ABSTRACT
Transferring hydroponically grown Arabidopsis plants (Arabidopsis thaliana L.) to boron (B)-free medium kills the cells in root tips within an hour. To understand the mechanism underlying the induced rapid cell death, the Arabidopsis response to B deprivation was characterized using inhibitors of presumably involved cellular processes. The results suggest that stretching of the plasma membrane and the influx of calcium ions through mechanosensitive channels triggered the responses and that reactive oxygen species were overproduced under B-deprived condition. In addition, nitric oxide was observed to be involved in cell death, suggesting that Arabidopsis root cells undergo programmed cell death upon B deprivation. RNA sequencing analysis revealed that B deprivation induced transcriptome changes that resembled pathogen-induced responses. In summary, we speculate that B deprivation induces hypersensitive responses in Arabidopsis roots as a response to defective cell wall structures.
1. Introduction
Boron (B) is an essential micronutrient for vascular plants. Soils low in B content are distributed worldwide, and B deficiency has been one of the most widespread problems in crop production (Shorrocks Citation1997). Symptoms of B deficiency include various physiological disorders, such as the inhibited expansion of young leaves, cracked stems, and failure to set seed (Broadley et al. Citation2012). Plants absorb B as boric acid, and boric acid is converted to borate diesters that covalently cross-link pectin rhamnogalacturonan II (RG-II) regions in the cell wall (B-RG-II complex) (Kobayashi et al. Citation1996; O’Neill et al. Citation2004). The cross-linked pectin forms a gel that is considered to occur within the cellulose–xyloglucan network and reportedly plays multiple roles, including the maintenance of cell wall mechanical strength, intercellular adhesion, water holding capacity, pH buffering, and ion binding (Ridley et al. Citation2001). Significant contributions of B-RG-II linkage to pectin gel formation have been shown (Kobayashi et al. Citation1999), and mutants for RG-II synthesis have been reported to exhibit reduced cell wall strength and developmental abnormalities (Iwai et al. Citation2002; O’Neill et al. Citation2001; Ryden et al. Citation2003). These findings suggest that physiological disorders observed under B deficiency are caused by the failure in pectin gel formation and consequent cell wall destabilization. However, the exact causal relationship between cell wall structural impairment and various symptoms of B deficiency remains unclear. To understand this association, we have been analyzing B deprivation-induced changes at cellular and molecular levels.
We have previously reported that transferring the suspension-cultured tobacco cells to a B-free medium induces the activation of calcium ion (Ca2+) channels within a few minutes and the expression of stress-responsive genes within 1 h or earlier (Kobayashi et al. Citation2004; Koshiba et al. Citation2010). Such a rapid response has been observed in the roots of intact plants as well (Oiwa et al. Citation2013). Hydroponically cultured Arabidopsis plants exhibited the upregulation of stress-responsive genes within 1 h after transfer to a B-free medium. More remarkably, cell death, detected by Evans blue staining, occurred in the root elongation zone within 1 h of B deprivation. The accumulation of reactive oxygen species (ROS) was observed in the areas stained with Evans blue, suggesting the involvement of ROS in rapid cell death. Similar rapid cell death and ROS accumulation in the root elongation zone were induced by Ca2+ deprivation, another cross-linker of pectin, but not by magnesium or potassium deprivation (Oiwa et al. Citation2013). This difference suggests that the rapid induction of cell death is not simply a response to essential nutrient deprivation, but associated specifically with the failure in pectin cross-linking. Molecular and physiological mechanisms underlying these rapid responses are largely unknown. Therefore, elucidating these mechanisms will enhance our understanding of B significance in the cell wall architecture. In addition, this may contribute to the identification of mechanisms by which plant cells monitor their cell wall integrity. Thus, in this study, we further characterized responses of Arabidopsis plants to B deprivation using compounds affecting the predicted components of the process. We also performed transcriptome analysis of B-deprived roots. Results of these analyses indicate that changes in cell wall mechanical properties, Ca2+ influx, and the production of both ROS and nitric oxide (NO) constitute the signaling pathway leading to rapid cell death, which may be programmed cell death (PCD) activated in response to cell wall destabilization.
2. Materials and methods
2.1. Plant cultivation and B deprivation treatment
Seeds of Arabidopsis thaliana L. ecotype Columbia-0 (Col-0) were surface sterilized and sown in sterile quarter-strength Murashige and Skoog (MS) medium with 1% sucrose (7.5 µM B as boric acid) in 6-well culture dishes (3 mL per well). The seedlings were cultured for 7–8 days after germination in a growth chamber set at 22°C without shaking. For B deprivation treatment, azomethine H at a final concentration of 200 µM was added to the cultures in order to mask B in the medium. Water was added to control samples. After 1-h incubation, seedlings were stained with Evans blue to detect cell death in the root elongation zone. The medium was removed from the wells and 0.05% (w/v) Evans blue was added. After 10-min incubation, the seedlings were rinsed with distilled water several times, mounted on slides, and viewed under a microscope (Olympus BX-51; Olympus, Tokyo, Japan). The scheme of this procedure is illustrated as Supplemental Figure S1. Experiments examining the effects of various compounds on B deprivation-induced cell death were repeated at least three times for each treatment.
The accumulation of superoxide and hydrogen peroxide was detected by staining with fluorescent indicators dihydroethidium (DHE; Sigma-Aldrich) and 3′-O-acetyl-6′-O-pentafluorobenzenesulfonyl-2′,7′-difluorofluorescein (BES-H2O2-Ac; Wako Chemicals, Osaka, Japan), respectively. After 1-h deprivation treatment, the seedlings were stained with 10 µM DHE and 10 µM BES-H2O-Ac, incubated for 20 min at room temperature in the dark, and then observed under a laser scanning confocal microscope (Fluoview FV500, Olympus). Both reagents were diluted from 10 mM stock solutions in dimethylsulfoxide.
