ABSTRACT
Herbs of the Labiatae have relatively low salt tolerance. They are widely grown in drylands, but salt stress there is a typical problem and may reduce yields. To examine their salt tolerance mechanisms, we grew basil, sage, thyme, and oregano in nutrient solution containing 50 mM NaCl and determined the biomass; contents of Na, K, and Mg in leaf blades, stems, and roots; contents of total chlorophyll, malondialdehyde (MDA), hydrogen peroxide in leaf blades; and activities of antioxidative enzymes in leaf blades. The salt tolerance decreased in the order of basil ≈ sage > thyme > oregano. The good salt tolerance of basil was explained by a significant increase in the activity of catalase, in addition to the low Na/K ratio of leaf blades due to the retention of Na in stems and roots and of K in leaf blades. The good salt tolerance of sage was explained by the low Na/K ratio in leaf blades and the prevention of lipid peroxidation by high antioxidative enzyme activities, despite its poorer management of nutrient uptake. In thyme, although catalase activity increased significantly to alleviate salt-induced oxidative stress caused by Na influx into all parts, low K and Mg allowed shoot weight in particular to decrease. In oregano, antioxidative responses appeared as significant increases in ascorbate peroxidase and glutathione reductase activity, and K was accumulated in leaf blades, but serious salt-induced oxidative stress caused by high Na influx into all parts reduced the growth of all parts. These results show that despite similar responses among species, salt tolerance is not necessarily the same. In this experiment, we revealed the salt tolerance mechanism of each of four Labiatae herbs by revealing their strengths and weaknesses in nutrient uptake and antioxidative responses.
1. Introduction
Around the world, soil salinity severely limits agricultural production. In drylands, damage is exacerbated by excessive irrigation and by salinization of groundwater (Siyal et al. Citation2002), and the affected area is likely to increase. Many medicinal and culinary herbs are grown in drylands, but salinity causes yield losses (Said-Al Ahl and Omer Citation2011). There are more than 3000 species of herbs in the Labiatae (Lamiaceae) family (Raja Citation2012), with a range of salt tolerances (Wu et al. Citation2016). To select tolerant cultivars that can be grown in salt-affected soil and to develop genetically modified forms, we must elucidate the salt tolerance mechanisms used.
In general, salt stress arises from osmotic stress and ionic stress, which cause secondary oxidative stress, with a huge influence on plant growth. Normally, salt first causes water deficit and then disturbance of ionic homeostasis through the inhibition of physiologically important enzymatic reactions (Parida and Das Citation2005). The Na/K ratio is an important indicator of salt tolerance (Shabala and Cuin Citation2007); some salt-tolerant plants limit Na+ transport into leaves and selectively take up K+, allowing physiological processes to remain functional at a lower Na/K ratio (Hasanuzzaman et al. Citation2014). However, when Na+ starts to disturb photosynthetic reactions, electron transfer in the chloroplast thylakoid membrane is inhibited; the surplus electrons are passed to oxygen molecules, and reactive oxygen species (ROS) such as superoxide anion (O2−) in photosystem I (PSI) and singlet oxygen (1O2) in PSII are produced. If these ROS are not rapidly eliminated and instead accumulate, plant growth is remarkably reduced (Foyer and Noctor Citation2000).
In general, plants use antioxidative enzymes and antioxidants to remove ROS (Gill and Tuteja Citation2010). Among the main antioxidative enzymes, superoxide dismutase (SOD) converts O2− into hydrogen peroxide (H2O2); ascorbate peroxidase (APX) and catalase (CAT) detoxify H2O2 to water; and glutathione reductase (GR) catalyzes the reduction from glutathione disulfide (GSSG) to the sulfhydryl form glutathione (GSH). APX and GR can operate in conjunction with antioxidants, such as ascorbic acid and glutathione, respectively, and play an important role in a high-efficiency ROS removal system called the water–water cycle (Asada Citation2006).
