ABSTRACT
Changes in weather and management practices such as manure and fertilizer applications have a major effect on nitrous oxide (N2O) and nitric oxide (NO) emissions from soils. N2O and NO emissions exhibit high intra- and inter-annual fluctuations, which are also highly influenced by land-use change. In this study we investigated how land-use change between grassland and cornfield affects soil N2O and NO emissions using long-term field measurements in a mollic andosol soil in Southern Hokkaido, Japan. Soil N2O and NO emissions were monitored for 5 years in a 30-year old grassland (OG), which was then plowed and converted to a cornfield for 3 years and then converted back to grassland (new grassland, NG) for another 3 years. We established four treatment plots: control, without any nitrogen (N) input (CT plot); chemical fertilizer only (F plot); chemical fertilizer and manure (MF plot); and manure only (M plot).
Changing land use from OG to cornfield increased annual N2O emissions by 6–7 times, while the change from cornfield to NG resulted in a 0.3–0.6 times reduction in annual N2O emissions. N2O emissions in the newly established grassland were 2–5 times higher than those in the 30-year old grassland. Soil mineral N (NO3− and NH4+) was higher in cornfield, followed by NG and lowest in OG, while water extractable organic carbon (WEOC) did not significantly change with changing land use but tended to be higher in OG and NG than in cornfield. The ratio of WEOC to soil NO3− was the most important explanatory variable for differences in N2O emissions as land use changed. High N input, surplus soil N, and precipitation and low soil pH led to increased N2O emissions. N2O emissions in fertilizer- and/or manure-amended plots were 3–4, 2–5 and 1.4–2 times higher than those in the control treatment in OG, cornfield and NG, respectively. NO emissions were largely influenced by soil mineral N and N addition, and showed less response to changing land use. There were high inter-annual variations in both NO and N2O emissions in all plots, including the control treatment, highlighting the need for long-term measurements when determining local emission rates.
1. Introduction
Nitrous oxide (N2O) is an important greenhouse gas (IPCC Citation2007; UNEP Citation2013) and is currently the most important substance emitted into the atmosphere causing the depletion of the ozone layer (UNEP Citation2013), whereas nitric oxide (NO) is a highly reactive trace gas important in atmospheric chemistry as it contributes to acid rain deposition and for its regulation of photochemical production of ozone in the troposphere (Crutzen Citation1979; Logan Citation1983; Davidson et al. Citation1993; Eickenscheidt and Brumme Citation2013).
The largest source of anthropogenic N2O emissions is agricultural soils, accounting for about 66% of gross anthropogenic emissions (UNEP Citation2013). N2O and NO emissions from soils and agricultural systems are expected to increase further due to increased use of nitrogen (N) fertilizers and manure to meet demand for increased food production (Mosier and Kroeze Citation2000; FAO Citation2003; US EPA Citation2006; Smith et al. Citation2007; Ciais et al. Citation2013; UNEP Citation2013). Microbial transformation of chemical N is an important source of both N2O and NO emissions (Vitousek et al. Citation1997; Medinets et al. Citation2015). While agricultural soils are not considered to be the major source of NO globally, they are still very important sources, especially when fossil fuels are not considered (Bouwman et al. Citation2002a). Tillage and manure application can increase NO emission by up to 7 times, and as much as 11% of applied fertilizer N can be emitted as NO (Skiba et al. Citation1997).
It is generally accepted and widely reported that N2O and NO emission are increased by N fertilization (Jin et al. Citation2010; Vanderzaag et al. Citation2011; Shimizu et al. Citation2013; Owen et al. Citation2015). However, some studies have reported possible reductions in N2O emissions with improved management of organic materials (Alluvione et al. Citation2010; Ryals and Silver Citation2012; UNEP Citation2013). Changing fertilizer type to those less susceptible to nitrification, timing of fertilization and use of organic N sources could help mitigate NO emissions (Davidson et al. Citation1993; Skiba et al. Citation1997; Smith et al. Citation1997). There are still many unknowns and high uncertainties in estimating representative annual N2O and NO emissions from an individual site, as emissions from the same site greatly vary from year to year.
In grasslands, large amounts of N accumulate in plant biomass, resulting in N-rich organic matter over time (Davies et al. Citation2001; Shepherd et al. Citation2006; Velthof et al. Citation2010). When grasslands are plowed, there is increased soil available N, as this N is mineralized (Whitehead et al. Citation1990; Necpa ́lova ́ et al. Citation2013), resulting in increased N losses through leaching (Whitehead et al. Citation1990; Necpa ́lova ́ et al. Citation2013) and N gas emissions (Smith and Conen Citation2004; Oenema et al. Citation2005; Smith et al. Citation2007; UNEP Citation2013). Compared to grasslands, croplands are plowed annually, increasing the physical breakdown of soil structure and organic matter, and soil aeration, and consequently leading to rapid microbial decomposition of organic matter (Ussiri and Lal Citation2009; Necpa ́lova ́ et al. Citation2013). Assessing how N2O and NO emissions change when land use is alternated between grassland and cropland is important to fully understand the potential for mitigation of the emissions during the transition from one land use to the other.
Freezing and thawing can stimulate N2O and NO emissions (Katayanagi and Hatano Citation2012; Burchill et al. Citation2014) by releasing carbon (C) and N through microbial lysis, and through physical entrapment and release during soil freezing and melting. However, the contribution of winter and thawing periods to annual N2O and NO emissions, and its annual variation, are not well known.
Long-term field data on N2O and NO emissions is currently scarce (Tubiello et al. Citation2013). In this study we report results of continuous monitoring of N2O emissions for 11 years following manure and chemical fertilizer applications, combined with changing land use. While many studies have reported the effects of fertilizer, manure and land-use change on N2O emissions, very few, if any, have measured continuously the changes in the soil properties and N2O emissions for as long as 11 years with changing land use, covering a permanent grassland, a cornfield and a newly established grassland. The objectives were: (1) to assess the effect of long-term manure and chemical fertilizer applications on N2O and NO emissions; (2) to investigate the effect of land-use change (from grassland to cornfield and back) on N2O and NO emissions; (3) to investigate the factors driving intra- and inter-annual variations in N2O and NO emissions; and (4) to quantify the contribution of winter and thawing periods to annual N2O and NO emissions.
2. Materials and methods
2.1. Study site
This study was carried out at the Hokkaido University Shizunai experimental livestock farm of the Field Science Center for Northern Biosphere in Shin-Hidaka city, Southern Hokkaido, Japan (42°26ʹN, 142°29ʹE). The site is relatively cool in summer and cold in winter, with average annual air temperature and precipitation values of 8.1°C and 1252 mm, respectively. The soil surface is covered with snow from the end of December to the beginning of March. The soil is derived from Tarumae (b) volcanic ash (Jin et al. Citation2010; Shimizu et al. Citation2010), and is classified as Mollic Andosol (IUSS Working Group WRB Citation2006).
2.2. Field experimental designs and plot management
During the study period, land use was as an old grassland (OG) from 2005 to 2009, cornfield (2010–2012) and newly established grassland (NG, 2013–2015). The old grassland had been established more than 30 years prior to the beginning of this study in 2005. The dominant grass species was reed canary grass (Phalaris arundinacea L.) and meadow foxtail (Alopecurus pratensis L.) in OG, and timothy grass (Phleum pretense L.) in NG.
The average amount of mineral fertilizer applied in OG before commencement of this study was 133 ± 36 kg N ha−1 year−1. From 1990 to 2004, the grassland was harvested for hay at least twice a year. In September 2009 herbicide was applied, and the field was plowed in December.
Three treatment plots, namely (1) control without N addition (CT plot), (2) chemical N fertilizer only (F plot), and (3) chemical N fertilizer and composted beef cattle manure (MF plot), were set up in 2005. In 2011, a fourth plot with composted beef cattle manure only (M plot) was added. Each plot was 5 × 5 m in size, and all treatment plots were replicated 4 times and arranged as shown in Fig. S1. The treatment plots for this study were set up within a large 2-ha field as shown in Fig. S1 and as previously described by Shimizu et al. (Citation2010).
shows the timing of fertilizer and manure applications, and other management practices. The type of chemical fertilizer was ammonium sulfate and the manure was composited beef cattle manure with bedding litter (bark). The gross manure N and fertilizer N application rates were as shown in . Lime was applied in all plots from 2008 to 2015 at an average rate of 400 kg CaCO3 ha−1 year−1.
Table 1. Timing and kind of field management activities.
Table 2. Manure and chemical fertilizer nitrogen (N) application rates from 2005 to 2015.
2.3. Soil and weather measurements
Soil samples were collected at 5 cm depth during each sampling day from April to November (non-freezing period) in all treatment plots. Soil samples were sieved (2-mm sieve) and extracted in deionized water or in 2 M potassium chloride (KCl) solution, and the extracts stored at 4°C until analysis for dissolved nutrients after being filtered through 0.2-μm membrane filters. From the water extracts, soil nitrate (NO3−) concentrations were analyzed by ion chromatography (Dionex QIC Analyzer; Dionex Japan, Osaka, Japan); soil pH was measured using a combined electrode pH meter (F-8 pH meter; Horiba, Kyoto, Japan); and water extractable organic carbon (WEOC) was measured using a total organic carbon (TOC) analyzer (TOC 5000A; Shimadzu, Japan). Ammonium nitrogen (NH4+-N) in the 2 M KCl extract solution was determined using the indophenol blue method (UV mini 1240; Shimadzu, Kyoto, Japan).