Nitric oxide was detected using diaminorhodamine-4M acetoxymethyl ester (DAR-4M AM; Goryo Chemicals, Sapporo, Japan). The reagent was added to the medium at the onset of the deprivation treatment at a final concentration of 10 µM, diluted from a 5 mM stock solution in dimethylsulfoxide. After 1-h deprivation treatment in the dark, seedlings were mounted on slides and observed under a laser scanning confocal microscope (Fluoview FV500).
2.2. RNA isolation and sequencing
Roots from B-deprived or control seedlings were cut and used for RNA extraction. Roots of 10–11 seedlings were collected and homogenized using Minilys tissue homogenizer (Bertin Technologies, Paris, France) in the extraction buffer of the Total RNA Extraction Kit (Plant) (RBC Bioscience, New Taipei City, Taiwan). Total RNA was then purified from the homogenate using the kit according to the manufacturer’s instruction with on-column deoxyribonuclease treatment. After confirming the integrity of RNA by electrophoresis on Agilent 2100 Bioanalyzer, mRNA library was constructed using an Illumina TruSeq RNA Sample Prep Kit v2 (Illumina, San Diego, CA, USA); the libraries were pooled with each volume after purification and equalization using an Agencourt AMPure XP system (Beckman Coulter, Brea, CA, USA), following the manufacturer’s instructions. The paired-end sequences in the pooled library were determined using an Illumina MiSeq sequencer with MiSeq Reagent Kit v3 (150 cycles) (Illumina). For each sample, 3–4 million paired-end reads were obtained. The short read data sets were deposited in the DDBJ Sequence Read Archive under accession number DRA006216.
2.3. Differential gene expression analysis
The sequences were quality-checked using FastQC software (https://www.bioinformatics.babraham.ac.uk/projects/fastqc/) and were trimmed using Trimmomatic version 0.36 (Bolger et al. Citation2014). The trimmed sequences were mapped to the A. thaliana gene model TAIR10 using TopHat version 2.0.13 (Kim et al. Citation2013) with bowtie2 version 2.2.4, and transcript abundances were estimated using cufflinks version 2.2.1 (Trapnell et al. Citation2010). Count data from three biological replicates for each treatment were used to estimate differential gene expression using cuffdiff version 2.2.1, setting the false discovery rate (FDR) ≤0.05 as the significance threshold and including quartile normalization.
2.4. Reagents
Diphenylene iodonium (DPI) was obtained from Toronto Research Chemicals (Ontario, Canada). 2,4,6-Trimethylpiperidine (TMP) was obtained from Nacalai Tesuque (Kyoto, Japan). NG-Monomethyl-l-arginine (l-NMMA) and 2-(4-carboxyphenyl)-4,4,5,5-tetramethylimidazoline-1-oxyl-3-oxide (cPTIO) were obtained from Dojin Chemicals (Kumamoto, Japan).
3. Results
3.1. Improvement of the experimental system for B deprivation
We have previously reported that rapid responses to B deprivation, including cell death in the root elongation zone or stress gene expression could be induced by transferring hydroponically cultured Arabidopsis plants to a B-free medium (Oiwa et al. Citation2013). Examining whether B deprivation responses are altered by various compounds can be useful to characterize the mechanism underlying rapid responses. However, the conventional method requires several liters of culture solution for the deprivation treatment; thus it is rather difficult to examine the effect of expensive compounds using the system. Therefore, in the present study, we developed a small-scale experimental system capable of inducing rapid B deprivation responses in Arabidopsis. Several seedlings were axenically grown in 3 mL of quarter-strength MS medium in polystyrene dishes. Simply exchanging the external solution from the growing medium to a B-free medium failed to induce rapid cell death; this was probably due to the carry-over of B from the growing medium retained on the seedling surfaces. Therefore, we performed B deprivation treatment by adding 200 µM of azomethine H, a compound that forms complexes with boric acid, to the medium without prior rinsing of the seedlings. Concentration of B (boric acid) in the growth medium was 7.5 µM, which was expected to be masked and made unavailable for the seedlings following azomethine H addition at more than 20-fold molar excess. As shown in , azomethine H addition to the medium resulted in staining with Evans blue in the root elongation zone within 1 h, similar to that observed with the conventional method of B deprivation (Oiwa et al. Citation2013). Additionally, the treatment caused darkening of the root tips, which has been occasionally observed in B-deprived roots (Oiwa et al. Citation2013). These changes in appearance were greatly reduced when a fivefold molar excess of boric acid (1 mM) was added to the medium together with azomethine H (), indicating that the responses observed upon this treatment were induced by the unavailability of B in the medium. This system has several advantages over the conventional method. First, the small volume of media required for the treatment makes it feasible to test expensive compounds as modifiers of the responses. Second, the time required to grow the plants for the experiment is 7–8 days after germination, which is much shorter than that required for the conventional method (5 weeks). Third, the system avoids the removal of growing medium or repeated washing, which are treatments possibly imposing mechanical damage on the plants, potentially complicating the interpretation of the results. Taken together, we consider that our experimental system is useful to induce rapid B deprivation responses in Arabidopsis. Therefore, we used this system for the following study.
Figure 1. Induction of cell death by azomethine H treatment in Arabidopsis roots. To the medium of liquid-cultured Arabidopsis seedlings, water (Control), 200 µM azomethine H (Azomethine), or 200 µM azomethine H plus 1 mM boric acid (Azomethine+B) were added. After incubation for 1 h, seedlings were stained with Evans blue to detect dead cells. An arrow indicates the region intensely stained with Evans blue. Scale bar = 500 µm.