Antioxidative capacity differs markedly among plant species. In the Labiatae, some herbs, such as basil (Ocimum basilicum L.), sage (Salvia officinalis L.), thyme (Thymus vulgaris L.), and oregano (Origanum vulgare L.), have high antioxidative capacity (Dumbravă et al. Citation2012; Roby et al. Citation2012). This capacity provides defense against various environmental stresses such as salinity, drought, and heat, and thus plants with high antioxidative capacity are likely to be stress tolerant (Gill and Tuteja Citation2010).
Basil can tolerate saline soil (Sahay and Patra Citation2014), but sage is moderately salt sensitive (Mouna et al. Citation2009). Under salt stress, sage directs its photosynthates away from biomass production toward responses to salt stress, increasing the production of essential oils as secondary metabolites and the production of fatty acids (Taarit et al. Citation2009). Salt treatment reduced the biomass of thyme by almost half, possibly by provoking increased C and N contents in amino acids in roots as a stress response (Cordovilla et al. Citation2016). While irrigation with saline water (4 dS m−1) significantly reduced the growth of oregano, increasing the available soil moisture and supplying potassium humate in irrigation water improved growth (Said-Al Ahl and Hussein Citation2010). On the other hand, a salt-tolerant cultivar of marjoram (Origanum majorana L.) had high potassium selectivity and antioxidative enzyme activity and showed morphological adaptations to salt (Baâtour et al. Citation2012).
Antioxidative reactions and nutrient uptake can differ among species within the same family. This makes it difficult to predict which species are sensitive and which are tolerant to salt. As there is little information about antioxidative reactions and nutrient uptake under salt stress among members of the Labiatae, it is necessary to test the salt responses of different species. We grew basil, sage, thyme, and oregano to elucidate their salt tolerance mechanisms.
2. Materials and methods
2.1. Cultivation and sampling
Basil, sage, thyme, and oregano were grown in a glasshouse of the Faculty of Agriculture, Tottori University (35°51′52″N, 134°17′36″E), from 9 April to 1 July 2015. Seeds were sown and germinated in plastic containers with a mesh base (47 cm L × 33 cm W × 8 cm D) filled with moistened vermiculite. After a few weeks, the seedlings were transplanted into plastic containers (58 cm L × 37 cm W × 15 cm D) filled with 30 L of standard nutrient solution and were grown for 30 days (basil and sage) or 28 days (thyme and oregano). The nutrient solution contained the following (mM): [NH4+] 0.55, [NO3−] 1.1, [K+] 0.5, [Ca2+] 0.375, [Mg2+] 0.5, [PO43−] 0.15, [Mn2+] 4.55 × 10–3, [Mo6+] 2.6 × 10–4, [B3−] 0.95 × 10–2, [Zn2+] 0.75 × 10–3, [Cu2+] 0.8 × 10–4, [EDTA-Fe] 1.8 × 10–2, supplied as (NH4)2SO4, KH2PO4, KNO3, Ca(NO3)2·4H2O, MgSO4·7H2O, MnSO4·5H2O, (NH4)6Mo7O24·4H2O, H3BO3, ZnSO4·7H2O, and CuSO4·5H2O. The pH was adjusted to 6.0–6.5. All water was tap water, with ~8.6 ppm Na+ (0.37 mM Na+).
After initial growth, 20 plants of each species were retained in the standard nutrient solution as controls, and another 20 received salinity treatment in the standard nutrient solution plus 50 mM NaCl. The plants were grown for 13 days (basil and sage), 14 days (thyme), or 7 days (oregano). The oregano was harvested early as it appeared not to grow. All experiments were conducted with four replications. After harvest, eight plants of each species were randomly selected from each treatment, divided into leaf blades, stems + petioles, and roots, weighed fresh (FW), dried in an air-forced oven at 70°C for 72 h, and weighed dry (DW). From the other 12 plants, a few leaves were randomly harvested for the determination of chlorophyll content, and the rest of the leaves were immediately frozen in liquid nitrogen and stored at −80°C until use for physiological analysis.
