ABSTRACT
Soil salinity is a major limiting factor for crop production in arid and semi-arid regions of northwest China. Flue gas desulfurization gypsum (FGDG) is valuable waste resource which can be used to improve saline soil. Monolith lysimeter leaching experiment was conducted with FGDG in heavily saline-sodic soil of northwest China. The four FGDG treatments with nine replicates for each treatment were applied when the FGDG rate was 0, 15, 30, and 60 t ha−1, respectively. Undisturbed sodic-saline soil was carefully collected in the 40-cm deep soil column. The results indicated that improvement effect on the depth of 0–10 cm soil layer was the best when the rate of FGDG was 60 t ha−1. It can reduce pH by 1.85, exchangeable sodium percentage (ESP) by 44%, and exchangeable Na+ by 7.37 cmol/kg in top soil layer. The values of the above soil parameters fell in the normal range due to FGDG treatment. At the same time, FGDG application reduced soil bulk density and increased saturated hydraulic conductivity. But from the maize growth, the emergence rate, plant height, shoot, and root weight were the best when the FGDG rate was 30 t ha−1. The results also showed that the FGDG application increased soil electrical conductivity value. Therefore, it is quite necessary to move saline ions into the deep soil layer through leaching process. Although effect of FGDG treatment of 60 t ha−1 on top soil was the best, considering the improvement effects of entire soil profile, we recommend that the optimum rate of FGDG was 30 t ha−1.
1. Introduction
Soil salinization and secondary salinization exist worldwide, especially in arid and semi-arid regions (Zhang Citation2004). There are about 3.47 × 107 ha saline soil in China, of which inland soil accounts for 70% of the total saline soil, including those in north-west, north-east, and middle and lower reaches of Yellow River (Wang Citation1993). Sodic-saline soil is one of the inland alkali-saline soil types, with high salt content (mainly Na2CO3 and NaHCO3), very high alkali level, high pH, poor physical properties (poor structure and permeability), resulting in a significant reduction in crop growth and development (Yu and Chen Citation1999; Li et al. Citation2005a). On one hand, the soil contains higher level of soluble salts and thus high osmotic potential, resulting in the so-called plant physiological drought due to limited absorption of water by roots from the soil solution (Yin et al. Citation1998). One the other hand, the sodic-saline soil limits plant absorption of mineral nutrients. Plants reduce absorption of essential mineral nutrients such as potassium and phosphorus because of high Na+, high pH, and alkali level in sodic-saline soil. Available phosphorus significantly declines in alkali environment, resulting in plant malnutrition (Li et al. Citation2005b). Therefore, reduction of soil Na+ content through effective management practices is the primary goal for improving sodic-saline soil.
Gypsum is an excellent amendment to improve sodic-saline soil. As early as late 1900s, Hilgad, American soil scientist, guided farmers to improve sodic-saline soil by using gypsum (Kelly Citation1959; Jenny Citation1988). At the early twentieth century, the release of flue gas from coal-fired power plant caused a significant environmental concern; flue gas desulfurization technology was introduced to fix SO2 in the form of CaSO4, producing a byproduct called flue gas desulfurization gypsum (FGDG). Total amount of FGDG produced in China in 2016 was more than 5.0 × 107 t, which is a potential pollutant to the eco-environment (Sun and Zhang Citation2016). It has received great attention to improve saline soil by using FGDG. In India, research indicated that FGDG application improved sodic-saline soil, reduced pH from 8.8 to 7.5, and increased crop yield by threefold (Crews and Dick Citation1998). Clark and Zetosk (Citation1997) noted that FGDG application can improve saline soil and increase forage yield. Chun et al. (Citation2001) also demonstrated that FGDG application in sodic soil can remove the amount of sodium out of the soil profile and decrease the soil sodium adsorption ration and exchangeable sodium percentage (ESP). Since 2000, scientists in China have conducted research on evaluation of FGDG’s effect on sodic-saline soil improvement, soil environment, and plant growth and development (Wang et al. Citation2005; Zhang et al. Citation2007; Xiao et al. Citation2010; Liu et al. Citation2015; Mao et al. Citation2016). Research results from field environments (Xu et al. Citation2001; Li et al. Citation2006; Zhao et al. Citation2006) showed that FGDG application can improve sodic-saline soil by improving soil structure and enhance salt leaching process; and by altering soluble salt components, increasing ion exchange capacity, and regulating soil pH (Liu et al. Citation1980).