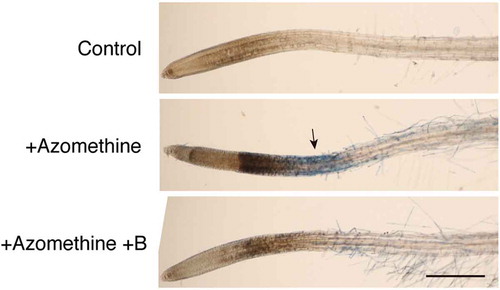
3.2. Molecules involved in rapid responses to B deprivation
We have previously reported ROS accumulation in the elongation zone of B-deprived Arabidopsis roots, suggesting the involvement of ROS in rapid B deprivation responses (Oiwa et al. Citation2013). In addition, Ca2+ influx has been implicated in the responses, because transferring suspension-cultured tobacco cells to B-free medium induced the activation of Ca2+ channels within a few minutes of transfer, and the deprivation-induced expression of stress-responsive genes was inhibited by a Ca2+ channel blocker (Koshiba et al. Citation2010). On the basis of these findings, we constructed a working hypothesis for the mechanism of rapid B deprivation responses in Arabidopsis, presented in . Plant cell walls enclose the protoplasm, counteracting osmotic pressure. When B is not available to form B-RG-II cross-links in the cell wall pectin, the tensile strength of cell wall would not be maintained (Ryden et al. Citation2003) and the protoplasm would expand. This change would impose stretching forces on the plasma membrane, which may activate mechanosensitive channels in the plasma membrane to allow Ca2+ influx. Consequently, the increased Ca2+ concentration may trigger various cellular responses, including ROS production. If this model is valid, cell death can be mitigated by blocking any step in this sequence. Thus, we examined the effects of inhibitors for each supposed step.
Figure 2. A working hypothesis for the immediate responses to boron (B) deprivation in Arabidopsis roots. B deprivation induces incomplete cross-linking of pectin thereby decreasing the tensile strength of the cell wall. The change may allow protoplasts to absorb water, which in turn causes the stretching of the plasma membrane. The mechanical change is converted to a cellular signal as calcium ions (Ca2+) enter through the activation of mechanosensitive calcium channels to induce downstream responses, including the overproduction of reactive oxygen species (ROS) and consequent cell death.
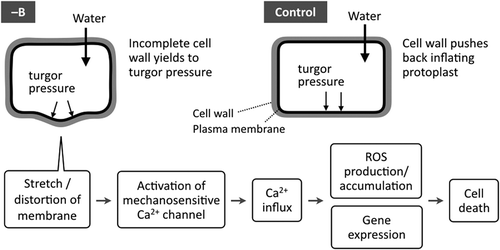
We first examined whether ROS scavengers modified rapid cell death under B deprivation. As shown in , B deprivation induced darkening and Evans blue uptake in the root tips. However, the simultaneous addition of 1 mM TMP as an ROS scavenger almost completely inhibited the reaction (). This result supports the hypothesis that ROS production is associated with rapid B deprivation responses in Arabidopsis roots. To identify the specific type of ROS critical for the response, B-deprived roots were double-stained with DHE and BES-H2O2-Ac, which preferentially detect superoxide and hydrogen peroxide, respectively (Tsukagoshi et al. Citation2010). Compared with control roots, B-deprived roots emitted more intense DHE-derived ethidium fluorescence in the same region as that stained with Evans blue, whereas no clear difference could be observed between the treatment using BES-H2O2-Ac (), suggesting that superoxide was involved in cell death. Consistent with this assumption, DPI, an inhibitor of superoxide-producing NADPH oxidases on the plasma membrane, mitigated B deprivation responses in a dose-dependent manner ().
Figure 3. Involvement of reactive oxygen species (ROS) in rapid cell death induced by B deprivation. (a) Effects of an ROS scavenger and inhibitor on ROS synthesis. Hydroponically cultured Arabidopsis seedlings were treated with water (Control) or azomethine H (−B) as in . To examine the possible involvement of ROS in cell death, either TMP (1 mM), as a superoxide scavenger, or DPI (1 or 10 µM), as an inhibitor of superoxide synthesis, was added to the medium together with azomethine (−B+TMP, −B+DPI 1 µM, and −B+DPI 10 µM, respectively). Scale bar = 500 µm. (b) Detection of ROS in B-deprived Arabidopsis roots. Seedlings were treated with water (Control) or azomethine H (−B) for 1 h and then subjected to double staining with dihydroethidium (DHE) and 3′-O-acetyl-6′-O-pentafluorobenzenesulfonyl-2′,7′-difluorofluorescein (BES-H2O2-Ac), which preferentially react with superoxide or hydrogen peroxide, respectively. The roots were observed under a confocal laser scanning microscope. The bottom panels show merged images of DHE- and BES-derived fluorescence, in which signals from DHE and BES are shown in magenta and green pseudo-colors, respectively. Double-headed arrows indicate the region corresponding to the region intensely stained with Evans blue. Scale bar = 500 µm.
