absorbed by rice plants.
Mihara et al. (Citation2016) investigated the effect of water conservative irrigation (WCI) on the availability of Si to rice plants in both field and microcosm experiments. In WCI, midsummer drainage and intermittent irrigation with minimal amounts of water after midsummer drainage are conducted to reduce water consumption. Conserving water use is important for sustainable rice production under circumstances of increasing industrial water consumption and climate change (Rijsberman Citation2006). Although it would be expected that a considerable amount of Si in soil solution would be lost during intermittent drainage because of co-precipitation with Iron (III) oxide-hydroxide (Fe(OH)3), the seasonal variation in soil solution Si concentration was similar between treatment plots with and without WCI in field experiments, and a microcosm experiment without rice plants also indicated that a small loss of Si in soil solution occurs due to intermittent drainage. However, as the soil release potentials of Si and Fe during a rice growth period can vary among soils, the impact of the water regime on the availability of Si to rice plants may also vary. Irrespective of WCI, a series of water regimes including submergence, midsummer drainage and subsequent intermittent drainage are generally performed in Japan, and an application of water saving systems such as alternate wetting and drying throughout the crop period (AWD; Ye et al. Citation2013) and intermittent irrigation followed by shallow water management (System of Rice Intensification; SRI; Uphoff et al. Citation2011) is becoming more widely used in the world.
The objective of the present study was to evaluate the influence of reducible Fe on the availability of Si by rice. For this purpose, relationships between the available Si content in soil, as estimated by the successive water leaching method, with the Si content in rice shoots and ears at harvest and the content of oxalate-extractable Si (Sio), Fe (Feo) and Al (Alo) in soil were determined. The Feo content was used as approximation of reducible Fe content. The effect of intermittent flooding on the Si and Fe concentrations in a soil solution was also compared using three paddy soil samples with different combinations of available Si and Feo contents in microcosm experiments. To estimate the potential of co-precipitation of Si with Fe during oxidation of Fe2+, a mixing experiment of metasilicic acid and Fe2+ at various ratios was also conducted.
2. Materials and methods
2.1. Soil samples used and analytical methods of soil and plant samples
Soil samples were collected from the plow layer (0–12 cm depth) of 13 paddy rice fields in Chiba Prefecture, Japan, after the 2009 harvest.
The fields are distributed in an area with latitude 35°35ʹ33ʹʹ to 35°51ʹ19ʹʹ N and longitude 140°15ʹ59ʹʹ to 140°29ʹ32ʹʹE. Annual mean temperature and annual precipitation in this area from 2003 to 2010 were 14.6°C and 1547 mm, respectively (Japan Meteorological Agency Citation2015). The same rice cultivar (Oryza sativa L., cv. Koshihikari) was used with a planting density of 14.9–18.4 hills m−2. A chemical fertilizer containing 30 kg nitrogen (N) ha−1 in the form of ammonium sulfate and ammonium phosphate was applied as basal fertilizer, and an additional 10 kg N ha−1 in the form of ammonium sulfate or urea was topdressed. Transplantation and harvesting were conducted on 29 or 30 April and 5 or 6 September, respectively. Midsummer drainage was performed between 15 and 30 June. Rice straw was returned to the field at the harvesting, and silicate was not applied as an amendment to any field. All the soils were classified into Typic Fluvaquents in Soil Taxonomy (USDA Citation2014). Soil samples were collected from five points in each field using a stainless steel shovel, mixed thoroughly, air-dried and sieved (< 2 mm).
The available Si content in the soil samples was determined using the successive water leaching method (Kitada et al. Citation1992). Soil samples (10 g) were suspended in 45 mL of distilled water and placed in an incubator (Model 2–2175, Isuzu Seisakusho, Niigata, Japan) with the temperature regulated at 30°C for 42 d under dark conditions. The flood water (7 cm depth) was replaced with an equivalent weight of fresh distilled water by pipetting once a week. The Si concentration in the flood water was determined by the molybdenum blue colorimetric method (Hallmark et al. Citation1982). The cumulative amounts of Si leached were regressed to a primary function, y = ax (r2 = 0.99–1.00). Examples of elution patterns are shown in . Because the cumulative daily mean temperature during the rice cultivation period in 2009 corresponded to 95 d (average of 94–96 d) at 30°C, the slope of the regression lines (average amount of Si leached per day) was multiplied by 95 (Kitada et al. Citation1992) and normalized to 1 kg of dry soil.