2.2. Leaf water content
Leaf water content was calculated as (FW – DW)/DW.
2.3. Chlorophyll a + b content
To determine the chlorophyll content of the leaf blades, we homogenized about 0.2 g of fresh sample using a mortar and pestle in 1 mL of 99.5% (v v−1) ethanol with some quartz sand. The extracts were filtered through filter paper (No. 5C) and made up to 50 mL with ethanol. Absorbance was measured at 665 and 649 nm with a spectrophotometer (U-1800, Hitachi, Tokyo, Japan), and chlorophyll (a + b) content was calculated according to the equation of Wintermans and De Mots (Citation1965).
2.4. Nutrient contents
Nutrient contents were determined by the wet digestion method. Dried powered samples (~0.2 g) were put into a flask with 15 mL of acid mixture (1 vol. sulfuric acid: 10 vol. nitric acid: 4 vol. perchloric acid) and digested at 350°C. The contents of potassium (K), sodium (Na), and magnesium (Mg) were determined by atomic absorption spectrophotometer (Z-2310, Hitachi, Tokyo, Japan).
2.5. Leaf MDA content
The content of MDA, an indicator of lipid peroxidation, in leaf blades was measured as 2-thiobarbituric acid–reactive substances according to Ghanem et al. (Citation2008). The MDA content was calculated by subtracting the non-specific absorbance (600 nm) from the specific absorbance (532 nm) of the reactive substances and using the molar extinction coefficient (155 mM−1 cm−1).
2.6. Leaf hydrogen peroxide content
The H2O2 content in leaf blades was measured according to Ferguson et al. (Citation1983) with slight modifications. The frozen leaf blades (0.5 g) were homogenized in 5 mL of cold acetone using a mortar and pestle; 2 mL of the extract was centrifuged (4°C, 3000g, 10 min), and 1 mL of supernatant was placed in another tube with 0.23 mL of 17 M (28%) ammonia solution and 0.1 mL of 20% (v v−1) titanium chloride. The solution was centrifuged as before. The supernatant was discarded, and the precipitate was washed with 1 mL of acetone and then centrifuged as before. This process was repeated until the precipitate was completely white. The white precipitate was mixed with 3 mL of 1 M sulfuric acid and left overnight. The following day, the H2O2 content was determined by measuring absorbance at 410 nm. Standards in the range of 0–500 ppm H2O2 were also prepared.
2.7. Crude enzyme extraction
Frozen leaf blades (0.3–0.4 g) were ground using a mortar and pestle in liquid nitrogen and homogenized with 5 mL of 50 mM K-P buffer containing 1 mM ascorbic acid (pH 7.8). After centrifugation (4°C, 15 000g, 30 min), the supernatants were assayed for enzyme activity.
2.8. CAT, APX, GR, and SOD activities
We measured CAT, APX, and GR activities in leaf blades according to the method of Tanaka et al. (Citation1982). For measurement of CAT activity, specific absorbance of H2O2 (230 nm) was measured in 1 mL of assay mixture containing 100 mM K-P buffer (pH 7.8), 20 mM H2O2, and 2% (v v−1) crude enzyme extract. CAT activity was determined as the amount of H2O2 scavenged per minute, using an extinction coefficient of 0.04 mM−1 cm−1.
For measurement of APX activity, specific absorbance of l-ascorbic acid (290 nm) was measured in 1 mL of assay mixture containing 100 mM K-P buffer, 0.5 mM l-ascorbic acid, and 2% (v v−1) crude enzyme extract. APX activity was determined as the amount of l-ascorbic acid oxidized per minute, using an extinction coefficient of 2.8 mM−1 cm−1.
For measurement of GR activity, specific absorbance of NADPH (340 nm) was measured in 1 mL of assay mixture containing 50 mM K-P buffer, 0.1 mM EDTA, 0.02 mM reduced NADPH, 0.02 mM oxidized glutathione, and 5% (v v−1) crude enzyme extract. GR activity was determined as the decrement of NADPH per minute, using an extinction coefficient of 6.2 mM−1 cm−1.