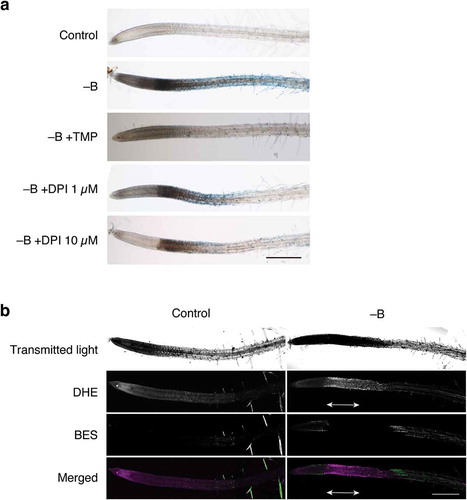
To explore the possibility that Ca2+ influx plays a role in rapid B deprivation responses in Arabidopsis roots, we examined the effect of lanthanum ion (La3+), a Ca2+ channel blocker, on rapid cell death under B deprivation. As shown in , La3+ addition effectively suppressed root darkening and Evans blue staining in response to B deprivation. This result confirms that Ca2+ influx is indeed involved in rapid B deprivation responses in Arabidopsis roots. We further hypothesized that Ca2+ influx is mediated by mechanosensitive channels on the plasma membrane (). Findeklee and Goldbach (Citation1996) have reported that the mechanical strength of the cell wall could rapidly decrease upon B deprivation. The weakened cell wall may not be able to counteract protoplast turgor, allowing the plasma membrane to stretch and activate mechanosensitive channels. A similar mechanism is known to operate in yeast for the maintenance of cell wall integrity (Levin Citation2005). Based on this, osmoticum addition to the external medium should reduce the turgor pressure, thereby suppressing the cellular responses. Therefore, we added 0.15 M sorbitol to the medium, as this treatment has been shown to suppress lignin deposition in Arabidopsis roots as a response to inhibited cellulose biosynthesis (Hamann et al. Citation2009). The treatment indeed mitigated Evans blue staining in elongation zone and tip darkening of roots in response to B deprivation (). We also observed that the addition of sorbitol or La3+ to the medium decreased DHE-derived ethidium fluorescence in the roots deprived of B (Supplemental Figure S2), suggesting that B deprivation-induced ROS overproduction is downstream of the putative Ca2+ influx through mechanosensitive channels. Overall, these results support our working hypothesis presented in , which suggests that the decreased mechanical strength of the cell wall and the consequent Ca2+ influx through activated mechanosensitive channels trigger rapid B deprivation response in Arabidopsis.
Figure 4. Involvement of calcium influx and membrane stretching in rapid cell death induced by boron (B) deprivation. Hydroponically cultured Arabidopsis seedlings were treated with water (Control) or azomethine H (−B) as in . To examine the possible involvement of calcium influx through mechanosensitive channels in cell death, 30 µM lanthanum ion, as a channel blocker, (−B+La) or 0.15 M sorbitol, as an osmoticum, (−B+sorbitol) was added together with azomethine H. Asterisk and arrowhead in the bottom panel indicate the region where darkening and Evans blue staining was seen in B-deprived roots (–B), respectively. Scale bar = 500 µm.
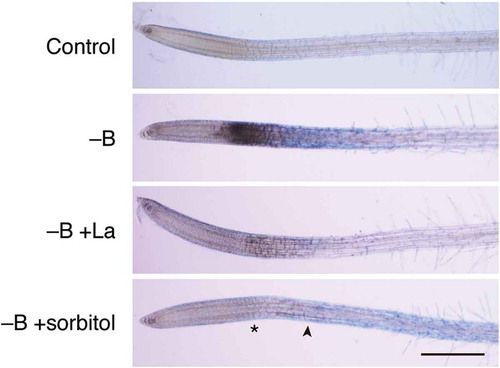
During the course of examining effects of various compounds on B deprivation responses, we also observed that 0.5 mM cPTIO, a scavenger of nitric oxide (NO), almost completely suppressed rapid cell death (). l-NMMA is an inhibitor of mammalian NO synthase and is capable of blocking NO synthesis in plants as well. The addition of 1 mM l-NMMA to B-free medium effectively suppressed cell death (). Staining B-deprived roots with DAR-4M, a fluorescent probe for NO, detected a signal in the same region in elongation zone as that stained with Evans blue (). These results together indicate that NO is produced upon B deprivation, and it is associated with rapid B deprivation responses in Arabidopsis roots.
Figure 5. Involvement of nitric oxide (NO) in rapid cell death induced by boron (B) deprivation. (a) Effects of an NO synthesis inhibitor and an NO scavenger on cell death. Hydroponically cultured Arabidopsis seedlings were treated with water (Control) or azomethine H (−B) as in . To examine the possible involvement of NO in cell death, 1 mM NG-monomethyl-l-arginine, as an inhibitor of NO synthesis, (−B+NMMA) or 2-(4-carboxyphenyl)-4,4,5,5-tetramethylimidazoline-1-oxyl-3-oxide, as an NO scavenger, (−B+cPTIO) was added together with azomethine H. Scale bar = 500 µm. (b) Detection of NO in B-deprived roots. Seedlings were treated with water (Control), azomethine H (−B), or azomethine H plus NMMA (−B+NMMA) for 1 h. Seedlings were stained with 10 µM diaminorhodamine-4M acetoxymethyl ester (DAR-4M), incubated for 1 h in the dark, and observed under a confocal laser scanning microscope. The bottom panels show merged images from transmitted light images and DAR-4M signals. Scale bar = 500 µm.