Poorly crystalline Fe and Al as well as Si associated with them were extracted with 0.2 M ammonium oxalate (pH 3.0) (Jackson et al. Citation1986). Fe, Al and Si concentrations in the extracts were determined using an inductively coupled plasma atomic emission spectrometer (ICP-AES; Iris, Nippon Jarrell Ash, Kyoto, Japan). Particle size distribution was measured by a pipette method according to Gee and Bauder (Citation1986).
Determination of the Si content in rice plants was according to Saito et al. (Citation2005). At harvest, the number of tillers for 30 plants chosen randomly was counted, and rice shoots and ears from six plants that had an average number of tillers and were > 5 m apart from each other were collected. The plant samples were gently washed with water, dried in an oven at 70°C for 72 h, weighed and pulverized. The pulverized samples were soaked in 20 mL g−1 of a 1.5 M Hydrogen fluoride (HF)–0.6 M Hydrochloric acid (HCl) solution and allowed to stand for 30 min at 30°C with intermittent stirring. Distilled water was then added at a rate of 80 mL g−1 and the suspension was stirred sufficiently and allowed to settle for 20 mim. An aliquot of the supernatant was collected and the Si concentration was determined by the molybdenum yellow colorimetric method (Hallmark et al. Citation1982).
2.2. Soil microcosm experiment
Three soils with different available Si and Feo contents, Kitasuga, Kitahatori and Naganuma-1 soils, were used. Absorbent cotton was stuffed at the bottom of a glass column (7.2 cm inner diameter × 12 cm high) with a cock at the outlet closed, and 250 mL of ultrapure water and 250 g of a soil sample were added in portions so as to create flooded conditions (six replicates). To simulate the development of reducing conditions in the presence of labile organic matter, such as rice straw, stubble and roots, commercial cellulose and xylan, a model of hemicellulose, were mixed with the soil samples at a rate of 1.25 g kg−1, respectively, beforehand. The soil thickness was 8–9 cm. A soil water sampler consisting of a 5-cm porous cup (6 mmφ) connected to a plastic tube with a stopper at the opposite end (DIK-8393, Daiki, Saitama, Japan) was inserted at the center of the column to a depth of 7 cm. The soil columns were then wrapped with aluminum foil and placed in a room with the temperature regulated at 25°C. During the incubation period of 46–55 d, each of the three columns were maintained under flooded conditions with a floodwater depth of 2–3 cm (continuous flooding treatment). The other three columns were drained after a sufficient duration under reducing conditions (18–22 d) by opening the cock at the outlet for a 4–7-d period and re-flooded (3 cm floodwater depth) by pouring ultrapure water from the column top (intermittent flooding treatment). This drainage procedure was repeated 3 times at intervals of 6–8 d.
During the incubation period, the soil Eh was measured at a depth of 5 cm (pH/ORP meter R-52, HORIBA, Tokyo, Japan) intermittently. Soil solution Fe and Si concentrations were generally measured on the same dates as the soil Eh was recorded. Aliquots of 5 (after drainage) or 10 (under flooded conditions) mL of soil solutions were collected using the soil water samplers, acidified with concentrated Nitric acid (HNO3), and filtered (< 0.45 μm). The reduction in floodwater depth due to the collection of 10 mL soil solution was less than 0.25 cm. Si and Fe concentrations in the soil solutions were determined using ICP-AES.