SOD activity in leaf blades was measured according to the method of Tanaka and Sugahara (Citation1980). First, 1 mL of crude enzyme extract was dialyzed through a dialysis membrane against 500 mL of 10 mM K-P buffer (changed every 3 h) for 12 h. SOD activity was determined in the dialyzed extracts. The specific absorbance of reduced cytochrome c (550 nm) was measured in 1 mL of assay mixture containing 50 mM K-P buffer, 0.1 mM EDTA, 0.1 mM xanthine, 10 µM cytochrome c, 5% (v v−1) crude enzyme extract, and 1.6 × 10−2 U xanthine oxidase. The rate of increase of absorbance (v) was calculated in relation to the rate of increase when 5% (v v−1) crude enzyme extract was replaced with distilled water (V), where 1 unit was defined as v/V = 0.5, and 1 unit of SOD activity was calculated as V/v − 1.
2.9. Protein assay
Protein content in crude enzyme extracts was measured as described by Bradford (Citation1976). Enzyme activity was calculated from the total protein content.
2.10. Statistical analyses
Data are the means of four replications. Means were compared between control and salt treatments by Student’s t-test at p < 0.05, and among leaf blades, stems, and roots by one-way ANOVA followed by Duncan’s Multiple Range Test at p < 0.05, in SPSS v. 25.0 software (IBM, Armonk, NY, USA).
3. Results
3.1. Dry weight
Salinity treatment did not affect dry weight in basil or sage. It significantly decreased dry weights of leaf blades and stems in thyme, and of all parts in oregano ().
Figure 1. Effect of salinity treatment on dry weight in basil, sage, thyme, and oregano.
Different large alphabetic letters and * indicate significant differences in total dry weight and dry weight in each part between control and salinity treatment in the same species (t-test; p < 0.05). 0 Na; standard nutrient solution (Control), 50 Na; standard nutrient solution containing 50 mM NaCl (Salinity treatment). Error bars in the figure indicate standard errors of four replications.
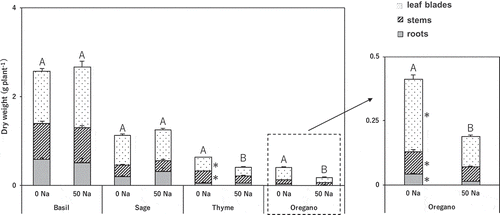
3.2. Leaf water content
Salinity treatment did not affect leaf water content in basil, thyme, or oregano. It slightly but significantly increased leaf water content in sage ().
Figure 2. Effect of salinity treatment on leaf water content in basil, sage, thyme, and oregano.
** indicates significant differences between control and salinity treatment in the same species (t-test; p < 0.01). 0 Na; standard nutrient solution (Control), 50 Na; standard nutrient solution containing 50 mM NaCl (Salinity treatment). Error bars in the figure indicate standard errors of four replications.
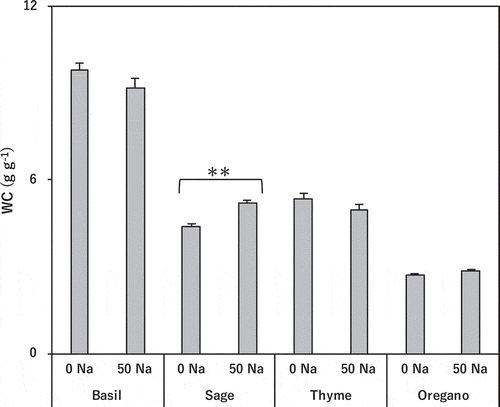
3.3. Leaf chlorophyll a + b content
Salinity treatment did not affect chlorophyll a + b content in basil. It slightly decreased the content in sage, and significantly decreased it in thyme and oregano ().
Figure 3. Effect of salinity treatment on leaf chlorophyll (a + b) content in basil, sage, thyme, and oregano.