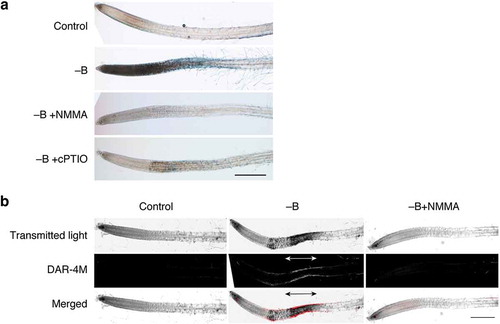
3.3. Gene expression in B-deprived roots
As another method to explore the mechanism underlying rapid B deprivation responses in Arabidopsis roots, transcriptome changes in response to B deprivation were analyzed by RNA sequencing. Transcript abundance was measured in control and B-deprived roots and differential gene expression was analyzed. By setting the threshold of significance to FDR ≤0.05 and the absolute value of log2 fold change ≥1, respectively 1264 and 996 upregulated and downregulated genes were identified in B-deprived roots (). The genes differentially expressed between the control and B-deprived roots are summarized in Tables S1 (upregulated) and S2 (downregulated). We previously reported several Arabidopsis genes as B deprivation-inducible (Oiwa et al. Citation2013). Among the genes, MPK3 (At3g45640), WRKY40 (At1g80840), AOX (At3g22370), and ERF2 (At5g47220) were upregulated also in this study (Table S1). On the other hand, expressions of MPK6 (At2g43790), PAL (At2g37040), NIMIN2a (At3g25882), and ChitIV (At3g54420) were not significantly different between control and B-deprived roots. Expression of NHL10 (At2g35980) was rather suppressed under B deprivation (Table S2). Takano et al. (Citation2006) reported that NIP5;1 (At4g10380), a boric acid channel gene, could be upregulated by B deprivation within 3 h. On the other hand, in this study the gene was found to be downregulated at 1 h after treatment. At present, the reason for these inconsistencies is unclear, but it might be due to differences in the experimental setup; plants were grown hydroponically and deprived of B by transferring to B-free medium in the previous studies, whereas the seedlings grown in an axenic liquid culture were deprived of B by masking medium B with a boric acid chelator in this study.
Figure 6. Scatter plot of expression values of genes in B-deprived and control samples. Log 2 values of fragments per kilobase of exon per million mapped sequence reads (FPKM) in each sample are plotted. Genes with significant differences in expression between B-deprived and control sample [false discovery rate ≤0.05 and an absolute value of log2 (fold change) ≥1] are shown as black dots, whereas those without significant differences are shown as gray dots.
![Figure 6. Scatter plot of expression values of genes in B-deprived and control samples. Log 2 values of fragments per kilobase of exon per million mapped sequence reads (FPKM) in each sample are plotted. Genes with significant differences in expression between B-deprived and control sample [false discovery rate ≤0.05 and an absolute value of log2 (fold change) ≥1] are shown as black dots, whereas those without significant differences are shown as gray dots.](/cms/asset/5e4d6312-b602-4aea-9da0-e97dee179fa3/tssp_a_1416670_f0006_b.gif)
To obtain insights into the biological context of the responses, a gene ontology term enrichment analysis was performed for the differentially expressed genes using the DAVID 6.8 web tool (Huang et al. Citation2009a; Huang et al. Citation2009b; https://david.ncifcrf.gov). The analysis revealed more than 20 biological processes overrepresented (p ≤ 0.01 and FDR ≤0.05) among the genes upregulated by B deprivation ( and Supplemental Table S3). Remarkable enrichment could be observed for genes associated with the interaction with other organisms, including response to chitin, defense response to bacterium, response to bacterium, and defense response to insect (). In addition, enrichment was also observed for the genes involved in wounding and jasmonic acid signaling, including response to wounding, response to jasmonic acid, regulation of jasmonic acid-mediated signaling pathway, or jasmonic acid biosynthetic process (). The enrichment of these genes implies that B deprivation caused transcriptome changes similar to those induced by pathogen attack. Genes associated with other abiotic stress responses were also enriched (), suggesting that a part of the transcriptome change may represent common stress responses. Genes downregulated by B deprivation included those associated with cell wall structure, including plant-type cell wall organization and plant-type cell wall modification involved in multidimensional cell growth (). Genes involved in flavonoid biosynthesis were also overrepresented in the downregulated set of genes ().
Table 1. Gene ontology term enrichment analysis of the genes induced by boron (B) deprivation for 1 h.
We further characterized the B deprivation-induced transcriptome change through a comparison with the known changes induced under various conditions using the Plant Gene Set Enrichment Analysis (PlantGSEA; http://structuralbiology.cau.edu.cn/PlantGSEA/) tool kit under default parameters (Yi et al. Citation2013). Literature-derived gene sets were employed as predefined gene sets in the analysis. Top 10 gene sets with the highest overlap with genes up- or downregulated in B-deprived roots are summarized in . The gene sets overlapping with the genes upregulated by B deprivation include those responsive to pathogenesis, such as ‘RAMONELL_CRAB-SHELL-CHITIN-TREATED_CHITO-OCTAMER-TREATED_SEEDLING_UP’ (Ramonell et al. Citation2005), ‘WAN_WT_CHITOOCTAOSE-TREATED_WATER-TREATED_DIFF’, or ‘WAN_FLG_ELF26_CHITOOCTAOSE_COMMON_DIFF’ (Wan et al. Citation2008), which are upregulated by the application of chitin oligomer, or ‘BAE_NEP1_30MIN_UP’, which is induced by the application of necrosis- and ethylene-inducing peptide (Nep1) produced by Fusarium oxysporum (Bae et al. Citation2006). The similarity of expression profiles between B deprivation and pathogenesis genes is consistent with the result obtained with gene ontology term enrichment analysis (). Overlap with ‘LEE_FLU_WT_UP’ (Lee et al. Citation2007) suggests that some of the genes induced may reflect the plant response to ROS accumulation (). Genes suppressed by B deprivation also showed an overlap with the immunity-related set ‘RAMONELL_CRAB-SHELL-CHITIN-TREATED_CHITO-OCTAMER-TREATED_SEEDLING_DN’ (Ramonell et al. Citation2005). Taken together, B deprivation may induce physiological changes similar to those observed upon pathogenesis.
Table 2. Top 10 gene sets with the highest overlap with B deprivation-induced genes observed using the gene set enrichment analysis.