2.3. Mixing experiment of silica and iron solution
A 588-mg sample of sodium metasilicate (Na2SiO3・9H2O; Wako, Tokyo, Japan) was dissolved in 1 L of ultrapure water and transformed to acid form by reacting with cation exchange resin (Amberlite IR-120, Sigma-Aldrich Japan, Tokyo) using the batch method until no more pH decrease was observed (pH 5.11). The solution was collected by decantation and filtered through a membrane filter (< 0.1 μm) to remove colloidal silica. Aliquots of the resulting solution were diluted to half or one fourth using ultrapure water, and at last three Si solutions containing 13.7, 26.8 and 53.7 mg Si L−1 were obtained. Then a 10-mL aliquot of each Si solution was mixed with 5 mL of a ferrous chloride tetrahydrate (FeCl2 · 4H2O) solution (148, 324, 608, 1500 or 2110 mg Fe L−1) in triplicate. The Si and Fe concentrations in the mixed solutions cover the ranges observed in the soil microcosm experiments. The pH of the sampled solution was regulated at 6.5, the general pH of paddy soils under flooded conditions, using 0.1 M Sodium hydrogen carbonate (NaHCO3) (pH, 7.93) and shaken at 120 rpm for 8 d at 25°C. During the reaction, the pH was maintained at 6.5 by adding 0.1 M NaHCO3 once a day. Although pH became stable after 3 d, suggesting that hydrolysis of Fe3+ was almost completed, the reaction was continued for simulating the duration of intermittent drainage in the microcosm experiments. The solutions were then made up to 50 mL with ultrapure water and filtered (< 0.45 μm). Si and Fe concentrations in the solutions were determined using ICP-AES.
3. Results
3.1. Relationships between available Si content in soil and Si content in rice plant or other soil properties
The available Si content and other properties of the soil samples as well as the Si content of rice shoots and ears for the 13 rice paddy fields are presented in . The available Si content ranged from 151 to 449 mg Si kg−1, which showed significant correlations to the Si contents of both rice shoots, 43.2–70.2 g Si kg−1 (r = 0.73; P < 0.005), and ears, 8.1–15.7 g Si kg−1 (r = 0.56; P < 0.05) (A). The sum of the amounts of Si in rice shoots and ears per m2 (37.0–67.3 g Si m−2; sum of Si uptake in ) also correlated positively to the available Si content (r = 0.56; P < 0.05).
The available Si contents in soil were equivalent to 10–180% (average 62%) of the Sio content, 0.23–2.96 g kg−1, and there was no correlation between them (r = −0.03). The Sio content correlated positively to the content of Alo (r = 0.97; P < 0.005), clay (r = 0.83; P < 0.005) and silt (r = 0.63; P < 0.05) in soil, while the available Si content was correlated with none of the Alo, clay or silt contents. In addition, no relationship was found between the sum of Si uptake by rice and any of the soil properties determined in the present study other than the available Si and Feo contents.
The Feo content ranged from 2.0–13.2 g Fe kg−1, of which a close relationship was observed for the silt and clay content (r = 0.70–0.73; P < 0.01). While the Feo content was also correlated positively with the Si content in rice shoots (r = 0.69; P < 0.01), the correlation with the available Si content was insignificant (r = 0.25; B).
Based on the variations in available Si and Feo contents, the Kitasuga, Kitahatori and Naganuma-1 soils were used for the microcosm experiments. The Kitasuga soil contained larger amounts of available Si and Feo. The available Si content for the Kitahatori soil (449 mg Si kg−1) was similar to that for the Kitasuga soil (395 mg Si kg−1), while the Feo content in the Kitahatori soil (5.8 g Fe kg−1) was almost half that of the Kitasuga soil (11.1 g Fe kg−1). The Naganuma-1 soil had a smaller available Si content (250 mg Si kg−1) and a similar Feo content (6.9 g Fe kg−1) compared to the Kitahatori soil. The Kitahatori and Naganuma-1 soils were also similar in soil texture and the Kitasuga soil was more clayey than the other two soils.
3.2. Behavior of Si in solution during continuously or intermittently flooded incubation (microcosm experiment)
shows variations in soil Eh and Fe and Si concentrations in soil solutions in the Kitasuga, Kitahatori and Naganuma-1 soils, respectively. The soil Eh in the Kitasuga (A) and Kitahatori (D) soils decreased to near 0 V within 1 d after the initial flooding and ranged between −0.1 and −0.2 V from 1 to 3 weeks. The Eh of the Naganuma-1 soil also reached −0.1 V on day 8 (G). Thereafter, a similar Eh level was maintained throughout the incubation period in the continuous flooding treatment. In the case of the intermittent flooding treatment, the soil Eh increased to 0.0–0.2 V following drainage and returned to −0.1 to −0.2 V by re-flooding in all of the soils.