** indicates significant difference between control and salinity treatment in the same species (t-test; p < 0.01). 0 Na; standard nutrient solution (Control), 50 Na; standard nutrient solution containing 50 mM NaCl (Salinity treatment). Error bars in the figure indicate standard errors of four replications.
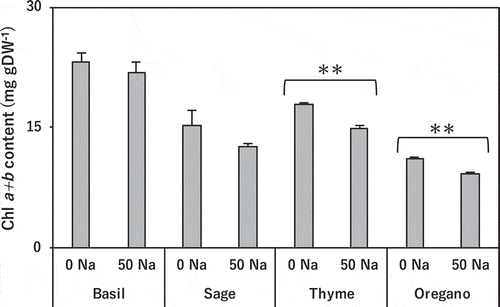
3.4. Leaf MDA content
Salinity treatment did not affect leaf MDA content in basil. It significantly decreased it in sage, slightly increased it in thyme, and significantly increased it in oregano ().
Figure 4. Effect of salinity treatment on leaf MDA content in basil, sage, thyme, and oregano.
* and ** indicate significant differences between control and salinity treatment in the same species (t-test; p < 0.05 and p < 0.01). 0 Na; standard nutrient solution (Control), 50 Na; standard nutrient solution containing 50 mM NaCl (Salinity treatment). Error bars in the figure indicate standard errors of four replications.
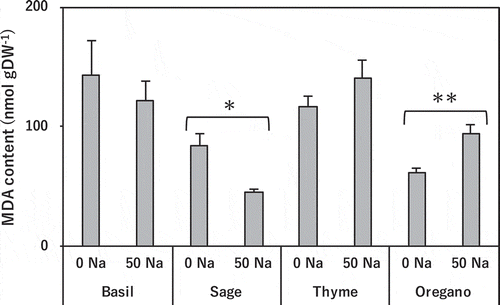
3.5. Leaf H2O2 content
Salinity treatment did not significantly affect leaf H2O2 content in any species, but the content was much higher in sage in both treatments than in the other species ().
Figure 5. Effect of salinity treatment on leaf H2O2 content in basil, sage, thyme, and oregano.
There was no significant difference (t-test; p < 0.05) between control and salinity treatment in the same species. 0 Na; standard nutrient solution (Control), 50 Na; standard nutrient solution containing 50 mM NaCl (Salinity treatment). Error bars in the figure indicate standard errors of four replications.
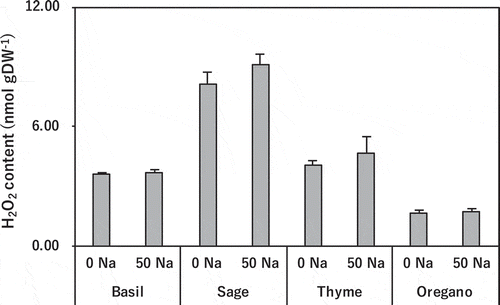
3.6. Leaf antioxidative enzyme activities
Salinity treatment did not affect CAT activity in sage or oregano, but it significantly increased it in basil and thyme (). It did not significantly affect SOD activity in any species, but slightly increased it in sage, thyme, and oregano (). It did not affect APX activity in basil, sage, or thyme, but significantly increased it in oregano (). It did not affect GR activity in basil or thyme, but slightly increased it in sage, and significantly increased it in oregano ().
Figure 6. Effect of salt treatment on leaf antioxidative enzyme activities in basil, sage, thyme, and oregano.
(a) CAT activity, (b) superoxide dismutase activity (SOD), (c) APX activity, (d) GR activity. * and ** indicate significant differences between control and salinity treatment in the same species (t-test; p < 0.05 and p < 0.01). 0 Na; standard nutrient solution (Control), 50 Na; standard nutrient solution containing 50 mM NaCl (Salinity treatment). Error bars in the figure indicate standard errors of four replications.