In OG and NG, soil moisture was measured at 0–6 cm depth using the frequency domain reflectometry (FDR) method (DIK-311A; Daiki, Saitama, Japan). Calibration curves were made to calculate water-filled pore space (WFPS) from the FDR device reading and percent total porosity (Linn and Doran Citation1984; Jin et al. Citation2010). In the cornfield, soil moisture content was measured gravimetrically from soil samples collected at a depth of 0–5 cm.
Daily precipitation and air temperature were obtained from the nearest Automated Meteorological Data Acquisition System (AMEDAS) station of the Japan Meteorological Agency. Thermocouple thermometers (TR-52, TD, Nagano, Japan) were permanently installed in each plot to measure soil temperature at 5 cm depth at 30-min intervals. On each sampling day air temperature inside the chamber and soil temperature (5 cm depth) were measured using a hand-held thermometer (CT220; CUSTOM, Tokyo, Japan).
2.4. Gas flux sampling and measurement
N2O and NO fluxes were measured using static closed chambers. The chambers were made of stainless steel and were 20 cm in diameter and 25 cm in height in the cornfield, and 40 cm wide and 30 cm high in OG and NG. Details of the chambers were as reported by Toma and Hatano (Citation2007). The chambers were placed onto chamber bases, which were installed permanently during the measurement period to a depth of 5 cm. Chamber bases could not be used in winter; therefore, in winter chambers were inserted directly to 5 cm depth a day before measurements. We did not remove the snow during winter measurements. After each sampling the chambers were removed from the bases.
Gas samples were taken between 8:00 am and 12:00 pm on each sampling day using a gas-tight syringe through a three-way valve fitted onto the chamber cover. The normal sampling frequency was once or twice every fortnight, except in winter when sampling was conducted once or twice every month. A more intensive sampling regime of every 2 to 5 d was carried out after fertilization and other events that are known to stimulate gas flux. Gas samples from the headspace of each chamber were collected into pre-vacuumed Tedlar bags for NO analysis or a 20-mL vial bottle for N2O. Samples were taken at 0 and 30 min in OG, 0 and 20 min in cornfield and 0, 15 and 30 min in NG after chamber closure. To check the accuracy of flux calculated using only two headspace concentrations, we compared the slope of the change of N2O concentration inside the chamber over time using the three headspace concentrations (at 0, 15 and 30 min) and using two headspace concentrations (at 0 and 30 min) for all chambers in the 2013–2015 period (n = 772). The results showed that the slopes from the three and two headspace concentrations had a 1:1 linear relationship (R2 = 0.9997). We then compared the slopes of three and two headspace concentrations when N2O was low (below the median), when it was high (above the median) and using the whole data set, and there was no significant difference among the three regression lines (F = 0.0018, p = 0.9981). This result indicates that flux from the two headspace concentrations could be used for treatment comparisons (De Klein et al. Citation2003; Stolk et al. Citation2009; De Klein Citation2015).
NO gas concentrations were analyzed in the laboratory within the same day of sampling using a nitrogen oxides (NOx) analyzer (Model 265P; Kimoto Electric, Osaka, Japan). N2O gas concentrations were analyzed within 3 months using a gas chromatograph fitted with an electron capture detector (Model GC-14B; Shimadzu, Kyoto, Japan). NO and N2O concentrations in the samples were calculated using calibration curves made with standard gases. The concentrations of standard gases used were 0.3, 0.6, 0.9, 2.8, 6.2, 9.3 and 30.9 ppm for N2O, and 0.01, 0.02, 0.04, 0.1, 0.2, 0.4, 1 and 2 ppm for NO.
The gas flux from the soil was calculated using the following linear regression equation (Katayanagi and Hatano Citation2012):
where F is the gas flux in μg m−2 hr−1; ρ is the density of each gas at standard conditions (N2O = 1.97 × 106 mg m−3, and NO = 1.34 × 106 mg m−3); V is the volume of the chamber (m3); A is the surface area of the chamber (m2); Δc/∆t (10−6 m3 m−3 h−1) is the ratio of change in gas concentration in the chamber during the sampling time; T is the air temperature inside the chamber (°C); and α is the ratio of molar mass of N of the molecular weight of each respective gas.
Cumulative annual emissions were calculated by linear interpolation between sampling events and numerical integration of underlying area using the trapezoid rule (Whittaker and Robinson Citation1967; Ussiri et al. Citation2009). The winter period was defined as the period from mid-December, when maximum soil temperature fell below 5°C, to the end of February when maximum temperatures recorded reached 0°C. The thawing period was defined as the period from when minimum daily temperatures reached 0°C, to the time when soils were completely melted (minimum soil temperatures ~5°C) (Kurganova et al. Citation2007; Katayanagi and Hatano Citation2012).
2.5. Heterotrophic soil respiration and estimation of mineralized N
Heterotrophic respiration (RH) was measured as carbon dioxide (CO2) emission from bare soil (plant- and root-excluded soil) as described by Limin et al. (Citation2015). Bare plots were established as described by Shimizu et al. (Citation2009). Briefly, the aboveground plants and root were removed, and a root-proofing sheet (BKS9812; TOYOBO, Osaka, Japan) was vertically inserted from the soil surface to 30 cm depth to inhibit regrowth of roots.
RH was measured using the closed chamber method as described in section 2.4. RH was measured in the CT plot from 2005 to 2009, and in all plots from 2010 to 2015. RH in CT and F plots was regarded as heterotrophic respiration from soil organic matter decomposition (RHs), while RH from manure amended plots included RHs and heterotrophic respiration from manure decomposition (RHm). Therefore, RHm in MF was estimated by subtracting the RH from the F plot, while it was calculated in the M plot by subtracting the RH from the CT plot. From 2005 to 2009, RHm was calculated as the difference in total CO2 emissions in planted plots between the MF and F plots (Li et al. Citation2015; Shimizu et al. Citation2015).
The total mineralized N was calculated as the sum of soil organic matter N and manure N mineralization. The mineralized N from soil organic matter and manure was calculated by dividing RHs and RHm by the soil and manure C/N ratios, respectively.
2.6. Plant N uptake, total N input and soil surplus N
Net primary production (NPP) was measured as the net increase in plant biomass (aboveground and belowground biomass) annually (Shimizu et al. Citation2015).
In grassland the plant biomass was collected 4 times in a year in April, June, August and October, as described by Shimizu et al. (Citation2009). The aboveground biomass was manually harvested by cutting all the plant biomass within a 0.5 m × 0.5 m quadrat. Two aboveground samples were collected and averaged for each of the four treatment replicates during each sampling event. The belowground biomass was measured by taking a soil block (0.25 × 0.25 × 0.30 m) at each of the four replications, from the same points where the aboveground biomass was collected, and then manually separating the roots from the soil.
In cornfield the plant biomass was collected once a year, at the end of the growing season just before harvesting. For each of the four treatment replications, corn plants within a 1.5 m × 1 m area were collected by uprooting them (by digging) to 30 cm depth to include all the roots for each plant.
Plant roots were washed in water using a 0.5-mm sieve to completely remove the soil particles and other debris. The plant samples were oven dried at 70°C for more than 72 h and weighed. Each dried sample was analyzed for total C and N contents with an N/C analyzer (SUMIGRAPH NC–1000, Sumika Chemical Analysis Service, Ltd., Osaka, Japan).
Surplus soil N was calculated as the difference between total N input (sum of soil and manure mineralized N and chemical fertilizer N) and plant N uptake.
2.7. Data analysis
Statistical analysis was done using STATA-13 (Stata corporation, Texas, USA). Two-way analysis of variance (ANOVA) was used to evaluate the differences in annual fluxes across years and treatments within each land use. One-way ANOVA was used to assess the differences in annual N2O emissions and chemical properties among the land uses for each treatment. Annual N2O and NO data were natural log transformed [y = log (x + 1)] before ANOVA. The value 1 was added to prevent generation of negative log transformed values.
Pearson’s correlation test was used to test the relationship between weather and soil variables with N2O fluxes and cumulative annual emissions. Step-wise single and multiple regression analyses were used to explain the influence of soil and environmental variables on annual N2O and NO emissions.
3. Results
3.1. Soil and weather variables
Mean annual air temperatures were within long-term normal values for most of the years during this study except for 2007, which was recorded at 0.7°C higher than the long-term average value of 8.2°C. The coolest as well as the driest year was 2005 (8.0°C, 999 mm). The years 2009, 2010, 2011 and 2013 were wetter than average with annual precipitation at least 200 mm higher than the 30-year average of 1252 mm.