However, due to large variation in soil alkali level between different areas in field conditions, the research results from the field plots may not be accurate. In some cases, the variation in sodic-saline level between plots may be large enough to mask the treatment effects (Doran and Turnbull Citation1997). We hypothesized that using Monolith lysimeter leaching experiment can solve the problem of spatial variability and yield more accurate experimental results on dynamic changes in the physicochemical properties of sodic soils after applying FGDG.
Recent research has showed that FGDG can significantly increase germination rates and crop products (Wang et al. Citation2008; Sloan et al. Citation1999; Nan et al. Citation2016). When FGDG were used in the reclamation of sodic soils, their high content of soluble salts may adversely affect plant growth, as they reduce the osmotic potential of plant roots (Shannon Citation1997). Thus, it is necessary to determine a suitable application rate for FGDG that allows crops to tolerate not only sodicity, but also salinity. Wang et al. (Citation2012) showed that the physical and chemical properties of the reclaimed soils were best when the FGDG were applied at 7.5 t ha−1 by pot experiment. Li et al. (Citation2015) verified that survival rates of woody plants were the highest when the FGDG were applied at 45 and 60 t ha−1 in reclaimed tidal lands in the Yangtze River delta by field experiment. Another report from the field demonstration study in a tidal flat soil in the Yangtze River estuary evaluated that the optimal rate of FGDG was 60 t ha−1(Mao et al. Citation2016). Obviously, the best rate is different in the different regions and different degree of salinization. In the study, it is necessary to elicit the optimal FGDG rate in the condition of heavy sodic-saline soil under drought region.
The objectives of this study were to (i) investigate the dynamic changes of physicochemical properties at different soil layers with FGDG’s improvement of sodic-saline soil by using original soil columns collected from heavily contaminated sodic-saline soil and (ii) to determine optimum rate of FGDG based on responses of soil physicochemical properties and plant growth for the soil improvement. The goal was to provide theoretical basis and technical support to improve sodic-saline soil.
2. Materials and methods
2.1. Undisturbed soil column collection site
The experiment was conducted at the Shanxi Academy of Agricultural Sciences research station, located at Yu-Bao village(49º9ʹN,113º 53ʹE), Tianzhen county, Shanxi province, China. It has semi-arid, temperate continental climate with annual precipitation of 400 mm, and evaporation rate of 2033 mm. The saline accumulates in the soil, resulting in sodic-saline soil formed due to high water table, dry climate, and high evaporation rate at this site. The soil salts mainly consist of Na2CO3 and NaHCO3, resulting in heavily sodic-saline soil. The cultivated horizon (0–0.10 m) of the soil at the site contained 86.04% sand, 12.28% silt, and 1.63% clay. It had an initial pH (1 soil: 5 water) of 10.12 and contained 2.43 g/kg of organic matter, and 0.20 g/kg of total nitrogen. The other basic physical and chemical properties of the soil are listed in .
Table 1. Physical and chemical properties of the soil for this study.
2.2. Soil column collection method and experimental design
An area with flat, undisturbed sodic-saline soil was carefully selected for soil column collection in 24 April 2014. Undisturbed soil column at 40-cm deep was collected using a PVC tube (25 cm diam., 50-cm long; A). At first, soil cross section at 1-m deep was prepared. The PVC tube was pushed slowly with uniform force to be inserted into the soil with the sharp end toward the soil. After reaching 40-cm deep, the PVC tube was removed after the soil around the PVC tube was removed with proper tools. Milted Vaseline was pour into the space between soil column and the PVC tube to present leaking of irrigated water and have accurate leaching effect. After the Vaseline became solid, the PVC tube was placed a plastic supporter filled with a thin layer of arenaceous quartz with a piece of nylon cloth on the top. This design was to allow leachates to drain into leachate collection tube smoothly and also prevent soil from entering into the leachate collection tube (B). The whole PVC tube with undisturbed soil column along with the supporter and collection tube was placed in the soil (40-cm deep) under a rainout shelter of 2.5 m tall. The rainout shelter received sunlight and had normal air circulation inside (C). A small pump was used to collect leachate through a pipe of thin tubing connecting the leachate collection tube.
Four treatments were applied as follows:
CK (water irrigation control);
T1 (FGDG 75 g per column (15 t ha−1); water irrigation);
T2 (FGDG 150 g per column (30 t ha−1); water irrigation);
T3 (FGDG 300 g per column (60 t ha−1); water irrigation).