4. Discussion
We have previously reported that Arabidopsis roots immediately respond to B deprivation, as revealed by cell death in the elongation zone and the upregulation of stress-responsive genes within 1 h of treatment (Oiwa et al. Citation2013). In our previous report, we hypothesized the involvement of Ca2+ influx and ROS production in the responses; however, experimental data substantiating the hypothesis was not provided. In this study, we developed a working hypothesis for the mechanism of rapid B deprivation responses in Arabidopsis taking into account the possible involvement of Ca2+ influx and ROS production () and examined the validity of this model using compounds known to perturb the assumed processes. We observed that a Ca2+ channel blocker, an ROS scavenger, and an ROS generation inhibitor effectively mitigated B deprivation-induced cell death ( and ). These results confirm that Ca2+ and ROS are indeed involved in the rapid B deprivation responses. In addition, supplementing the medium with osmoticum also mitigated rapid cell death (), supporting our hypothesis that the stretching of the plasma membranes due to a loss of cell wall strength plays a role in the responses. The reduction in tensile strength of the cell wall can occur immediately after B deprivation, which has been shown by the immediate and transient decrease in the mechanical strength of squash cell walls upon B deprivation (Findeklee and Goldbach Citation1996). The consequent Ca2+ influx can activate various cellular processes, including ROS production by membrane-bound NADPH oxidases (Sagi and Fluhr Citation2006). Thus, the loss of cell wall integrity can initiate stress signaling immediately after B deprivation and may not necessarily require a dedicated sensor molecule for external B concentration. Notably, rapid cell death occurred only in the elongation zone (; Oiwa et al. Citation2013), implying that B deprivation exerts significant effects through the failure in cross-linking of newly synthesized pectin but not through the collapse of existing B-RG-II linkages.
A key question regarding the Arabidopsis response to B deprivation is the reason for the rapidity of cell death induction. Cells stained with Evans blue could be observed already at 0.5 h after B deprivation (data not shown). We have previously revealed that, in tobacco BY-2 cells, B deprivation causes necrotic cell death due to oxidative damage (Koshiba et al. Citation2009). As discussed above, B deprivation can impair the structural integrity of newly synthesized cell walls in growing tissues, which may lead to ROS overproduction. Nonetheless, 0.5 h appears to be a very short duration for ROS to accumulate to a level causing catastrophic cell death. Consistent with this assumption, the cell death of tobacco cells was not obvious within the initial 0.5 h (Koshiba et al. Citation2009), despite the fact that their metabolism had been significantly impacted by that time (Koshiba et al. Citation2013). Therefore, we speculated that rapid cell death in Arabidopsis roots might be of a spontaneous nature, as in the case of PCD. The speculation is supported by the finding that NO was produced in B-deprived roots and the inhibition of NO accumulation prevented rapid cell death (). It is well established that NO is required for the execution of PCD under abiotic or biotic stress conditions (Groß et al. Citation2013).
If this rapid cell death occurs as PCD, the question arises why B deprivation activates PCD. Transcriptome analysis in the present study revealed that B deprivation induced a change similar to pathogen responses. Plant cells recognize pathogen attack using receptors that bind pathogen-derived molecules, such as flagellin or chitin (Jones and Dangl Citation2006). Additionally, plants may perceive the attack through the action of mechanosensors, such as mechanosensitive channels on plasma membranes (Hématy et al. Citation2009), as the pathogens often degrade the host cell wall as a physical barrier to penetrate. As discussed, B deprivation perhaps destabilizes the cell wall; therefore, the signal transduction pathways triggered by B deprivation and pathogen attack possibly converge to common downstream events. Hypersensitive responses (HR) are often seen in plant cells attacked by pathogens and include the induction of PCD in ROS- and NO-dependent manners (Hong et al. Citation2008). Hence, B-deprived root cells may undergo PCD, as a part of an HR induced by the destabilized cell wall structure. In the present study, it should be noted that the transcriptome change due to B deprivation was similar to that triggered by chitin, flagellin, or bacterial translation elongation factor (). These molecules are regarded as evolutionarily conserved microbial components and are referred to as pathogen-associated molecular patterns (PAMPs). Classically, PAMP-triggered immunity (PTI) was not believed to induce HR, whereas the immunity triggered by species-, race-, or strain-specific effector molecules [effector-triggered immunity (ETI)] induces HR (Jones and Dangl Citation2006). However, more recent studies suggest that PTI and ETI are not strictly separated but are continuous systems, sharing many signal transduction components as well as downstream events (Thomma et al. Citation2011). In fact, Naito et al. (Citation2007) have demonstrated that flagellin, a type of PAMP, indeed induced HR in Arabidopsis. Thus, defective cell wall structures possibly induce HR during B deprivation, considering that the downstream events are common with PTI. Disrupted cell wall structures are often associated with PCD. Duval et al. (Citation2005) have reported the induction of PCD in Arabidopsis cells treated with inhibitors of cellulose biosynthesis. Arabidopsis plants with inhibited biosynthesis of apiose, a sugar in RG-II, have been reported to undergo cell death (Ahn et al. Citation2006). These cell deaths may also be induced by the similar mechanism as discussed here.
We have previously reported tobacco cells cultured in B-free medium experience cell death due to oxidative damage and that this cell death is not PCD (Koshiba et al. Citation2009). This finding apparently contradicts the results of the present study, where rapid cell death in B-deprived Arabidopsis roots may be PCD. This apparent discrepancy is perhaps because the rapid cell death in B-deprived Arabidopsis roots is induced by a distinct mechanism from that induces the death of tobacco cells observed after longer duration of B deprivation. As discussed above, rapid cell death observed in the present study may be a part of HR; thus, it can be considered as a type of overreaction. Destabilization of cell wall structure due to insufficient pectin cross-linking may be severe enough to induce various metabolic changes; however, this may not be fatal within 1 h of B deprivation. In the case of cultured tobacco cells, stress-inducible genes are upregulated within 1 h of B deprivation, and plasma membrane Ca2+ channels activate within a few minutes in an ROS-dependent manner (Koshiba et al. Citation2010). Therefore, cultured tobacco cells also immediately respond to B deprivation; nonetheless, they do not die within 1 h, but take more than 24 h to show a decrease in viability (Koshiba et al. Citation2009). Apparently, an HR-like PCD can be induced in Arabidopsis roots but not in tobacco cells after B deprivation. This difference in lethality is probably due to the concentration of ROS produced upon B deprivation. As discussed by Koshiba et al. (Citation2009), ROS produced in B-deprived tobacco cells may not be high enough to induce PCD immediately; however, persistent ROS production and consequent ROS accumulation can induce oxidative damages and necrotic cell death. It is also suggested that rapid cell death observed in the present study may be a response to the sudden change in external B concentration and would not be seen in the case of chronic deficiency. Nonetheless, the events observed upon B deprivation treatment can represent the plant response to defective pectin cross-linking. Further analysis of these responses will provide a better understanding of the physiological importance of pectin gels and the mechanism of sensing cell wall–cytoplasm integrity in plants.