Soil nitrate (NO3−) and ammonium (NH4+) concentrations were significantly higher in cornfield than grassland and higher in NG than OG (p < 0.01; ). NO3− significantly increased in 2010 after converting grassland to cornfield, and decreased slightly in 2011 and 2012. In the first year after conversion from grassland to cornfield, NO3−N concentration in control plot (without N addition) increased from an average of 1 to 60 mg kg−1, but decreased to 12 mg kg−1 by the third year of the cornfield () and declined further in the new grassland. NH4+ concentration, on the other hand, did not increase in the first year of cornfield but showed high values in 2012, the third year of cornfield. Soil NO3− and NH4+ concentrations were higher in chemical fertilizer-amended plots (MF and F) compared to CT and M plots in all three land uses throughout the study period, and were always lowest in the control treatment. Peaks of both soil NO3− and NH4+ concentrations were observed following chemical fertilizer applications in spring, and short-lived peaks in NO3− concentrations were sometimes observed after manure application.
Figure 1. Soil nitrate nitrogen (N), ammonium N and water extractable soil organic carbon (WEOC). CT is control plot; F is chemical fertilizer plot; MF is combined chemical fertilizer and manure plot; M is manure only plot. Dashed arrows indicate dates of manure application; solid arrows with open V-shaped tip indicate dates of chemical fertilizer application; solid arrows with round top and normal closed tip indicate dates of plowing.
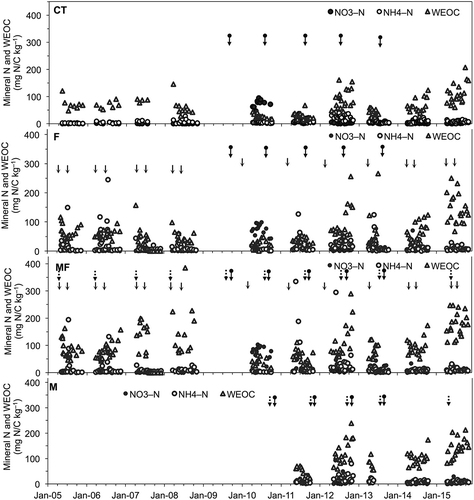
WEOC did not change much with changing land use but tended to be higher in OG and NG compared to cornfield. WEOC was significantly lower in 2010, the first year of conversion from grassland to cornfield, and increased annually in the 3 years of cornfield. WEOC was higher in the manure-amended plots (MF and M) than in the plots without manure application (p < 0.01).
The ratio of WEOC to NO3− was highest in OG, followed by NG, and lowest in cornfield in all of the plots.
Soil pH was always lower in F plot compared to MF, M and CT plots (p < 0.001). Soil pH in MF and M (long-term manure application) was higher than that in CT plot. Soil pH in all plots increased annually from 2008 due to liming.
3.2. Temporal variations of N2O fluxes
Nitrous oxide fluxes were very episodic and displayed high variations within and across years throughout the study period (). Intra-annual variations were highly influenced by mean daily temperature and precipitation.
Figure 2. Daily precipitation and air temperature and daily N2O flux. CT is control plot; F is chemical fertilizer plot; MF is combined chemical fertilizer and manure plot; M is manure only plot. Dashed arrows indicate dates of manure application; solid arrows with open V-shaped tip indicate dates of chemical fertilizer application; solid arrows with round top and normal closed tip indicate dates of plowing.
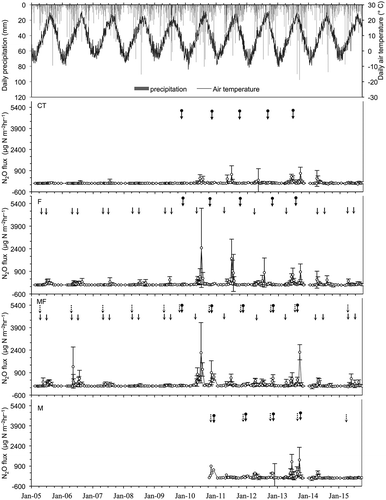
The timing when the highest fluxes were found differed depending on the land use and fertilizer application. In OG, the highest fluxes in the MF plot—275.5, 1290.6, 140.3, and 93.5 µg N2O-N m−2 hr−1—were found on 20, 11, 29 and 18 May in 2005, 2006, 2007 and 2009, respectively. All of these followed combined chemical fertilizer and manure applications in spring, except in 2008 when the highest flux (71.7 µg N2O-N m−2 hr−1) was found on 14 July after the second fertilizer application. In the F plot the highest fluxes—313.8, 211.1, 206.8, 175.7 and 333.8 µg N2O-N m−2 hr−1—were found after the second fertilization on 18, 15, 18 and 7 July and 25 June in 2005, 2006, 2007, 2008 and 2009, respectively. This was despite the lower N application rate in the second application compared to the first one in May. In the control plot, the highest fluxes in OG—50.3, 66.2, 114.5, 22.8 and 30.4 µg N2O-N m−2 hr−1—in 2005, 2006, 2007, 2008 and 2009, respectively, were always found between July and August, and were all preceded by cumulative precipitation of more than 40 mm within 7 d before sampling. In cornfield (2010–2012) the highest fluxes in all treatment plots were found in either June or July and were preceded by high precipitation. The highest fluxes in cornfield ranged from 223.7 to 638.4 µg N2O-N m−2 hr−1 in control plot, 822.1 to 2461.4 µg N2O-N m−2 hr−1 in the F plot, and 527.4 to 2223.5 µg N2O-N m−2 hr−1 in the MF plot.
In 2013, the first year of new grassland (but before it was well established), disproportionately high fluxes (713.8, 871.0, 2260.9 and 1359.2 μg N2O–N m−2 hr−1 in CT, F, MF and M plots, respectively) were found on 18 September, 2 d after very high precipitation (97 mm in 1 d) on 16 September. On 5 and 20 June in 2013, 54.3 and 40 mm rainfall was recorded, respectively, and high fluxes were found for samples collected within 5 d. Precipitation higher than 40 mm per day was recorded at least 6 times in 2014 and 2015, but the fluxes were relatively low.
In all of the plots, winter N2O emissions were very low throughout the study. High fluxes during the thawing period were found in all plots throughout the study period.
Nitrous oxide fluxes were highest in the cornfield, followed by NG, and lowest in OG ( and ). Nitrous oxide fluxes in chemical fertilizer-amended plots (F, MF) were higher than those without chemical fertilizer application (p < 0.01). The manure-only plot tended to have higher emissions than the control plot.
Table 3. Annual nitrous oxide (N2O) emissions (mean ± standard deviation, SD) from 2005 to 2015 in unfertilized control plots (CT), chemical fertilizer plot (F), manure and chemical fertilizer plot (MF) and manure plot (M).
Inter-annual variations in cumulative N2O emissions were more pronounced in cornfield and NG than in OG (). Annual N2O emissions were lower in OG, followed by NG, and highest in cornfield (). Averaged over the entire study period for each land use and compared within each treatment, annual N2O emissions in cornfield were 6–7 times higher than in OG (p < 0.001) and 1.5–3 times higher than in NG (). The emissions in NG were 2–5 times higher than those in OG (p < 0.001).
The emissions in the cornfield were highest in the first year after conversion from grassland (2010) and lowest in the third year. After conversion from cornfield to NG, the annual emissions declined slightly in 2013 (first year of conversion), but by the second and third years after conversion, the emissions in NG were significantly lower than in the cornfield. Within each land-use type, there were significant differences in annual N2O emissions among plots and among years (p < 0.01).
3.2.1. Contribution of winter and thawing periods to annual emissions
In grassland (both OG and NG), contributions of winter N2O emissions to annual emissions ranged from 0 to 7% in all plots, except for 2008 where winter emissions in the CT plot accounted for 25% and 2015 where cumulative winter emissions in F and CT plots were negative (). In cornfield, winter emissions in CT and F plots contributed 2–18%, while in the manure-amended plots, winter emissions contributed as much as 35% to the total annual emissions.
Table 4. Winter nitrous oxide (N2O) emissions (kg N ha−1) and their contribution to total annual emissions in brackets (%).
The thawing period tended to make a higher contribution to annual emissions in the unfertilized control treatments (). In 2014, the thawing period accounted for more than 45% of total annual emissions in all plots.
Table 5. Nitrous oxide (N2O) emissions during the thawing period (kg N ha−1), and their contribution to total annual emissions in parentheses (%).
3.3. Temporal variations of NO fluxes
Intra-annual variations of NO fluxes showed a similar trend to N2O fluxes. However, the NO fluxes were very low throughout the study period, with only the MF plot showing higher values (). The highest NO fluxes were always found after fertilizer and manure applications.
Figure 3. Daily nitric oxide (NO) flux. CT is control plot; F is chemical fertilizer plot; MF is combined chemical fertilizer and manure plot; M is manure only plot. Dashed arrows indicate dates of manure application; solid arrows with open V-shaped tip indicate dates of chemical fertilizer application; solid arrows with round top and normal closed tip indicate dates of plowing.