There were nine replicates for each treatment. The FGDG was mixed with the soil at 10 cm deep to minimize disturbing of the soil column. The experiment lasted for 4 months (May through August). The irrigation schedule is listed in .
Table 2. Schedule of watering and sampling.
The purpose of using a larger amount of irrigation water at first time relative the following irrigation time was to mimic the process of leaching soil salt with enough water under field conditions. The irrigation rate after first irrigation was higher enough to allow soil moisture close to field capacity. After the first irrigating, the top of PVC tube was sealed with plastic film to prevent water loss via evaporation for keeping the process of leaching soil salt. From the second irrigating, the plastic film was no longer used. The Na+ content in the leachate was determined. Soil samples were collected at various depths of soil column (0–10, 10–20, 20–30, and 30–40 cm). Soil bulk density, saturated hydraulic conductivity, pH, electrical conductivity (EC), water-soluble cations, ions as well as exchangeable Na+, Ca2+, Mg2+, and K+ were measured. The ESP is calculated as follows:
where Naexch+, Caexch2+, Mgexch2+, and Kexch+ are the amounts of exchangeable Na+, Ca2+, Mg2+, and K+ in soil (cmol kg−1).
On 13 June 2014, 10 seeds of maize (cv. Yayu 8) were planted in each soil column, emergence date, plant height, and weight was measured at the day 90.
2.3. Analysis of soil samples
The samples were air-dried and passed through a 1-mm sieve. The EC, pH, soluble anions, and soluble cations were measured using 1:5 water extracts. The soluble cations were measured using an atomic absorption spectrophotometer, soluble anions were determined by anion chromatography, and exchangeable cations were determined in 1 M ammonium acetate (pH = 7) extract. Following this extraction and washing with 96% alcohol, the cation exchange capacity was determined by the removal of ammonium ions by distillation (Rhodes Citation1982). Na and K were determined by flame emission spectroscopy in the extract, and Ca and Mg were determined by atomic absorption spectrophotometer. Soil pH was determined using the glass electrode method.
Soil bulk density was determined using a soil core (stainless steel cylinder with a volume of 100 cm3) (Blake and Hartge Citation1986). After the intact samples were brought to the laboratory, they were dried at 105°C.
Saturated hydraulic conductivity was measured using a downward flow experiment with constant-head (Jury and Horton Citation2004). The soil samples collected from undisturbed soil column with a circular soil cutter were soaked for 24 h. After removing the cover, an empty circular soil cutter was connected with the cutter containing soil column, and a beaker (100 mL) was placed under the cutter and soil column. Water was added into the empty circular soil cutter to reach 5 mm deep (1 mm below the edge of the cutter). The volume of leachate was measured every 10 or 20 min from the time when first drop of leachate occurred, and water was added to the original level each time when the leachate was measured. Water temperature was measured. The process stopped when the amount of leachate remained same over each time interval.
The saturated hydraulic conductivity is calculated according to Darcy’s law:
where Kt represents saturated hydraulic conductivity (cm·h−1), Q represents volume of leachate mL), S is area of cross section for leaching(cm2), t is duration of leaching(min), L is the length of soil column (cm), and H is water thickness(cm).
In order to eliminate the effects of temperature, the saturated hydraulic conductivity calculated above is converted into the one under 10°C using the following equation:
where Kt represents saturated hydraulic conductivity under certain water temperature cm·h−1), K10 represents saturated hydraulic conductivity at 10°C (cm·h−1), and T is water temperature (°C).
2.4. Data analysis
The Minitab v. 16.0 software package was used for all statistical analyses. One-way ANOVA was used to compare the soil physical and chemical properties among the treatments. All statistical analyses were conducted at the 5% level of significance. The SigmaPlot v.12.0 software package was used for all graphs.
3. Results
3.1. Effects of FGDG on physical properties of the heavily sodic-saline soil
As FGDG rate increased, soil bulk density declined at top soil layer (0–10 cm) and middle soil layer (10–20 cm), but increased at bottom soil layer (20–40 cm) (). FGDG applied at 15, 30, and 60 t ha−1 reduced soil bulk density from original 1.72 g cm−1 to 1.47, 1.41, and 1.31 g cm−3, respectively, at 0–10 cm soil layer; similarly, the three treatments reduced soil bulk density from the original 1.64 to 1.56, 1.60, and 1.59 g cm−3, respectively, at 10–20 cm soil layer. However, the soil bulk density was increased from the original value of 1.62 to 1.63, 1.63, and 1.66 g cm−3, respectively, at 20–40 cm soil layer due to the FGDG application at 15, 30, and 60 t ha−1.