The soil solution Fe concentration in the Kitasuga and Kitahatori soils (B and E) increased abruptly from 1 d after initial flooding. The maximum value, 540 mg Fe L−1 for the Kitasuga soil and 680 mg Fe L−1 for the Kitahatori soil, was recorded on day 15, and the Fe concentration decreased thereafter. The soil solution Fe concentration in the Naganuma-1 soil also increased after the initial flooding, increased to 560 mg Fe L−1 (day 18), and then decreased (H). In the continuous flooding treatment, the Fe concentration during the latter half of the incubation period was in the range of 79–130 mg Fe L−1 in the Kitasuga soil (days 26–50), 110–180 mg Fe L−1 in the Kitahatori soil (days 34–55), and 180–260 mg Fe L−1 in the Naganuma soil (days 29–46). In the intermittent flooding treatment, the Fe concentration decreased to 5–11 mg Fe L−1 (Kitasuga), 11–25 mg Fe L−1 (Kitahatori), or 4.7–38 mg Fe L−1 (Naganuma-1) during 3–6 d after the start of drainage and increased to 120–200 mg Fe L−1 after re-flooding. The maximum decrease in Fe concentration relative to that in the continuous flooding treatment on the same day reached 93–96, 93–94 and 83–90% in the first, second and third drainages, respectively.
In the Kitasuga and Kitahatori soils, the soil solution Si concentration also increased abruptly after the initial flooding, which was much faster than the increase in Fe concentration. The maximum value of 29 or 26 mg Si L−1 was recorded on day 8 (Kitasuga) and day 1 (Kitahatori), respectively, and then slowly decreased (C and F). The decrease in Si concentration in the continuous flooding treatment terminated earlier than that for the Fe concentration, with values of 14–20 mg Si L−1 from day 22 onward for both soils. The soil solution Si concentration in the intermittent flooding treatment decreased further following drainage: from 22–23 to 15 mg Si L−1 at the first drainage and from 17–19 to 14 mg Si L−1 at the second drainage. The Si concentration increased to the level similar to that in the continuous flooding treatment following re-flooding or at the end of the drainage period. The maximum difference (decrease) from the continuous flooding treatment ranged from 15 to 22% when the Si concentration in the continuous flooding treatment on the same day is assumed to be 100%. When the Si concentration in the intermittent drainage treatment at the last record before the drainage was assumed to be 100%, the reduction ranged from 19 to 32%. No decrease in Si concentration was observed at the third drainage.
In the Naganuma-1 soil (I), the soil solution Si concentration remained nearly constant in both treatments, 9–12 mg Si L−1, throughout the incubation period.
3.3. Relationship between Fe concentration and the amount of Si precipitated
A shows the relationship between the initial Fe concentration and the amount of Si precipitated after the Fe2+ and metasilicic acid solutions were gently stirred for 8 d at pH 6.5. When the initial Fe concentration was the same, the decrease in Si concentration in the solution with a higher initial Si concentration was consistently higher. When the initial Si concentration was the same, the amount of Si precipitated was greater in the solution with a higher initial Fe concentration. The proportion of Si decrease during the 8-d mixing was 24–39% at an initial Fe concentration of 50 mg Fe L−1 while it was 95–97% at an initial Fe concentration of 704 mg Fe L−1.
B shows the relationship between the Si concentration in aqueous phase and that in solid phase after mixing with Fe2+ solutions. Their relationship can be expressed using the Freundlich isotherm:
where x indicates the Si concentration in aqueous phase and y indicates the amount of Si precipitated per unit weight of Fe precipitated.