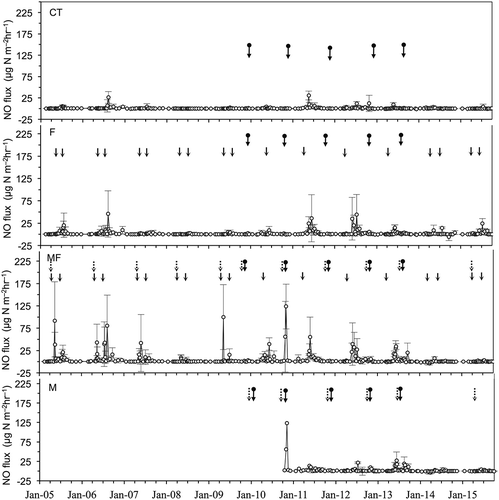
Annual NO emissions were higher in MF and F plots and lowest in the control plots (p < 0.05). Annual NO emissions ranged from 0.01 to 0.18, 0.03 to 0.65, −0.16 to 1.8 and −0.01 to 0.66 kg N ha−1 in CT, F, MF and M plots, respectively. There was no significant difference in annual NO emissions between the grassland and cornfield.
During winter and thawing periods NO fluxes were generally low and varied widely. Winter and thawing period NO fluxes in the CT plot contributed more to annual emissions compared to MF and F plots. The highest contributions of winter and thawing seasons to annual NO emissions were 55 and 32%, respectively, in the CT plot (Tables S1 and S2).
3.4. N2O-N/NO-N ratio
The ratio of N2O-N to NO-N (N2O-N/NO-N) is used an indicator of the dominant mechanism of N2O production in the soil. If the ratio is less than 1, nitrification is the mechanism of N2O production; if it is greater that 100 denitrification is the main mechanism (Bouwman Citation1990). In OG, 2.4, 79.2 and 18.4% of the N2O-N/NO-N ratio values were less than 1, between 1 and 100, and greater than 100, respectively. In cornfield and NG, less than 1% (0.7 and 0.9%, respectively) of the N2O-N/NO-N values were less than 1. About 69.6 and 64.5% of the N2O-N/NO-N values were between 1 and 100, and 29.7 and 34.5% were greater than 100 in cornfield and NG, respectively. Nitrous oxide flux increased with increasing N2O-N/NO-N values in all plots and land uses combined.
3.5. Factors controlling N2O and NO emissions
Daily N2O fluxes were influenced by soil temperature, precipitation, soil pH, moisture content and N supply. In OG, the instantaneous N2O fluxes had significant positive correlations with soil temperature (p < 0.001), NO3− concentration (p < 0.01) and NH4+ concentration (p < 0.001), and non-significant (ns) negative correlations with WFPS, soil pH and WEOC. In the cornfield, correlations were positive with NO3− (ns) and soil temperature (p < 0.001), and negative but non-significant with NH4+, WFPS, pH and WEOC. In NG, N2O correlated positively with soil temperature, NH4+ concentration and NO3− (p < 0.01), and negatively with soil pH (p < 0.05), WEOC (p < 0.05) and WFPS (ns).
Annual N2O emissions, in all three land uses, increased with total N input and surplus N in the soil (p < 0.05). Annual precipitation had a significant positive linear correlation with annual N2O emission in cornfield, and an exponential relationship in NG (). Soil pH showed a negative correlation with annual N2O emission, but it was significant only in cornfield (). However, the ratio of surplus N emitted as N2O (N2O-N/surplus N) had a stronger negative correlation with soil pH in all three land uses ().
Figure 4. Relationship between annual nitrous oxide (N2O) emission and annual precipitation in (a) old grassland, (b) cornfield and (c) new grassland. CT is control plot; F is chemical fertilizer plot; MF is combined chemical fertilizer and manure plot; M is manure only plot.
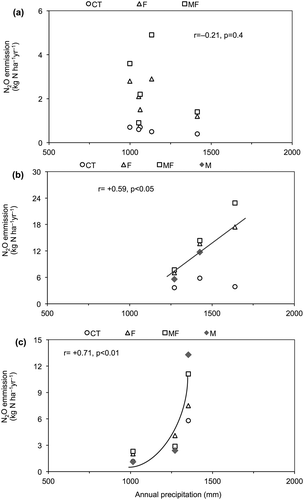
Figure 5. Relationship between annual nitrous oxide (N2O) emission and soil pH and ratio of annual nitrogen emitted as N2O (N2O-N) to surplus nitrogen and soil pH in (a, b) old grassland, (c, d) cornfield, and (e, f) new grassland. CT is control plot; F is chemical fertilizer plot; MF is combined chemical fertilizer and manure plot; M is manure only plot.
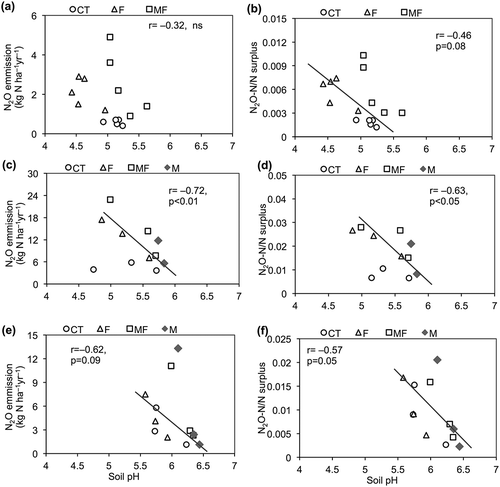
The ratio of WEOC to soil nitrate (WEOC/NO3−) was the major driver of changing N2O emission as the land use changed (). The WEOC/NO3− ratio explained 78% of changes in annual N2O emission as land use changed in the control plot ().
Table 6. Multiple and single linear regression models accounting for change in annual nitrous oxide (N2O) emission with changing land use in the unfertilized control plots (CT), chemical fertilizer plot (F) and manure and chemical fertilizer plot (MF).
Figure 6. Relationship between annual nitrous oxide (N2O) emission and the ratio of mean water extractable organic carbon to mean soil nitrate (WEOC/NO3−). Data in white symbols are in old grassland, gray symbols in cornfield and black symbols in new grassland. CT is control plot; F is chemical fertilizer plot; MF is combined chemical fertilizer and manure plot; M is manure only plot.
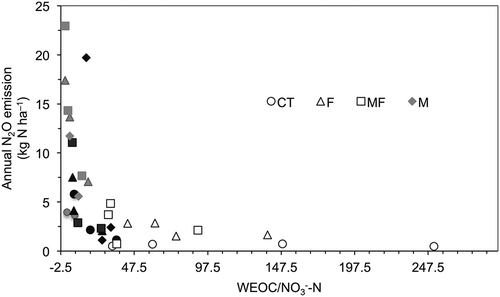
Nitric oxide fluxes only showed significant correlations with WFPS (negative) in all treatments, and with soil NO3− and NH4+ (positive) in F and MF plots (p < 0.05). N addition was the single most important factor affecting annual NO emissions.
3.6. Heterotrophic soil respiration (RH), mineralized N, plant N uptake and surplus N
Total RH and total mineralized N were higher in manure-amended plots than in F and CT plots, and higher in cornfield than in OG and NG (p < 0.05; Table S3). Plant N uptake in OG and cornfield was not statistically different, but was higher than in NG (p < 0.01) (Table S4). Surplus soil N in cornfield was higher than in both OG and NG (p < 0.01), and higher in NG than OG (p < 0.05; Table S4). Chemical fertilization significantly increased plant N uptake (p < 0.05).
4. Discussion
4.1. Temporal variation in N2O and NO emissions
The N2O fluxes in this study were highly variable, and peak emissions occurred either after N addition or after high rainfall. In OG and the last 2 years of NG, all peak emissions occurred after N addition, with less influence of rainfall. In cornfield and the first year of NG rainfall had a larger impact on peak emissions than N addition did. These differences in the response of peak N2O fluxes among the three land uses were presumably due to differences in soil mineral N content, aeration and redox conditions. High NO3− content in cornfield and in the first year of NG provided a substrate for denitrifiers, while high precipitation created favorable conditions for denitrification. Occurrence of high rainfall when the WEOC/NO3− ratio was high, in OG and in the last 2 years of NG, would have favored complete denitrification to N2 gas and hence fewer N2O fluxes (Burchill et al. Citation2014; Iqbal et al. Citation2014).
In OG, N2O peaks following N application were higher and lasted longer after the second fertilization in summer compared to the first application in spring in the F plot. In MF and M plots, the peaks were higher in spring when both manure and chemical fertilizer were applied. Manure applications enhance microbial activity, which reduces soil O2 levels, creating conditions that favor N2O emissions (Collins et al. Citation2011; Zhang et al. Citation2014), which could explain the observed differences between F and manure plots.