Figure 2. Soil bulk density and SHS as affected by different FGDG treatments at different sampling depth.
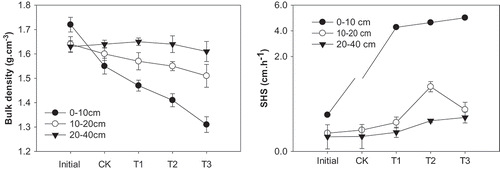
FGDG treatments at 15, 30, and 60 t ha−1 increased saturated hydraulic conductivity by 45.5-fold, 47.0-fold, and 49.2-fold relative to the original value at top soil layer (0–10 cm) (). At middle soil layer (10–20 cm), the three FGDG treatments increased saturated hydraulic conductivity by 0.6-fold, 2.5-fold, and 2.0-fold, respectively, as compared with the original value. At the bottom soil layer (20–40 cm), the three FGDG treatments increased the saturated hydraulic conductivity by 0.5-fold, 0.8-fold, and 1.3-fold, respectively, relative to the original value. The FGDG greatly increased saturated hydraulic conductivity at top soil layer, and the effect of FGDG declined as soil depth increased.
3.2. Effects of FGDG on chemical properties of the heavily sodic-saline soil
3.3. pH and EC
Compared with the control, the application of FGDG can reduce pH significantly (p < 0.05) at top (0–10 cm) and middle soil layer (10–20 cm), especially at the top soil layer with the greatest decrease of pH value (). The pH value at top and middle soil layer declined sharply from day 0 to day 30, and then slowly from day 30 to day 90. The FGDG treatments at 15, 30, and 60 t ha−1 reduced pH from the original value of 10.12 (day 0) to 8.55, 8.42, and 8.27 as measured at day 90 at top soil layer. No obvious change in soil pH was found for the control during the 90 days. In addition, FGDG did not impact soil pH at bottom soil layers (20–30 and 30–40 cm deep).
Application of FGDG reduced EC compared with initial value (). At the top soil layer, EC value declined, then increased, and then declined during the period of 90days in responses to FGDG treatments, with FGDG at 60 t ha−1 having the greatest changes. All FGDG treatments had higher EC than the control. For the other soil layers (10–20, 20–30, and 30–40 cm), FGDG tended to decline from day 0 to day 30, and leveled off from day 30 to day 90 during which no difference in EC was found between the FGDG treatments and control. Overall, as leaching effects of irrigation, compared with initial value, the soil EC decreased with FGDG application except T3 (60 t ha−1) in 30d and 60d reduced.
3.3.1. Effects of FGDG on exchangeable Na± and ESP in the heavily sodic-saline soil
The FGDG treatments reduced soil exchangeable Na+ content at top (0–10 cm) and middle (10–20 cm) soil layers, with the exchangeable Na+ declining the most at the top soil layer (0–10 cm) (). The FGDG treatments at 15, 30, and 60 t ha−1 reduced exchangeable Na+ from the original value (day 0) to 1.2, 1.0, and 0.6 cmol kg−1. As FGDG rate increased, greater decline in the exchangeable Na+ content at the top soil layer (0–10 cm) was found. For the 20–30 cm soil layer, the exchangeable Na+ content at day 90 was higher than that at day 0, with higher value in FGDG treatments relative to the control. However, at the 30–40 cm soil layer, no difference in exchangeable Na+ was found between treatments and the control.
Figure 5. Soil Ex-Na+ as affected by different FGDG treatments at different sampling depth and dates.
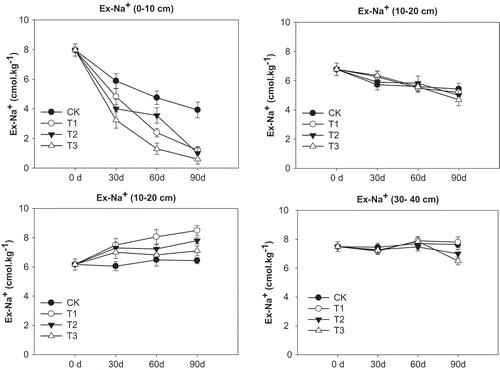
Effects of FGDG on ESP varied depending on soil depth and sampling time (). The FGDG treatments reduced soil ESP at the two soil layers (0–10 and 10–20 cm). As sampling time progressed, the FGDG effects on ESP at the top soil layer (0–10 cm) increased. At day 90, the FGDG treatments at 15, 30, and 60 t ha−1 reduced ESP from the original value of 52% (day 0) to 17%, 13%, and 8%, respectively. As the rate increased, the FGDG effects on ESP increased. No significant effect of FGDG on ESP was found for the bottom soil layers (20–30 and 30–40 cm).