4. Discussion
The findings reported herein indicate that the available Si content in the plow layer soil, as evaluated by the successive water leaching method, is reflected in the Si content in rice plants (A). Makabe et al. (Citation2009) suggested that Sio content reflects the size of the plant-available Si pool on a positive correlation between Sio and phosphate buffer-extractable Si contents. However, a similar correlation was not observed between the Sio and available Si content in the present study. The close relationship between the Sio with Alo contents and the lack of any correlation between the Sio and Feo contents suggest that Alo-associated Si is the dominant form of Sio. A portion of the Alo-associated Si may be eluted into the soil solution with absorption of dissolved Si by plants due to the transition of the equilibrium between solid and solution phases (Imaizumi and Yoshida Citation1958; Sommer et al. Citation2006). On the other hand, even though the amount of the Feo-associated Si is smaller than that of the Alo-associated Si, the release rate of the Feo-associated Si when the Feo is reduced might be greater than that of the Alo-associated Si based on the transition of the equilibrium between solid and solution phases. However, the variation in the available Si content among the soils could not be explained by either or both of Alo and Feo contents. These observations suggest the presence of the other significant sources of plant-available Si. The importance of the Feo-associated Si, which was expected from the positive correlation, is not denied, and probably the contribution from different major sources varies among soils. An accumulation of plant phytoliths and the amount of rice straw returned to soil recently (Seyfferth et al. Citation2013) are also possible sources of the available Si, but confirming this is beyond the objective of the present study.
In the microcosm experiments, the difference in Feo content among the three soils () was not related to the Fe concentration in the soil solution (). As the volume of soil solution in the column was less than 150 mL, the maximum amount of the leached Fe accounted for only 3–7% of the amount of Feo in the three soils. Ferrous ion in the soil solution could be adsorbed to cation exchange sites of soil surface or moved to the floodwater and oxidized there. These processes may control the dissolved Fe concentration after soil Eh decreased under flooding conditions rather than the size of the reducible Fe pool. The gradual decrease in the dissolved Fe concentration from 2 to 4 weeks in the continuous flooding treatment may be due to the oxidation of Fe2+ dispersed at the surface of the floodwater, because oil-like films were observed.
Contrary to the Fe concentration, a difference in the Si concentration in soil solution among the three soils was evident and this difference corresponded to the available Si content in soil. Schwertmann (Citation1991) reported that Si adsorbed on Fe-oxide dissolves easily when soil Eh decreases. An increase in the soil solution Si concentration at the initial Eh decrease was observed in the Kitasuga and Kitahatori soils (). However, a large portion of Si in the soil solution had already been eluted on the day of irrigation, and the maximum Si concentration in the soil solution was recorded earlier than that of Fe. On the other hand, in the Naganuma-1 soil the soil solution Si concentration was increased by only 14–25%. Thus, a sufficient reduction of Fe is likely to be unnecessary for Si to leach into the soil solution. The largest increase in Si concentration following the initial soil Eh decrease in the Kitasuga soil and the highest Si concentration on day 0 in the Kitahatori soil among the three soils examined suggest a difference in the contribution of Si adsorbed to or occluded in Feo to total available Si between the two soils.
The decrease in the Si concentration in the soil solution following drainage was smaller compared to that in the Fe concentration, which was significant only for the Kitasuga and Kitahatori soils at the first and second drainages (). According to the results of the silica–Fe2+ mixing experiments, the proportion of Si precipitated was dependent on the Fe concentration rather than the Si concentration (B). However, a similar Fe concentration at the first and second drainages did not result in similar Si losses between the Kitahatori and Naganuma-1 soils, suggesting that a high Si concentration is required for a significant amount of co-precipitation in the soil solution. The relationship between the Si concentration in the aqueous phase and the amount of Si precipitated per unit weight of Fe precipitated in the silica-Fe2+ mixing experiment is shown in Equation (1). When the Fe concentration just before each drainage in the microcosm experiments was applied to Equation (1), the proportions of Si precipitated for the three soils was estimated to be 100, 80–100 and 25–100% in the first, second and third drainages, respectively. Those values were much greater than the observed values even for the Kitasuga or Kitahatori soils, where the proportions of Si precipitated, estimated as a ratio of the minimum Si concentration to the Si concentration before the drainage, were only 28–32% for the first drainage and 19–26% for the second drainage. In the soil solution, there are components that interact with Fe(III) other than Si, such as phosphate and dissolved organic matter (Maie et al. Citation2001), which may reduce the amount of Si that was co-precipitated with Fe compared to the silica-Fe2+ mixing experiments.