The timing of peak NO fluxes were similar to those of N2O despite being much smaller in magnitude (), which should be expected as both gases are mainly the products of nitrification and denitrification processes and are driven by similar abiotic factors (Davidson et al. Citation1993; Skiba et al. Citation1997; Yan et al. Citation2013; Medinets et al. Citation2015). Smaller peak NO fluxes relative to N2O are in agreement with results reported by Wang et al. (Citation2011) and Yan et al. (Citation2013). In this study, the peak N2O-N fluxes were up to 200 times higher than the peak NO-N fluxes, which is significantly higher than those reported by Wang et al. (Citation2011). However, the higher peak N2O than NO fluxes found in this study are contrary to results from other studies (Smith et al. Citation1997; Akiyama et al. Citation2000; Akiyama and Tsuruta Citation2002) which reported up to 20 times more NO-N than N2O-N. This contradiction among different studies could be due to differences in soil moisture and fertilizer types (Smith et al. Citation1997; Akiyama et al. Citation2000). When WFPS is greater than 60%, denitrification, which produces more N2O than NO, is predominant (Davidson et al. Citation1993; Smith et al. Citation1997) and diffusion of NO is limited, which allows further consumption of NO by denitrification (Skiba et al. Citation1997; Smith et al. Citation1997). The average WFPS value in this study was above 70%.
Few studies have reported long-term data of N2O and NO emissions. There was up to a 10-fold difference in inter-annual N2O emissions within each land use and treatment in this study. Differences in annual NO emissions were as great as 6 times. This high variation in annual emissions emphasizes the need for long-term studies to reduce uncertainties associated with chamber flux measurements for individual sites.
4.2. Influence of N application on N2O and NO emissions
The N2O emissions in fertilizer and manure-amended plots were 3–4, 2–5 and 1.4–2 times higher than in the control treatment in OG, cornfield and NG, respectively ( and ). These results are similar to those of Mosier et al. (Citation1991) who reported an increase of 2–3 times in N2O emission due to fertilization in native grassland and wheat (Triticum aestivum L.) prairies in the USA. Several studies have reported increased N2O emission with manure and fertilizer applications (Mu et al. Citation2006; Alluvione et al. Citation2010; Collins et al. Citation2011; Ryals and Silver Citation2012; Zhang et al. Citation2014). Manure applications enhance microbial activity, which reduces soil O2 levels, creating conditions that favor N2O emissions (Collins et al. Citation2011; Zhang et al. Citation2014).
Our results indicate that soil organic matter mineralization and plant N uptake are important parameters affecting N2O-N emissions as shown by the significant positive relationship between N2O emissions and surplus N and total N input. Therefore, soil organic matter decomposition and plant type should be included when evaluating the emission factors of different soils.
Chemical fertilizer and long-term manure application had a significant influence on soil properties such as pH, mineral N content and organic carbon content (). Soil pH was significantly decreased by chemical fertilizer application and increased by long-term manure application. Manure application increased and maintained soil pH probably due to the high pH of the manure (manure pH was around 7). The second reason is that manure increases the buffering capacity of soils due to the presence of carboxyl and phenolic hydroxyl groups in the manure (Whalen et al. Citation2000). The negative relationship between pH and N2O emission () suggests that under similar conditions, long-term manure could have benefits of reducing N2O emissions indirectly by increasing soil pH, while the opposite is true for chemical fertilizer.
Nitric oxide fluxes were stimulated just after fertilization, similar to many published reports (Skiba et al. Citation1997; Akiyama and Tsuruta Citation2002; Bouwman et al. Citation2002a; Cui et al. Citation2012). Although annual NO emissions were higher in inorganic N fertilized plots, regression analysis showed a non-significant increase in annual NO emissions with increasing N input, which disagrees with other studies (Cui et al. Citation2012; Yan et al. Citation2013) that reported a significant linear relation between annual NO emissions and fertilizer N input. One possible explanation for this seemingly non-significant response of annual NO emissions N input is that high moisture content in our site limited the diffusion of NO to the surface (Firestone and Davidson Citation1989; Skiba et al. Citation1997; Medinets et al. Citation2015) which in turn increases the likelihood of NO consumption in the soil by denitrification (Aneja et al. Citation1996; Akiyama and Tsuruta Citation2003; Yao et al. Citation2010; Pilegaard Citation2013).
4.3. Soil and environmental factors controlling N2O emissions
As expected, total N input and surplus N, NO3− and NH4+ concentrations in the soil were important controlling factors. In cornfield and NG in 2013, the highest N2O fluxes were recorded following rainfall higher than 40 mm in 1 d. Other factors such as tillage (Chapin et al. Citation2011; Li et al. Citation2015), oxygen availability (Firestone and Davidson Citation1989; Venterea et al. Citation2005; Iqbal et al. Citation2014) and precipitation (Koga et al. Citation2004) are more important when inorganic N is not limiting in the soil, and hence were very important factors in cornfield. In this study, the higher soil mineral N content (both NO3− and NH4+) in cornfield and NG, even in the control treatment without any N addition, could have been due to enhanced mineralization resulting from tillage (Shimizu et al. Citation2013). The higher heterotrophic respiration values observed in cornfield and NG compared to OG support this claim (Table S3).
Effects of soil moisture and rainfall on N2O production have been reported by many studies (Mosier et al. Citation1991; Choudhary et al. Citation2001; Sehy et al. Citation2003; Alluvione et al. Citation2010). High N2O fluxes associated with high soil moisture were likely to have come primarily from denitrification (Sehy et al. Citation2003; Alluvione et al. Citation2010; Shimizu et al. Citation2013). Precipitation enhanced N2O emission due to stimulation of substrate diffusivity and microbial activity with increased soil moisture content (Kusa et al. Citation2002; Bateman and Baggs Citation2005), reduced oxygen diffusivity (Saggar et al. Citation2013) and the resulting increase in denitrification (Saggar et al. Citation2013; Li et al. Citation2015). A negative but non-significant correlation between annual N2O emissions and precipitation in OG was found. In 2009, when the highest rainfall was recorded in OG, N2O emissions were very low. This could be due to lower total N input () and surplus N (Table S4), and also lower NO3− and NH4+ concentrations in OG (). Another reason could be that high rainfall in grassland, given the limited drainage in our site and high available carbon relative to NO3− (), might have promoted complete denitrification (Burchill et al. Citation2014; Iqbal et al. Citation2014).
The amount of surplus N emitted as N2O (N2O-N/surplus N) had a much stronger negative correlation with soil pH than just N2O-N and pH in all three land uses (). These results suggest that it is the excess (surplus) N in the soil that is much more influenced by soil conditions and transformed to N2O. This is supported by a significant positive correlation between N2O emissions and surplus N. A negative relationship between N2O and soil pH has been reported by a number of studies (Clough et al. Citation2004; Pan et al. Citation2012). Increased activity of N2O reductase enzyme relative to activities of NO3− and NO2− reductase enzymes at high pH may be the main reason for the low N2O at high pH (Pan et al. Citation2012). However, this result is contrary to the increased cumulative N2O production with increasing pH in grassland and forest soils in Canada reported by Cheng et al. (Citation2013).
Multiple regression analysis showed that soil moisture and NH4+ concentration were the key factors regulating NO fluxes, although NO fluxes showed strong positive correlation with temperature and NO3− concentration as single factors. The negative correlation of NO with WFPS is consistent with the reported impediment of the diffusion of NO at high moisture content, thereby allowing NO consumption (Davidson et al. Citation1993; Medinets et al. Citation2015). The fact that NH4+ showed a stronger controlling effect on NO than NO3− agrees with reports that nitrification was the major source of the NO fluxes (Cui et al. Citation2012). However, Skiba et al. (Citation1997) reported that denitrification produces more NO than nitrification but net release of NO from denitrification is lower due to impediment of NO diffusivity and NO consumption by denitrifiers.
4.4. Importance of winter and thawing periods N2O and NO emissions
Winter emissions contributed as much as 35 and 55% in N2O and NO emissions, respectively ( and S3). The contribution of winter N2O emissions was higher when manure was applied in autumn in cornfield compared to spring in grassland. Winter sampling was done twice or once a month and therefore these values might have been underestimated. However, this study clearly shows that winter emissions contribute a significant amount to annual emissions, and this calls for more intensive sampling and inclusion of winter emissions in annual budgets.
The 2-month-long thawing period (March to early May) contributed as much as 60% to annual emissions in some years ( and S2). In the control plots, thawing period emissions were even more important compared to the other plots. The N2O emissions increased following soil melting and as soil temperatures became warmer. The high fluxes in this period could be due to high accumulation of N2O through denitrification during the freezing period, and the physical release as the snow melts (Burchill et al. Citation2014) and low N2O reduction rate during thawing (Sehy et al. Citation2003; Katayanagi and Hatano Citation2012). Peaks of N2O emissions in the thawing period may also be due to enhanced mineralization of easily decomposable organic substrates by increased microbial activity (Wu et al. Citation2010).