Supplementary_files.zip
Download Zip (2.4 MB)Acknowledgments
We thank Ms. Sayaka Shinpo (Kazusa DNA Research Institute) for the RNA sequencing analysis. We also thank Dr. Masanori Kaido (Graduate School of Agriculture, Kyoto University) for providing the laser confocal scanning microscope facility.
Supplemental Data
Supplemental data for this article can be accessed here.
Additional information
Funding
References
- Ahn J-W, Verma R, Kim M, Lee J-Y, Kim Y-K, Bang J-W, Reiter W-D, Pai H-S 2006: Depletion of UDP-d-apiose/UDP-d-xylose synthases results in rhamnogalacturonan-II deficiency, cell wall thickening, and cell death in higher plants. J. Biol. Chem., 281, 13708–13716. doi:10.1074/jbc.M512403200
- Bae H, Kim MS, Sicher RC, Bae H-J, Bailey BA 2006: Necrosis- and ethylene-inducing peptide from fusarium oxysporum induces a complex cascade of transcripts associated with signal transduction and cell death in Arabidopsis. Plant Physiol., 141, 1056–1067. doi:10.1104/pp.106.076869
- Bolger AM, Lohse M, Usadel B 2014: Trimmomatic: a flexible trimmer for Illumina sequence data. Bioinformatics, 30, 2114–2120. doi:10.1093/bioinformatics/btu170
- Broadley M, Brown P, Cakmak I, Rengel Z, Zhao F 2012: Function of nutrients: micronutrients. In Marschner’s mineral nutrition of higher plants, Ed. Marschner P, pp. 191–248. Academic Press, San Diego.
- Duval I, Brochu V, Simard M, Beaulieu C, Beaudoin N 2005: Thaxtomin A induces programmed cell death in Arabidopsis thaliana suspension-cultured cells. Planta, 222, 820–831. doi:10.1007/s00425-005-0016-z
- Findeklee P, Goldbach HE 1996: Rapid effects of boron deficiency on cell wall elasticity modulus in Cucurbita pepo roots. Bot. Acta, 109, 463–465. doi:10.1111/j.1438-8677.1996.tb00599.x
- Groß F, Durner J, Gaupels F 2013: Nitric oxide, antioxidants and prooxidants in plant defence responses. Front. Plant Sci., 4, 419. doi:10.3389/fpls.2013.00419
- Hamann T, Bennett M, Mansfield J, Somerville C 2009: Identification of cell-wall stress as a hexose-dependent and osmosensitive regulator of plant responses. Plant J., 57, 1015–1026. doi:10.1111/j.1365-313X.2008.03744.x
- Hématy K, Cherk C, Somerville S 2009: Host-pathogen warfare at the plant cell wall. Curr. Opin. Plant Biol., 12, 406–413. doi:10.1016/j.pbi.2009.06.007
- Hong JK, Yun BW, Kang JG, Raja MU, Kwon E, Sorhagen K, Chu C, Wang Y, Loake GJ 2008: Nitric oxide function and signalling in plant disease resistance. J. Exp. Bot., 59, 147–154. doi:10.1093/jxb/erm244
- Huang DW, Sherman BT, Lempicki RA 2009a: Bioinformatics enrichment tools: paths toward the comprehensive functional analysis of large gene lists. Nucleic Acids Res., 37, 1–13. doi:10.1093/nar/gkn923
- Huang DW, Sherman BT, Lempicki RA 2009b: Systematic and integrative analysis of large gene lists using DAVID bioinformatics resources. Nat. Protoc., 4, 44–57. doi:10.1038/nprot.2008.211
- Iwai H, Masaoka N, Ishii T, Satoh S 2002: A pectin glucuronyltransferase gene is essential for intercellular attachment in the plant meristem. Proc. Natl. Acad. Sci. USA, 99, 16319–16324. doi:10.1073/pnas.252530499
- Jones JDG, Dangl JL 2006: The plant immune system. Nature, 444, 323–329. doi:10.1038/nature05286
- Kim D, Pertea G, Trapnell C, Pimentel H, Kelley R, Salzberg SL 2013: TopHat2: accurate alignment of transcriptomes in the presence of insertions, deletions and gene fusions. Genome Biol., 14, R36. doi:10.1186/gb-2013-14-4-r36
- Kobayashi M, Matoh T, Azuma J 1996: Two chains of rhamnogalacturonan II are cross-linked by borate-diol ester bonds in higher plant cell walls. Plant Physiol., 110, 1017–1020. doi:10.1104/pp.110.3.1017
- Kobayashi M, Mutoh T, Matoh T 2004: Boron nutrition of cultured tobacco BY-2 cells. IV. Genes induced under low boron supply. J. Exp. Bot., 55, 1441–1443. doi:10.1093/jxb/erh142
- Kobayashi M, Nakagawa H, Asaka T, Matoh T 1999: Borate-rhamnogalacturonan II bonding reinforced by Ca2+ retains pectic polysaccharides in higher-plant cell walls. Plant Physiol., 119, 199–204. doi:10.1104/pp.119.1.199
- Koshiba T, Kobayashi M, Ishihara A, Matoh T 2010: Boron nutrition of cultured tobacco BY-2 cells. VI. Calcium is involved in early responses to boron deprivation. Plant Cell Physiol., 51, 323–327. doi:10.1093/pcp/pcp179