In the intermittent flooding treatment in the microcosm experiments, Si concentration started increasing earlier than Fe concentration after re-flooding and rose to levels similar to those in the continuous flooding treatment within 0–3 d (). This may be due to compensation of Si from soil in order to maintain an equilibrium of Si concentrations between the solid and liquid phases (Sumida Citation1991). The maximum loss of Si in soil solution following drainage in the microcosm experiments was estimated to be 6–7 mg Si kg−1 for both the Kitasuga and Kitahatori soils, based on the amounts of water (250 mL) and soil (250 g) used and the difference in Si concentration from the last record before the drainage (5.7–7.0 mg Si L−1). The value becomes smaller, 3–4 mg kg−1, when the difference in Si concentration between continuous flooding and intermittent drainage treatments (2.6–3.4 mg Si L−1) is used for the calculation. However, these values were equivalent only to 1–2% of the available Si content in the soil sample (395–449 mg Si kg−1; ). Thus, even if the Si that was co-precipitated with Fe was not re-solubilized after re-flooding, Si in soil solutions could be compensated from the water soluble Si pool in soil. If Si in soil solution was lost due not to occlusion or incorporation into Fe hydroxide that was formed during drainage, but only to adsorption on the surface of Fe hydroxide or other minerals, Si could be re-dissolved after re-flooding without waiting for the re-reduction of Fe(III).
5. Conclusions
It was confirmed that the available Si content estimated by the successive water elution method reflects the amount of Si absorbed by rice. The amount of Si associated with Feo did not explain the variation in the available Si content among paddy soils, suggesting the presence of additional major sources of available Si to plants. The effect of the reduction/oxidation of Fe on the dissolved Si concentrations was small. The Si concentration in a soil solution under flooded conditions was related to the available Si content in soil, and a higher Si concentration could result in the significant co-precipitation of Si with Fe following drainage. However, the maximum decrease in Si concentration was 15–22% (vs. the continuous flooding treatment) or 19–32% (vs. just before drainage), which was much smaller than that in the Fe concentration and this value became smaller with repeating drainage. The observed Si decreases in soil solutions were also smaller than the estimated values based on the silica-Fe2+ mixing experiments, and the increases in the Si concentration occurred earlier than those in the Fe concentration after re-flooding. Thus, the present study showed that the reducible Fe is not a major factor in rice-available Si in paddy soil.
Acknowledgments
The authors wish to acknowledge valuable suggestions made by Drs. S. Asakawa, J. Murase and T. Watanabe, Nagoya University.
References
- Cai K, Gao D, Chen J, Luo S 2009: Probing the mechanisms of silicon-mediated pathogen resistance. Plant Signal Behav., 4, 1–3. doi:10.4161/psb.4.1.7280
- Delstanche S, Opfergelt S, Cardinal D, Elsass F, André L, Delvaux B 2009: Silicon isotopic fractionation during adsorption of aqueous monosilicic acid onto iron oxide. Geochim. Cosmochim. Acta, 73, 923–934. doi:10.1016/j.gca.2008.11.014
- Epstein E 1994: The anomaly of silicon in plant biology. Proc Natl. Acad Sci., 91, 11–17. doi:10.1073/pnas.91.1.11
- Epstein E 1999: Silicon. Annu. Rev. Plant Physiol. Plant Mol. Biol., 50, 641–664. doi:10.1146/annurev.arplant.50.1.641
- Gee GW, Bauder JW 1986: Particle-size analysis. In Methods of Soil Analysis. Part 1. Physical and Mineralogical Methods, Ed. Klute, A, 2nd ed. 383–411pp. Am. Soc. Agron. Inc & Soil Sci. Soc. Am. Inc, Madison, WI.