4.5. Effect of land-use type on N2O and NO emissions
In this study, the average annual N2O emissions in grassland (OG and NG) ranged from 0.4 to 4.9 kg N ha−1 yr−1, except in 2013 in NG when emissions ranged from 5.8 to13.3 9 kg N ha−1 yr−1. The high N2O emissions in NG in 2013 may have been due to plowing twice, in May and September, and reseeding of the grass. Higher precipitation in 2013 just after plowing and seeding in spring may have further stimulated N2O emissions. The average annual N2O emissions in cornfield ranged from 3.6 to 22.9 kg N ha−1 yr−1, and they were significantly higher than values reported by Alluvione et al. (Citation2010) in Italy of 3.9 to 8.7 and 3.9 kg N2O–N ha−1 and those of Choudhary et al. (Citation2001) who found mean values of 2.3 to 3.4 kg N2O–N ha−1 yr−1 in a silt clay loam soil. Higher N input and precipitation in this study could explain the observed differences in the N2O emissions.
Higher N2O emissions in cornfield compared to OG and NG were probably due to higher soil NO3− concentrations (), higher heterotrophic soil respiration and consequently higher mineralized N and higher surplus N. Furthermore, the perennial plants, in grassland, were always in the field and hence capable of taking up available soil N, especially in spring. In the cornfield, on the other hand, there was no plant uptake of available N in early spring and autumn, yet manure was applied in autumn and chemical fertilizer at the time of seeding. This lack of N uptake by plants in some periods, and hence the lack of synchronization of plant uptake and soil N availability in some periods, combined with higher precipitation, could have led to overall higher annual emissions in cornfield (FAO and IFA Citation2001; UNEP Citation2013; Iqbal et al. Citation2014). Tillage activities which were conducted every year in the cornfield further influenced heterotrophic soil respiration and N mineralization, and hence N2O emissions. Increasing N2O emissions due to tillage activities has been reported by several studies (Ruan and Philip Robertson Citation2013; Palm et al. Citation2014; Yonemura et al. Citation2014). The cornfield emissions were not significantly different from NG emissions of 2013 when tillage was conducted.
The average N2O emissions over the whole study period were higher in NG than OG ( and ). This could be attributed to higher NO3− concentration (), lower plant N uptake and, as a result, higher surplus N in NG compared to OG (Table S4). This means more applied N in OG was taken up by the plant, hence acting as a sink for N (Velthof et al. Citation2010; Necpa ́lova ́ et al. Citation2013; Iqbal et al. Citation2014). In this study, tillage activities and very high precipitation in 2013 in NG may have played a part in the observed higher emissions. In 2014, the emissions were much lower in NG, and by 2015 (3 years after establishment of new conversion) N2O emissions in NG were not significantly different from those in OG. Our results suggest that within 3 years after conversion from annual cropland to managed grassland, significant reductions in N2O emissions could be achieved.
Soil NO3− concentrations in the cornfield and 2013 in NG were significantly higher than in OG, while the WEOC did not differ significantly among the land-use types (). The ratio of WEOC to NO3−N was highest in OG and lowest in cornfield. High abundance of NO3− relative to labile organic carbon favors N2O release over N2 (Firestone and Davidson Citation1989; Chapin et al. Citation2011; Iqbal et al. Citation2014). This is because high NO3− (electron acceptor) will lead to depletion of the relatively less abundant electron donor (carbon) (Iqbal et al. Citation2014), resulting in incomplete denitrification and accumulating higher amounts of N2O in the soil. Lower NO3−, on the other hand, may stimulate the reduction of N2O to N2 (Firestone and Davidson Citation1989; Iqbal et al. Citation2014). Our results as shown in are in agreement with this interpretation.
Our study shows no significant differences in NO emissions among the three land uses. This finding is supported by Van Lent et al. (Citation2015). Skiba et al. (Citation1997) reviewed several papers and found conflicting reports of land-use effects on NO emissions. However, other studies have reported lower NO emissions in grassland compared to cornfield and attributed this to greater N-use efficiency due to longer growing seasons in grasslands (Bouwman et al. Citation2002b).
5. Conclusion
Annual N2O emissions in cornfield were 6–7 times higher than in OG and 1.5–3 times higher than in NG, and NG had 2–5 times higher N2O emissions than OG. Higher cornfield emissions compared to grassland, and higher emissions in NG compared to OG, were due to higher available soil mineral N relative to labile soil organic carbon which could have led to incomplete reduction of NO3− to N2, producing more N2O in the process. Lack of synchronization of N availability in the soil and plant N uptake may have further led to the high emissions in the cornfield as well as in the first year of NG. Within the first year of converting grassland to cornfield, N2O emissions increased by more than 500% and remained high 3 years later, while after converting from cornfield to new grassland emissions significantly declined within 3 years. Peaks of N2O flux following fertilization were heavily influenced by land use and interacted strongly with rainfall. Nitric oxide emissions were more influenced by nitrogen addition than soil and weather variables.
Winter and thawing period N2O and NO emissions contributed significantly to annual emissions, highlighting the need for a high frequency of measurements in these periods. There was up to a 10-fold difference in inter-annual N2O emissions within each land use and treatment in this study. Differences in annual NO emissions were as high as 6 times. This high variation in annual emissions emphasizes the need for long-term studies to reduce uncertainties associated with chamber flux measurements for individual sites.
Supplemetary information
Download MS Word (55.1 KB)Acknowledgments
This study was partly supported by a research grant provided by the projects ‘Establishment of good practices to mitigate greenhouse gas emissions from Japanese grasslands’ (FY 2004-2009) organized by the Japan Grassland Agriculture and Forage Seed Association (GAFSA), and ‘Development of Mitigation Technologies to Climate Change in the Agriculture Sector (FY 2010–2014)’ run by the Ministry of Agriculture, Forestry and Fisheries of Japan. The authors thank the staff and management of the Hokkaido University Shizunai Livestock experimental farm for their assistance in field management activities.
Supplemental data
Supplemental data for this article can be accessed here.
Additional information
Funding
References
- Akiyama H, Tsuruta H 2002: Effect of chemical fertilizer form on N2O, NO and NO2 fluxes from Andisol field. Nutr. Cycl. Agroecosyst., 63, 219–230. doi:10.1023/A:1021102925159
- Akiyama H, Tsuruta H 2003: Nitrous oxide, nitric oxide, and nitrogen dioxide fluxes from soils after manure and urea application. J. Environ. Qual., 32, 423–431. doi:10.2134/jeq2003.4230
- Akiyama H, Tsuruta H, Watanabe T 2000: N2O and NO emissions from soils after the application of different chemical fertilizers. Chemosphere Global Change Sci., 2, 313–320. doi:10.1016/S1465-9972(00)00010-6
- Alluvione F, Bertora C, Zavattaro L, Grignani C 2010: Nitrous oxide and carbon dioxide emissions following green manure and compost fertilization in corn. Soil Sci. Soc. Am. J., 74, 384–395. doi:10.2136/sssaj2009.0092
- Aneja VP, Robarge WP, Sullivan LJ, Moore TC, Pierce TE, Geron C, Gay B 1996: Seasonal variations of nitric oxide flux from agricultural soils in the Southeast United States. Tellus, 48, 626–640. doi:10.3402/tellusb.v48i5.15936
- Bateman EJ, Baggs EM 2005: Contributions of nitrification and denitrification to N2O emissions from soils at different water-filled pore space, Biol. Fertil. Soils, 41, 379–388. doi:10.1007/s00374-005-0858-3
- Bouwman AF 1990: Nitric oxide and nitrogen dioxide. In Soils and the Greenhouse Gas Effect, Ed. Bouwman AF, pp. 120–124. Chichester, Great Britain: John Wiley & Sons.
- Bouwman AF, Boumans LJ, Batjes N 2002a: Emissions of N2O and NO from fertilized fields: summary of available measurement data, Global Biogeochem. Cycles, 16(4), 6-1–6-13. doi:10.1029/2001GB001811
- Bouwman AF, Boumans LJM, Batjes NH 2002b: Modelling global annual N2O and NO emissions from fertilized fields, Global Biogeochem. Cycles, 16(4), 28–29. doi:10.1029/2001GB001812
- Burchill W, Li D, Lanigan GJ, Williams M, Humphreys J 2014: Interannual variation in nitrous oxide emissions from perennial ryegrass /white clover grassland used for dairy production, Glob. Chang. Biol., 20, 3137–3146. doi:10.1111/gcb.12595
- Chapin SF, Matson PA, Vitousek PM 2011: Principles of Terrestrial Ecosystem Ecology, 2nd ed. New York, NY: Springer. ISBN 978-1-4419-9503-2.
- Cheng Y, Cai Z, Chang SX, Wang J, Zhang J 2013: Effects of soil pH and salt on N2O production in adjacent forest and grassland soils in central Alberta, Can. J. Soil Sci., 13, 863–868.
- Choudhary MA, Akramkhanov A, Saggar S 2001: Nitrous oxide emissions in soils cropped with maize under long-term tillage and under permanent pasture in New Zealand. Soil Tillage Res., 62, 61–71. doi:10.1016/S0167-1987(01)00208-2
- Ciais P, Sabine C, Bala Get al. 2013: Carbon and other biogeochemical cycles. In Climate Change 2013: the Physical Science Basis. Contribution of Working Group I to the Fifth Assessment Report of the Intergovernmental Panel on Climate Change, Eds. Stocker TF, Qin D, Plattner GK, Tignor M, Allen SK, Boschung J, Nauel A, Xia Y, Bex V, Midgley PM. Cambridge: Cambridge University Press.