3.3.2. Effects of FGDG on water-soluble Na± and Na± in leachate of the heavily sodic-saline soil
Water-soluble Na+ content was monitored at different soil layers and different dates. As FGDG increased, the soil water-soluble Na+ content tended to decline relative to the control at the three soil layers (0–10, 10–20, and 20–30 cm), especially at day 90 when the FGDG treatments at 30 and 60 t ha−1 had less water-soluble Na+ at the three soil layers (), but no difference was found between treatments of 30 and 60 t ha−1. Otherwise, it was monitored that water-soluble Na+ content was higher at the 30–40 cm soil layers than at the three soil layers (0–10, 10–20, and 20–30 cm) in the three sampling dates, especially FGDG treatments at 60 t ha−1. This phenomenon indicates that water-soluble Na+ tends to accumulate at this layer. It can cause poor salt leaching.
Table 3. Changes of water-soluble Na+ content in soil and amount of Na+ from leachate.
Total salt content from leachate was higher from the soil treated with FGDG relative to non-treated control as measured at day 30, 60, and 90 (). Total Na+ salt from leachate was 15.900 g for the control, 19.812 g for FGDG at 15 t ha−1, 22.231 g for FGDG at 30 t ha−1, and 18.382 g at 60 t ha−1, respectively. The FGDG rate at 30 t ha−1 has the greatest leaching effect on Na+ salt.
3.4. Effects of FGDG on maize growth
At day 90, the rate of emergence, height, shoot, and root weight of maize were investigated (). Significant differences (p < 0.05) were observed between FGDG treatments and control. When compared among the different FGDG treatments, the values of these properties at 30 t ha−1 were the highest.
Table 4. Effects of FGDG on maize growth.
4. Discussion
4.1. Mechanism of FGDG’s effect on sodic-saline soil improvement
Calcium sulfate is the major component of FGDG. The mechanism of improving sodic-saline soil by FGDG is to replace Na+ on the soil colloids with Ca2+ from calcium sulfate in FGDG (US Salinity Laboratory Staff Citation1954). Thus, we have
The sodic-saline soil contains an excess of exchangeable Na+ on soil colloids, which is the major factor contributing to soil alkali (Hu et al. Citation2005). The Na+ has high degree of hydration and great dispersity; it is bound onto the surface of soil colloids and distributed in the space between soil particles, resulting in formation of dense, impermeable hardened soil layer (Suarez et al. Citation1984; Gupta and Abrol, Citation1990). The calcium cation from FGDG can replace Na+ on the surface of sodic-saline soil colloids, reducing soil exchangeable Na+ and then soil ESP (Quirk and Schofield Citation1955; Mcintyre Citation1979; Ilyas et al. Citation1997; Fares et al. Citation2000; Chi and Wang Citation2014). After the Ca2+ from FGDG is exchanged with Na+ from soil colloids, the outside layer of soil colloids occupied with Ca2+ no longer absorbs water molecule, and the colloid particles are able to get closer to each other, forming particle groups. When water enters into the space between particle groups, it cause particle group to expand. After drying, the particle groups shrink, causing soil cracking. As the process goes on, the soil aggregate structure is formed, resulting in loose soil, lower bulk density higher saturated hydraulic conductivity, which promote leaching spend of soil salts and eventually improves sodic-saline soil (Wu et al. Citation2000; Chen et al. Citation2004). Results in the experiment presented here showed that applying FGDG led to a decrease in soil bulk density and increase in saturated hydraulic conductivity.