- Gérard F, François M, Ranger J 2002: Processes controlling silica concentration in leaching and capillary soil solutions of an acidic brown forest soil (Rhône, France). Geoderma, 107, 197–226. doi:10.1016/S0016-7061(01)00149-5
- Hallmark CT, Wilding LP, Smeck NE 1982: Silicon. In Methods of Soil Analysis. Part 2. Chemical and Microbiological Properties, Ed. Page, AL, 263–273pp. Am. Soc. Agron. Inc & Soil Soc. Am. Inc, Madison, WI.
- Haynes RJ 2014: A contemporary overview of silicon availability in agricultural soils. J. Plant Nutr. Soil Sci., 177, 831–844. doi:10.1002/jpln.v177.6
- Icenhower JP, Dove PM 2000: The dissolution kinetics of amorphous silica into sodium chloride solutions: effects of temperature and ionic strength. Geochim. Cosmochim. Acta, 64, 4193–4203. doi:10.1016/S0016-7037(00)00487-7
- Imaizumi K, Yoshida S 1958: Edaphological studies on silicon supplying power of paddy fields. Bull Natl. Inst Agric Sci., B8, 261–304. (in Japanese with English summary).
- Jackson ML, Lim CH, Zelazny LW 1986: Oxides, hydroxides, and aluminumsilicates. In Methods of Soil Analysis, Part 1. Physical and Mineralogical Methods Ed. Klute, A, 2nd ed. 101–150pp. Am. Soc. Agron. Inc & Soil Soc. Am. Inc, Madison, WI.
- Japan Meteorological Agency. 2015. Meteorological Data in the Past. http://www.data.jma.go.jp/obd/stats/etm/view/nml-amd-ym (in Japanese).
- Kato N, Kumagai K, Nakagawa F, Sumida H 2002: Comparison of three methods for evaluation of available silicon in paddy soils. Proceeding of the Second Silicon in Agriculture Conference, pp. 58–61. Tsuruoka, Japan. Aug. 22–26, 2002.
- Kitada K, Kamekawa K, Akiyama Y 1992: The dissolution of silica in soil in rotational paddy fields by the surface water dissolution method. Jpn. J. Soil Sci. Plant Nutr., 63, 31–38. (in Japanese with English summary).
- Klotzbücher T, Leuther F, Marxen A, Vetterlein D, Horgan FG, Jahn R 2015: Forms and fluxes of potential plant-available silicon in irrigated lowland rice production (Laguna, the Philippines). Plant Soil., 393, 177–191. doi:10.1007/s11104-015-2480-y
- Kyuma K 2004: Paddy Soil Science. Kyoto Univ Press, Kyoto, Japan.
- Liang Y, Sun W, Zhu YG, Christie P 2007: Mechanisms of silicon-mediated alleviation of abiotic stresses in higher plants: A review. Environ. Poll., 147, 422–428. doi:10.1016/j.envpol.2006.06.008
- Ma JF 2004: Role of silicon in enhancing the resistance of plants to biotic and abiotic stresses. Soil Sci. Plant Nutr., 50, 11–18. doi:10.1080/00380768.2004.10408447
- Ma JF, Takahashi E 2002: Soil, Fertilizer, and Plant Silicon Research in Japan. Elsevier, Amsterdam, The Netherlands.