- Clough TJ, Kelliher FM, Sherlock RR, Ford CD 2004: Lime and soil moisture effects on nitrous oxide emissions from a urine patch. Soil Sci. Soc. Am. J., 68, 1600–1609. doi:10.2136/sssaj2004.1600
- Collins HP, Alva AK, Streubel JD, Fransen S, Frear C, Chen S, Kruger C, Granatstein D 2011: Greenhouse gas emissions from an irrigated silt loam soil amended with anaerobically digested dairy manure. Soil Sci. Soc. Am. J., 75, 2206–2216. doi:10.2136/sssaj2010.0360
- Crutzen PJ 1979: The role of NO and NO2 in the chemistry of the troposphere and stratosphere. Ann. Rev. Earth Planet. Sci., 7, 443–472. doi:10.1146/annurev.ea.07.050179.002303
- Cui F, Yan G, Zhou Z, Zheng X, Deng J 2012: Annual emissions of nitrous oxide and nitric oxide from a wheat–maize cropping system on a silt loam calcareous soil in the North China Plain. Soil Biol. Biochem., 48, 10–19. doi:10.1016/j.soilbio.2012.01.007
- Davidson AEA, Matson PA, Vitousek PM, Riley R, Dunkin K, Garcia-Mendez G, Maass JM 1993: Processes regulating soil emissions of NO and N2O in a seasonally dry tropical forest. Ecology, 74, 130–139. doi:10.2307/1939508
- Davies MG, Smith KA, Vinten AJA 2001: The mineralization and fate of nitrogen following ploughing of grass and grass-clover swards. Biol. Fertil. Soils, 33, 423–434. doi:10.1007/s003740100348
- De Klein CAM, Harvey M, Ed. 2015: Nitrous Oxide Chamber Methodology Guidelines. Wellington: Ministry of Primary Industies.
- De Klein CAM, Barton L, Sherlock RR, Li Z, Littlejohn RP 2003: Estimating a nitrous oxide emission factor for animal urine from some New Zealand pastoral soils. Austr. J. Soil Res., 41, 381–399. doi:10.1071/SR02128
- Eickenscheidt N, Brumme R 2013: Regulation of N2O and NOx emission patterns in six acid temperate beech forest soils by soil gas diffusivity, N turnover, and atmospheric NOx concentrations. Plant Soil, 369, 515–529. doi:10.1007/s11104-013-1602-7
- FAO 2003: World Agriculture: towards 2015/2030. London: Earthscan Publications.
- FAO and IFA 2001: Global Estimates of Gaseous Emissions of NH3, NO and N2O from Agricultural Land. Rome: Viale delle Terme di Caracalla.
- Firestone MK, Davidson EA 1989: Microbiological basis of NO and N2O production and consumption in soil. In Exchange of Trace Gases between Terrestrial Ecosystems and the Atmosphere, Eds. Andreae MO, Schimel DS. New York, NY: John Wiley & Sons.
- IPCC 2007: Climate Change 2007: the Physical Science Basis. Contribution of Working Group I to the Fourth Assessment Report of the Intergovernmental Panel on Climate Change. Cambridge, UK: Cambridge University Press.
- Iqbal J, Parkin TB, Helmers MJ, Zhou X, Castellano MJ 2014: Denitrification and nitrous oxide emissions in annual croplands, perennial grass buffers, and restored perennial grasslands, Soil Sci. Soc. Am. J. doi:10.2136/sssaj2014.05.0221
- IUSS Working Group WRB 2006: World Reference Base for Soil Resources. World Soil Resources Reports 103. Rome: FAO.
- Jin T, Shimizu M, Marutani S, Desyatkin AR, Iizuka N, Hata H, Hatano R 2010: Effect of chemical fertilizer and manure application on N2O emission from reed canary grassland in Hokkaido, Japan. Soil Sci. Plant Nutr., 56, 53–65. doi:10.1111/j.1747-0765.2010.00447.x
- Katayanagi N, Hatano R 2012: N2O emissions during the freezing and thawing periods from six fields in a livestock farm, southern Hokkaido, Japan. Soil Sci. Plant Nutr., 58, 261–271. doi:10.1080/00380768.2012.670810
- Koga N, Tsuruta H, Sawamoto T, Nishimura S, Yagi K 2004: N2O emission and CH4 uptake in arable fields managed under conventional and reduced tillage cropping systems in northern Japan. Global Biogeochem. Cycles, 18, 1–11. doi:10.1029/2004GB002260
- Kurganova I, Teepe R, Loftfield N 2007: Influence of freeze-thaw events on carbon dioxide emission from soils at different moisture and land use, Carbon Balance Manag., 2, 2. doi:10.1186/1750-0680-2-2
- Kusa K, Sawamoto T, Hatano R 2002: Nitrous oxide emissions for 6 years from a gray lowland soil cultivated with onions in Hokkaido, Japan, Nutr. Cycl. Agroecosys., 63, 239–247. doi:10.1023/A:1021167202601
- Li M, Shimizu M, Hatano R 2015: Evaluation of N2O and CO2 hot moments in managed grassland and cornfield, southern Hokkaido, Japan, Catena, 133, 1–13. doi:10.1016/j.catena.2015.04.014
- Limin A, Shimizu M, Mano M, Ono K, Miyata A, Wada H, Nozaki H, Hatano R 2015: Manure application has an effect on the carbon budget of a managed grassland in southern Hokkaido, Japan, Soil Sci. Plant Nutr., 61, 857–872. doi:10.1080/00380768.2015.1051930
- Linn DM, Doran JW 1984: Effect of water–filled pore–space on carbon dioxide and nitrous oxide production in tilled and non-tilled soils. Soil Sci. Soc. Am. J., 48, 1267–1272. doi:10.2136/sssaj1984.03615995004800060013x
- Logan JA 1983: Nitrogen oxides in the troposphere: global and regional budgets, J Geophy. Res., 88(C15), 10785. doi:10.1029/JC088iC15p10785
- Medinets S, Skiba U, Rennenberg H, Butterbach-Bahl K 2015: A review of soil NO transformation: associated processes and possible physiological significance on organisms, Soil Biol. Biochem., 80, 92–117. doi:10.1016/j.soilbio.2014.09.025
- Mosier A, Kroeze C 2000: Potential impact on the global atmospheric N2O budget of the increased nitrogen input required to meet future global food demands. Chemosphere Global Change Sci., 2, 465–473. doi:10.1016/S1465-9972(00)00039-8
- Mosier A, Schimel D, Valentine D, Bronson K, Parton W 1991: Methane and nitrous oxide fluxes in native, fertilized and cultivated grasslands. Nature, 350, 330–332. doi:10.1038/350330a0
- Mu Z, Kimura SD, Hatano R 2006: Estimation of global warming potential from upland cropping systems in central Hokkaido, Japan. Soil Sci. Plant Nutr., 52, 371–377. doi:10.1111/j.1747-0765.2006.00046.x
- Necpa ́lova ́ M, Casey I, Humphreys J 2013: Effect of ploughing and reseeding of permanent grassland on soil N, N leaching and nitrous oxide emissions from a clay-loam soil. Nutr. Cycl. Agroecosys., 95, 305–317. doi:10.1007/s10705-013-9564-y
- Oenema O, Wrage N, Velthof GL, Groenigen JW, Dolfing J, Kuikman PJ 2005: Trends in global nitrous oxide emissions from animal production systems, Nutr. Cycl. Agroecosyst., 72, 51–65. doi:10.1007/s10705-004-7354-2
- Owen JJ, Parton WJ, Silver WL 2015: Long-term impacts of manure amendments on carbon and greenhouse gas dynamics of rangelands, Glob. Chang. Biol., 21, 4533–4547. doi:10.1111/gcb.13044
- Palm C, Blanco-Canqui H, DeClerck F, Gatere L, Grace P 2014: Conservation agriculture and ecosystem services: an overview, Agric. Ecosyst. Environ., 187, 87–105. doi:10.1016/j.agee.2013.10.010
- Pan Y, Ye L, Ni B, Yuan Z 2012: Effect of pH on N2O reduction and accumulation during denitrification by methanol utilizing denitrifiers. Water Res., 46, 4832–4840. doi:10.1016/j.watres.2012.06.003
- Pilegaard K 2013: Processes regulating nitric oxide emissions from soils. Philos. Trans. R. Soc. Lond., B, Biol. Sci., 368, 20130126. doi:10.1098/rstb.2013.0126
- Ruan L, Philip Robertson G 2013: Initial nitrous oxide, carbon dioxide, and methane costs of converting conservation reserve program grassland to row crops under no-till vs. conventional tillage, Glob. Chang. Biol., 19, 2478–2489. doi:10.1111/gcb.12216
- Ryals R, Silver WL 2012: Effects of organic matter amendments on net primary productivity and greenhouse gas emissions in annual grasslands, Ecol. Appl., 23, 120821140338006. doi:10.1890/12-0620.1