4.2. Leaching is a key process for sodic-saline soil improvement by FGDG
Reclamation of sodic-saline soils requires removal of Na+ from the colloid’s cation exchange sites, usually by gypsum and leaching of the replaced Na+ out of the root zone in percolating water (Ilyas, M et al. Citation1997). The exchange reaction between gypsum and the soil’s exchangeable Na+ depends on the contact of gypsum with soil particles and the rate of removal of Na+ from the soil solution. Mixing of gypsum and fast removal of Na+ from the soil solution speeds the exchange process (Frenkel et al. Citation1989). The generated water-soluble Na+ leached downward through the soil when leaching occurred (Chun et al. Citation2001). The results of this study indicated that application of FGDG increased EC at the top soil layer in a short period of time after treatment. This may be caused by several factors. On one hand, total salt content may increase due to low solubility of CaSO4 (only 2 g L−1 at 25°C) (Wang et al. Citation2013). On the other hand, soluble Ca2+ may be exchanged with Na+ on soil colloids, resulting in accumulation of Na+ in soil solution. Because the excessive Na+ in the top soil layer is harmful to plant growth, the irrigation must be conducted with the application of FGDG (Wang et al. Citation2012). In this way, the Na+ on the top soil layer can be moved into deeper soil layer through leaching, eventually reducing EC in the top soil layer.
4.3. Optimum FGDG rate for heavily contaminated sodic-saline soil
Excess FGDG may result in accumulation of toxic ions in the deep soil layer and increase exchangeable Na+ and ESP, negatively, reduce positive effects of FGDG on sodic-saline soil improvement. Therefore, it is important to use proper rate of FGDG. Chen et al. (Citation2005) applied FGDG at 20.91, 30.62, and 50.33 t ha−1 in the light, medium, and heavy alkali soil, respectively. Xiao et al. (Citation2010) conducted a study aimed at reducing soil alkali level using undisturbed soil column and identified the optimum theoretical FGDG rates were 12, 53, 17.25, and 23.56 t ha−1 for light, medium, and heavily alkali soil, respectively. Although the formation process of heavily sodic-saline soil at Datong valley where the soil was collected for this study was complex and diversity, Na+ from soil colloids and soil solution was the most important factor leading to soil salinity. The objective of improving heavily sodic-saline soil was to reduce the Na+ content from soil colloid and soil solution by using optimum rate of FGDG. In this experiment, it can be concluded that FGDG rate at 30 t ha−1 may be suitable based on the change of water-soluble Na+ in the soil column and the amount of Na+ salt from leachate, and considering the other parameters including soil alkali level, soil bulk density, saturated hydraulic conductivity and maize growth. This rate was only from the experiment of soil column. Further field research is needed to confirm this conclusion.
5. Conclusion
This study demonstrated that the FGDG has a significant effect on soil physical, chemical properties, and plant growth in the top soil layer for the heavily sodic-saline soil. The effects became greater as the FGDG rate increased. Leaching through frequent irrigation is needed to wash the salt ions into deep soil layer because the FGDG may increase EC value in the top soil layer in a short period of time. The optimum FGDG rate of 30 t ha−1 gave the best results, which can obtain the best leaching of emergence rate of maize reached to 92%.
Acknowledgments
This research was supported by China’s International Science and Technology Cooperation Fund (2015DFA90990). The authors thank Prof. Russell Yost from Department of Tropical Plant and Soil Sciences, University of Hawaii at Manoa for his checking of the English language.
Additional information
Funding
References
- Blake GR, Hartge KH 1986: Bulk density. In Methods of Soil Analysis. Part I. Physical and Mineralogical Methods, Ed. Klute A, pp. 363–382. American Society of Agronomy, Madison.
- Chen FS, Zeng DH, Wang GR 2004: Ameliorative effects of peat and weathered coal on saline-alkali soil. J. Liaoning Tech. Univ., 23(6): 861–864.
- Chen H, Wang SJ, Chen CH 2005: The application and effect of desulphurized waste of flue gas in improving alkali soil. Agric. Res. Arid Areas, 23(4): 38–42.
- Chi CM, Wang ZC 2014: Saturated hydraulic conductivity and its influence factors of saline-alkali soils. Chin. J. Soil Sci., 45(3): 601–607.