- Koshiba T, Kobayashi M, Matoh T 2009: Boron nutrition of tobacco BY-2 cells. V. Oxidative damage is the major cause of cell death induced by boron deprivation. Plant Cell Physiol., 50, 26–36. doi:10.1093/pcp/pcn184
- Koshiba T, Kobayashi M, Matsuoka K, Fujiwara T, Matoh T 2013: Boron nutrition of cultured tobacco BY-2 cells. VII. Rapid induction of metabolic dysfunction in cells deprived of boron as revealed by microarray analysis. Soil Sci. Plant Nutr., 59, 189–194. doi:10.1080/00380768.2013.763383
- Lee KP, Kim C, Landgraf F, Apel K 2007: EXECUTER1- and EXECUTER2-dependent transfer of stress-related signals from the plastid to the nucleus of Arabidopsis thaliana. Proc. Natl. Acad. Sci. USA, 104, 10270–10275. doi:10.1073/pnas.0702061104
- Levin DE 2005: Cell wall integrity signaling in Saccharomyces cerevisiae. Microbiol. Mol. Biol. Rev., 69, 262–291. doi:10.1128/MMBR.69.2.262-291.2005
- Naito K, Ishiga Y, Toyoda K, Shiraishi T, Ichinose Y 2007: N-terminal domain including conserved flg22 is required for flagellin-induced hypersensitive cell death in Arabidopsis thaliana. J. Gen. Plant Pathol., 73, 281–285. doi:10.1007/s10327-007-0017-9
- O’Neill MA, Eberhard S, Albersheim P, Darvill AG 2001: Requirement of borate cross-linking of cell wall rhamnogalacturonan II for Arabidopsis growth. Science, 294, 846–849. doi:10.1126/science.1062319
- O’Neill MA, Ishii T, Albersheim P, Darvill AG 2004: Rhamnogalacturonan II: structure and function of a borate cross-linked cell wall pectic polysaccharide. Annu. Rev. Plant Biol., 55, 109–139. doi:10.1146/annurev.arplant.55.031903.141750
- Oiwa Y, Kitayama K, Kobayashi M, Matoh T 2013: Boron deprivation immediately causes cell death in growing roots of Arabidopsis thaliana (L.) Heynh. Soil Sci. Plant Nutr., 59, 621–627. doi:10.1080/00380768.2013.813382
- Ramonell K, Berrocal-Lobo M, Koh S, Wan J, Edwards H, Stacey G, Somerville S 2005: Loss-of-function mutations in chitin responsive genes show increased susceptibility to the powdery mildew pathogen Erysiphe cichoracearum. Plant Physiol., 138, 1027–1036. doi:10.1104/pp.105.060947
- Ridley BL, O’Neill MA, Mohnen D 2001: Pectins: structure, biosynthesis, and oligogalacturonide-related signaling. Phytochemistry, 57, 929–967. doi:10.1016/S0031-9422(01)00113-3
- Ryden P, Sugimoto-Shirasu K, Smith AC, Findlay K, Reiter W-D, McCann MC 2003: Tensile properties of Arabidopsis cell walls depend on both a xyloglucan cross-linked microfibrillar network and rhamnogalacturonan II-borate complexes. Plant Physiol., 132, 1033–1040. doi:10.1104/pp.103.021873
- Sagi M, Fluhr R 2006: Production of reactive oxygen species by plant NADPH oxidases. Plant Physiol., 141, 336–340. doi:10.1104/pp.106.078089
- Shorrocks V 1997: The occurrence and correction of boron deficiency. Plant Soil., 193, 121–148. doi:10.1023/A:1004216126069
- Takano J, Wada M, Ludewig U, Schaaf G, Von Wirén N, Fujiwara T 2006: The Arabidopsis major intrinsic protein NIP5;1 is essential for efficient boron uptake and plant development under boron limitation. Plant Cell, 18, 1498–1509. doi:10.1105/tpc.106.041640
- Thomma BPHJ, Nürnberger T, Joosten MHAJ 2011: Of PAMPs and effectors: the blurred PTI-ETI dichotomy. Plant Cell, 23, 4–15. doi:10.1105/tpc.110.082602
- Trapnell C, Williams BA, Pertea G, Mortazavi A, Kwan G, Van Baren MJ, Salzberg SL, Wold BJ, Pachter L 2010: Transcript assembly and quantification by RNA-Seq reveals unannotated transcripts and isoform switching during cell differentiation. Nat. Biotechnol., 28, 511–515. doi:10.1038/nbt.1621
- Tsukagoshi H, Busch W, Benfey PN 2010: Transcriptional regulation of ROS controls transition from proliferation to differentiation in the root. Cell, 143, 606–616. doi:10.1016/j.cell.2010.10.020
- Wan J, Zhang X-C, Neece D, Ramonell KM, Clough S, Kim S-Y, Stacey MG, Stacey G 2008: A LysM receptor-like kinase plays a critical role in chitin signaling and fungal resistance in Arabidopsis. Plant Cell, 20, 471–481. doi:10.1105/tpc.107.056754
- Yi X, Du Z, Su Z 2013: PlantGSEA: a gene set enrichment analysis toolkit for plant community. Nucleic Acids Res., 41, W98–W103. doi:10.1093/nar/gkt281