- Saggar S, Jha N, Deslippe J, Bolan NS, Luo J, Giltrap DL, Kim D-G, Zaman M, Tillman RW 2013: Denitrification and N2O: N2 production in temperate grasslands: processes, measurements, modelling and mitigating negative impacts, Sci. Total Environ., 465, 173–195. doi:10.1016/j.scitotenv.2012.11.050
- Sehy U, Ruser R, Munch JC 2003: Nitrous oxide fluxes from maize fields: relationship to yield, site-specific fertilization, and soil conditions. Agric. Ecosyst. Environ., 99, 97–111. doi:10.1016/S0167-8809(03)00139-7
- Shepherd MA, Hatch DJ, Jarvis SC, Bhogal A 2006: Nitrate leaching from reseeded pasture. Soil Use Manag, 17, 97–105. doi:10.1111/j.1475-2743.2001.tb00014.x
- Shimizu M, Hatano R, Arita Tet al. 2013: The effect of fertilizer and manure application on CH4 and N2O emissions from managed grasslands in Japan, Soil Sci. Plant Nutr., 59(1), 69–86. doi:10.1080/00380768.2012.733926
- Shimizu M, Limin A, Desyatkin AR, Jin T, Mano M, Ono K, Miyata A, Hata H, Hatano R 2015: Effect of manure application on seasonal carbon fluxes in a temperate managed grassland in Southern Hokkaido, Japan. Catena, 133, 474–485. doi:10.1016/j.catena.2015.05.011
- Shimizu M, Marutani S, Desyatkin AR, Jin T, Hata H, Hatano R 2009: The effect of manure application on carbon dynamics and budgets in a managed grassland of Southern Hokkaido, Japan, Agric. Ecosyst. Environ., 130, 31–40. doi:10.1016/j.agee.2008.11.013
- Shimizu M, Marutani S, Desyatkin AR, Jin T, Nakano K, Hata H, Hatano R 2010: Nitrous oxide emissions and nitrogen cycling in managed grassland in Southern Hokkaido, Japan. Soil Sci. Plant Nutr., 56, 676–688. doi:10.1111/j.1747-0765.2010.00496.x
- Skiba U, Fowler D, Smith K 1997: Nitric oxide emissions from agricultural soils in temperate and tropical climates: sources, controls and mitigation options. Nutr. Cycl. Agroecosyst., 48, 139–153. doi:10.1023/A:1009734514983
- Smith K, McTaggart IP, Tsuruta H 1997: Emissions of N2O and NO associated with nitrogen fertilization in intensive agriculture, and the potential for mitigation. Soil Use Manag, 13, 296–304. doi:10.1111/j.1475-2743.1997.tb00601.x
- Smith KA, Conen F 2004: Impacts of land management on fluxes of trace greenhouse gases, Soil Use Manag, 20, 255–263. doi:10.1079/SUM2004238
- Smith P, Martino D, Cai Zet al. 2007: Agriculture. In Climate Change 2007: mitigation. Contribution of Working Group III to the Fourth Assessment Report of the Intergovernmental Panel on Climate Change, Eds. Metz B, Davidson OR, Bosch PR, Dave R, Meyer LA. Cambridge, UK: Cambridge University Press.
- Stolk PC, Jacobs CMJ, Moors EJ, Hensen A, Velthof GL, Kabat P 2009: Significant non-linearity in nitrous oxide chamber data and its effect on calculated annual emissions. Biogeosciences Discuss., 6, 115–141. doi:10.5194/bgd-6-115-2009
- Toma Y, Hatano R 2007: Effect of crop residue C:N ratio on N2O emissions from Gray Lowland soil in Mikasa, Hokkaido, Japan. Soil Sci. Plant Nutr., 53, 198–205. doi:10.1111/j.1747-0765.2007.00125.x
- Tubiello FN, Salvatore M, Rossi S, Ferrara A, Fitton N, Smith P 2013: The FAOSTAT database of greenhouse gas emissions from agriculture, Environ. Res. Lett., 8, 015009. doi:10.1088/1748-9326/8/1/015009
- UNEP 2013: Drawing down N2O to Protect Climate and the Ozone Layer. A UNEP Synthesis Report. Nairobi: United Nations Environment Programme (UNEP).
- United States Environmental Protection Agency (US EPA) 2006: Global Anthropogenic Non-CO2 Greenhouse Gas Emissions: 1990-2020. Washington D.C.
- Ussiri DAN, Lal R 2009: Long-term tillage effects on soil carbon storage and carbon dioxide emissions in continuous corn cropping system from an alfisol in Ohio. Soil Tillage Res., 104, 39–47. doi:10.1016/j.still.2008.11.008
- Ussiri DAN, Lal R, Jarecki MK 2009: Nitrous oxide and methane emissions from long-term tillage under a continuous corn cropping system in Ohio. Soil Tillage Res., 104, 247–255. doi:10.1016/j.still.2009.03.001
- Van Lent J, Hergoualc’h K, Verchot LV 2015: Reviews and syntheses: soil N2O and NO emissions from land use and land-use change in the tropics and subtropics: a meta-analysis. Biogeosciences, 12, 7299–7313. doi:10.5194/bg-12-7299-2015
- Vanderzaag AC, Jayasundara S, Wagner-Riddle C 2011: Strategies to mitigate nitrous oxide emissions from land applied manure. Anim Feed Sci. Technol., 166–167, 464–479. doi:10.1016/j.anifeedsci.2011.04.034
- Velthof G, Hoving I, Dolfing J, Smit A, Kuikman P, Oenema O 2010: Method and timing of grassland renovation affects herbage yield, nitrate leaching, and nitrous oxide emission in intensively managed grasslands. Nutr. Cycl. Agroecosyst., 86, 401–412. doi:10.1007/s10705-009-9302-7
- Venterea RT, Burger M, Spokas K 2005: Nitrogen oxide and methane emissions under varying tillage and fertilizer management. J. Environ. Qual., 34, 1467–1477. doi:10.2134/jeq2005.0018
- Vitousek PM, Aber JD, Howarth RW, Likens GE, Matson PA, Schinder DW, Schlesinger WH, Tilman DG 1997: Human alteration of the global nitrogen cycle: sources and consequences, Ecological Appl., 7, 737–750.
- Wang R, Willibald G, Feng Q, Zheng X, Liao T, Brüggemann N, Butterbach-Bahl K 2011: Measurement of N2, N2O, NO, and CO2 emissions from soil with the gas-flow-soil-core technique. Environ. Sci. Techno., 45, 6066–6072. doi:10.1021/es1036578
- Whalen K, Chang C, Clayton GW, Carefoot JP 2000: Cattle manure amendments can increase the pH of acid soils. Soil Sci. Soc. Am. J., 64, 962–966. doi:10.2136/sssaj2000.643962x
- Whitehead DC, Bristow AW, Lockyer DR 1990: Organic matter and nitrogen in the unharvested fractions of grass swards in relation to the potential for nitrate leaching after ploughing. Plant Soil, 123, 39–49. doi:10.1007/BF00009924
- Whittaker ET, Robinson G 1967: Trapezoidal and Parabolic Rules. the Calculus Observation: A Trease of Numerical Mathematics. New York, NY: Dover.
- Wu X, Yao Z, Brüggemann N, Shen ZY, Wolf B, Dannenmann M, Zheng X, Butterbach-Bahl K 2010: Effects of soil moisture and temperature on CO2 and CH4 soil-atmosphere exchange of various land use/cover types in a semi–arid grassland in Inner Mongolia, China. Soil Biol. Biochem., 42, 773–787. doi:10.1016/j.soilbio.2010.01.013
- Yan G, Zheng X, Cui F, Yao Z, Zhou Z, Deng J, Xu Y 2013: Two-year simultaneous records of N2O and NO fluxes from a farmed cropland in the northern China plain with a reduced nitrogen addition rate by one-third. Agric. Ecosys. Environ., 178, 39–50. doi:10.1016/j.agee.2013.06.016
- Yao Z, Wu X, Wolf B, Dannenmann M, Butterbach-Bahl K, Brüggemann N, Chen W, Zheng X 2010: Soil-atmosphere exchange potential of NO and N2O in different land use types of Inner Mongolia as affected by soil temperature, soil moisture, freeze-thaw, and drying-wetting events. J. Geo. Res. Atmospheres, 115, 1–17. doi:10.1029/2009JD013528
- Yonemura S, Nouchi I, Nishimura S, Sakurai G, Togami K, Yagi K 2014: Soil respiration, N2O, and CH4 emissions from an Andisol under conventional-tillage and no-tillage cultivation for 4 years. Biol. Fertil. Soils, 50, 63–74. doi:10.1007/s00374-013-0831-5
- Zhang W, Yu Y, Li T, Sun W, Huang Y 2014: Net greenhouse gas balance in China’s croplands over the last three decades and its mitigation potential, Environ. Sci. Technol., 48, 2589−259.