- Chun S, Nishiyama M, Matsumoto S 2001: Sodic soils reclaimed with by-product from the flue gas desulfurization: corn production and soil quality. Environ. Pollut., 114, 453–459. doi:10.1016/S0269-7491(00)00226-8
- Clark RE, Zetosk RK 1997: Growth of forages on acid soil amended with flue gas de-sulfurization by products. Fuel, 76(8): 771–775. doi:10.1016/S0016-2361(97)00004-5
- Crews JT, Dick WA 1998: Liming acid forest soils with flue gas desulfurization by product growth of northern red oak and leach ate water quality. Environ. Pollut., 103(1): 55–61. doi:10.1016/S0269-7491(98)00137-7
- Doran JC, Turnbull JW 1997: Australian trees and shrubs:species for land rehabilitation and farm planting in the tropics [R]. Canberra:Australian Centre for International Agricultural Research. Environ. Sci., 10(1): 23–26.
- Fares A, Alva AK, Nkedi-Kizza P, Elrashidi MA 2000: Estimation of soil hydraulic properties of a sandy soil using capacitance probes and guelph permeameter. Soil Sci., 165(10): 768–777. doi:10.1097/00010694-200010000-00002
- Frenkel H, Gerstle Z, Alperovitch N 1989: Exchange-induced dissolution of gypsum and the reclamation of sodic soils. J. Soil Sci., 40, 599–611. doi:10.1111/j.1365-2389.1989.tb01301.x
- Gupta RJ, Abol IP 1990: Salt-affected soils: their reclamation and management for crop production. In Advances in Soil Science: soil Degradation. Advances in Soil Science, Eds. Lal R, Steward BA, Vol. 11, pp. 223–288. Springer-Verlag.
- Hu W, Shao MA, Wang QJ, Li Y 2005: Effects of sampling size on measurements of soil saturated hydraulic conductivity. Acta Pedologica Sinica, 42(6): 1041–1044.
- Ilyas M, Qureshi RH, Qadir MA 1997: Chemical changes in a saline-sodic soil after gypsum application and cropping. Soil Technol., 10, 247–260. doi:10.1016/S0933-3630(96)00121-3
- Jenny H 1988: Origin and Characters of Soil Resources, pp. 394–399. Science Press, Beijing.
- Jury WA, Horton H 2004: Soil Physical, 6th ed. Wiley, Hoboken, NJ.
- Kelly WP 1959: Saline and Alkaline Soil, pp. 2–10. Science Press, Beijing.
- Li B, Wang ZC, Sun ZG, Chen Y, Yang F 2005a: Resources and sustainable resource exploitation of salinized land in China. Agric. Res. Arid Areas, 23(2): 154–158.
- Li XN, Zhang Q, Chen MC, Zhang H 2005b: Study on effect of using three soil conditioners to phosphorus validity of soda-alkali Soil. J. Soil Water Conserv., 19(1): 71–74.
- Li XP, Mao YM, Liu XC 2015: Flue gas desulfurization gypsum application for enhancing the desalination of reclaimed tidal lands. Ecol. Eng., 82, 566–570. doi:10.1016/j.ecoleng.2015.04.010
- Li YJ, Miao QW, Chen CH, Xu XC, Wang SJ 2006: Influence of sodication and chemical improvement on soil aggregate. J. Arid Land Resour. Environ., 20(1): 136–139.
- Liu LP, Long XH, Shao HB, Liu ZP, Tao Y, Zhou QS, Zong JQ 2015: Ameliorants improve saline–alkaline soils on a large scale in northern Jiangsu Province, China. Ecol. Eng., 81, 328–334. doi:10.1016/j.ecoleng.2015.04.032
- Liu QS, Qiu FQ, Li FZ, Zhou HM, Chen EF 1980: Studies on the transformation of soil organic materials. Part I. Transformation of organic materials in relation to the amelioration of soda-saline soils. Acta Pedologica Sinica, 17(3): 16–19.
- Mao YM, Li XP, Dick WA, Chen LM 2016: Remediation of saline–sodic soil with flue gas desulfurization gypsum in a reclaimed tidal flat of southeast China. J. Environ. Sci., 45, 224–232. doi:10.1016/j.jes.2016.01.006
- Mcintyre DS 1979: Exchangeable sodium, subplasticity and hydraulic conductivity of some Australian soils. Aust. J. Soil Res., 17, 115–120. doi:10.1071/SR9790115
- Nan JK, Chen XM, Wang XY, Lashari MS, Wang YM, Guo ZC, Du ZJ 2016: Effects of applying flue gas desulfurization gypsum and humic acid on soil physicochemical properties and rapeseed yield of a saline-sodic cropland in the eastern coastal area of China. J. Soils Sediments, 16, 38–50. doi:10.1007/s11368-015-1186-3
- Quirk JP, Schofield RK 1955: The effect of electrolyte concentration on soil permeability. J. Soil Sci.,. 6(2): 163–178. doi:10.1111/(ISSN)1365-2389a
- Rhodes DR 1982: Cation exchange capacity. In Methods of Soil Analysis Part 2. Chemical Methods, Eds. Page AL, Miller RH, Keeney DR, pp. 149–165. America Society of Agronomy, Madison, Wisconsin.