- Maie N, Watanabe A, Kimura M 2001: Origin and properties of humus in the subsoil of irrigated rice paddies. IV. Effect of water percolation on the binding type of humus in a submerged plow layer soil. Soil Sci. Plant Nutr., 47, 1–8. doi:10.1080/00380768.2001.10408362
- Makabe S, Kakuda K, Sasaki Y, Ando T, Fujii H, Ando H 2009: Relationship between mineral composition or soil texture and available silicon in alluvial paddy soils on the Shounai plain, Japan. Soil Sci. Plant Nutr., 55, 300–308. doi:10.1111/j.1747-0765.2008.00352.x
- Mihara C, Makabe-Sasaki S, Watanabe A 2016: Dynamics of dissolved silicon in rice paddies under conditions of water conservation irrigation. J. Soils Sediments, 16, 547–556. doi:10.1007/s11368-015-1241-0
- Rijsberman FR 2006: Water scarcity: fact or fiction? Agric. Water Manage., 80, 5–22. doi:10.1016/j.agwat.2005.07.001
- Saito K, Yamamoto A, Sa T, Saigusa M 2005: Rapid, micro-methods to estimate plant silicon content by dilute hydrofluoric acid extraction and spectrometric molybdenum method. I. Silicon in rice plants and molybdenum yellow method. Soil Sci. Plant Nutr., 51, 29–36. doi:10.1111/j.1747-0765.2005.tb00003.x
- Sauer D, Saccone L, Conley DJ, Herrmann L, Sommer M 2006: Review of methodologies for extracting plant-available and amorphous Si from soils and aquatic sediments. Biogeochem, 80, 89–108. doi:10.1007/s10533-005-5879-3
- Savant NK, Korndörfer GH, Datnoff LE, Snyder GH 1999: Silicon nutrition and sugarcane production: a review. J. Plant Nutr., 22, 1853–1903. doi:10.1080/01904169909365761
- Savant NK, Snyder GH, Datnoff LE 1997: Silicon management and sustainable rice production. Ad. Agron., 58, 151–199.
- Schwertmann U 1991: Solubility and dissolution of iron-oxides. Plant Soil., 130, 1–25. doi:10.1007/BF00011851
- Seyfferth AL, Fendorf S 2012: Silicate mineral impacts on the uptake and storage of arsenic and plant nutrients in rice (Oryza sativa L.). Environ. Sci. Technol., 46, 13176–13183. doi:10.1021/es3025337
- Seyfferth AL, Kocar BD, Lee JA, Fendorf S 2013: Seasonal dynamics of dissolved silicon in a rice cropping system after straw incorporation. Geochim. Cosmochim. Acta, 123, 120–133. doi:10.1016/j.gca.2013.09.015
- Shigezumi M, Kitta Y, Kubo S, Mizuochi T 2002: The evaluation of the available silica in paddy soil by the phosphate buffer extraction method. Jpn. J. Soil Sci. Plant Nutr., 73, 383–390. (in Japanese with English summary).
- Sommer M, Kaczorek D, Kuzyakov Y, Breuer J 2006: Silicon pools and fluxes in soils and landscapes–a review. J. Plant Nutr. Soil Sci., 169, 310–329. doi:10.1002/(ISSN)1522-2624
- Sumida H 1991: Characteristics of silica dissolution and adsorption in paddy soils application to soil test for available silica. Jpn. J. Soil Sci. Plant Nutr., 62, 378–385. (in Japanese with English summary).
- Takahashi K 1981: Effects of slags on the growth and the silicon uptake by rice plants and the available silicates in paddy soils. Bull. Shikoku Agric. Exp. Stn., 38, 75–114. (in Japanese with English summary).
- Uphoff N, Kassam A, Harwood R 2011: SRI as a methodology for raising crop and water productivity: productive adaptations in rice agronomy and irrigation water management. Paddy Water Environ., 9, 3–11. doi:10.1007/s10333-010-0224-4
- USDA (United States Department of Agriculture). 2014. Keys to Soil Taxonomy. http://www.nrcs.usda.gov/wps/portal/nrcs/detail/soils/survey/class/taxonomy/?cid=nrcs142p2_053580
- Wahid PA, Kamalam NV 1993: Reductive dissolution of crystalline and amorphous Fe(III) oxides by microorganisms in submerged soil. Biol. Fertil. Soils, 15, 144–148. doi:10.1007/BF00336433
- Wedepohl KH 1995: The composition of the continental crust. Geochim. Cosmochim. Acta, 59, 1217–1232. doi:10.1016/0016-7037(95)00038-2
- Ye Y, Liang X, Chen Y, Liu J, Gu J, Guo R, Li L 2013: Alternate wetting and drying irrigation and controlled-release nitrogen fertilizer in late-season rice. Effects on dry matter accumulation, yield, water and nitrogen use. Field Crop Res., 144, 212–224. doi:10.1016/j.fcr.2012.12.003