- Shannon MC 1997: Adaptation of plants to salinity. In Advances in Soil Science, Eds. Lal R, Steward BA, pp. 75–120. Springer-Verlag.
- Sloan JJ, Dowdy RH, Dolan MS, Rehm GW 1999: Plant and soil responses to field-applied flue gas desulphurization residue. Fuel, 78, 169–174. doi:10.1016/S0016-2361(98)00135-5
- Suarez DL, Rhoades JD, Lavado R, Griee CM 1984: Effect of pH on saturated hydraulic conductivity and soil dispersion. Soil Sci. Soc. America J., 48, 50–55. doi:10.2136/sssaj1984.03615995004800010009x
- Sun YY, Zhang J 2016: Development and APPLICATION of Key Technology and Equipment for Comprehensive Utilization of FGDG. Yearbook of Zhong Guancun, pp. 327–328. Beijing Press, Beijing.
- US Salinity Laboratory Staff 1954: Diagnosis And Improvement of Saline and Alkaline Soils. US Department of Agriculture Handbook 60, US Government Printing Office, Washington, DC.
- Wang JM, Bai ZK, Yang PL 2012: Sodic soil properties and sunflower growth as affected by byproducts of flue gas desulfurization. Plos One, 7(12): e52437. doi:10.1371/journal.pone.0052437
- Wang JM, Bai ZK, Yang PL 2013: Effect of byproducts of flue gas desulfurization on the soluble salts composition and chemical properties of sodic soils. Plos One, 8(8): e71011. doi:10.1371/journal.pone.0071011
- Wang JM, Yang PL, Zhang JG, Shi Y 2005: Salinity effect on sunflower at seedling stage during improving sodic soils reclaimed with by-product from flue gas desulphurization (BFGD). Trans. CSAE, 21(9): 33–37.
- Wang SJ, Chen CH, Xu XC, Li YJ 2008: Amelioration of alkali soil using flue gas desulfurization byproducts: productivity and environmental quality. Environ. Pollut., 151, 200–204. doi:10.1016/j.envpol.2007.02.014
- Wang ZQ 1993: Salt Affected Soils of China, pp. 400–515. Science Press, Beijing.
- Wu LH, Wang GR, Zhang CX, Yasushi N, Yamaguchi. T 2000: Ecological effect of Peat and weathered coal on alkali-saline soil. Eco-Agriculture Res., 8(2): 34–37.
- Xiao GJ, Luo CK, Zhang FJ, Wang B, Zheng GQ, Yang J, Mao GL, Bai HB 2010: Application amount of desulfurized gypsum from coal fired power plants on improving the quality of alkalized soil. Res. Environ. Sci., 23(6): 762–767.
- Xu SG, Li SY, Liao XR, Lan PL 2001: Study on the use of desulphurisation products in planting peanut in a pot-experiment. Soil Environ. Sci., 10(1): 23–26.
- Yin HN, Bai HX, Zheng YS, Jin FH, Xi QT, Yasushi N, Yamaguchi. T 1998: Effect of applying peat to soda salinized soil on soil salinity in north plain of Liaohe River. Chin. J. Appl. Ecol., 9(5): 491–495.
- Yu RP, Chen DM 1999: Resources and exploitation of salinized land in China. Chin. J. Soil Sci., 30(4): 158–159.
- Zhang HJ, Li YJ, Chen CH, Xu XC, Wang SJ 2007: Research on the Eigen value’ s Change in the Process of improving alkaline soil with desulfurized gypsum. J. Arid Land Resour. Environ., 21(7): 165–168.
- Zhang JF 2004: Agroforestry and its application in amelioration of saline soils in eastern China coastal. Forestry Study in China, 6(2): 27–33. doi:10.1007/s11632-004-0016-2
- Zhao JH, Li Y, Wu LG, Li YJ, Che HZ 2006: Effect of gypsum on improving basified soil (Ⅱ)-Columns of mixture of gypsum with soil from top 20 cm. J. Yangtze Univ., 3(1): 119–122.