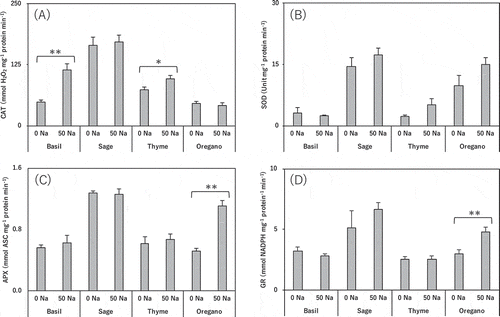
3.7. Nutrient contents
Basil: In the control treatment, the Na content was 10.93 mg g−1 in roots (92.6% of total) and 0.09 mg g−1 in leaf blades (1.5%) (). In the salinity treatment, it increased to 35.74 mg g−1 in stems and 30.10 mg g−1 in roots, but to just 7.03 mg g−1 in leaf blades, representing just 18% of the total, much lower than in the other species. Although the stem and root contents did not differ greatly, the stems had 52.8% of the Na and the roots had 29.2%. On the other hand, the K content was much lower in stems and roots than in leaf blades, and the distribution of K in leaf blades was increased by 30 percentage points relative to the control. Consequently, the Na/K ratio was low in leaf blades and high in roots. And the Mg content was decreased significantly in all parts.
Table 1. Effect of salinity treatment on nutrient contents in leaf blades, stems and roots of basil, sage, thyme, and oregano.
Sage: In the control treatment, the Na content was 7.55 mg g−1 in roots (64.7% of total) and 0.59 mg g−1 (16.2%) in leaf blades (). In the salinity treatment, however, it was greatly increased in both leaf blades (21.63 mg g−1, 51.7%) and roots (27.52 mg g−1, 27.2%). The K content was not changed in the leaf blades, but it was decreased slightly but significantly in the roots, and the distribution showed a tendency to increase in leaf blades and to decrease in stems and roots. The Na/K ratio was lower in leaf blades and higher in roots, as in basil. The Mg content was slightly but significantly decreased in both leaf blades and roots.
Thyme: In the control treatment, the Na content was 3.68 mg g−1 (22.1% of total) in roots and 1.46 mg g−1 (44.1%) in leaf blades (). The distribution was already higher in the leaf blades than in the roots, unlike in basil and sage. In the salinity treatment, however, the Na content was increased in all parts, with no significant difference among the parts, and the distribution was unchanged. On the other hand, the K content was greatly decreased in all parts, unlike in sage, but the distribution did not change between treatments. The Na/K ratio also was not significantly different among the parts. The Mg content was significantly decreased in all parts, particularly in leaf blades.
Oregano: In the control treatment, the Na content was 5.23 mg g−1 (27.6% of total) in roots and 1.89 mg g−1 (53.3%) in leaf blades (). In the salinity treatment, however, it was remarkably increased, especially in stems (67 mg g−1), and was the highest among the four herbs. The distribution was also greatly increased to 43.4% in stems, but lowest at 4.2% in roots. Interestingly, however, the K content nearly doubled in the leaf blades and decreased greatly in the roots. Similarly, the K distribution was also increased in leaf blades and decreased in roots. The Na/K ratio was significantly lower in leaf blades than in stems and roots, and there was no significant difference between stems and roots. The Mg content was significantly decreased in stems but increased in leaf blades.
4. Discussion
We evaluated the salt tolerance of four typical Labiatae herbs in relation to dry matter production and physiological processes. Basil and sage proved tolerant without decreasing dry matter production (). On the other hand, the dry matter production of thyme and oregano decreased greatly. Oregano was the most sensitive species. The leaf water content did not decrease in any species, so the plants did not become dehydrated by osmotic stress ().
First, we evaluated salt tolerance from the aspect of nutrient uptake. In all parts of all four species, the Na content was higher in the salinity treatment than in the control (). Therefore, Na was taken up and transported to where it could cause ionic stress, but the tissue concentrations depended on species.
In basil, the Na content stayed low in leaf blades even under salt stress, and Na accumulated instead in stems and roots (). This result indicates that basil could suppress Na transport into leaf blades or exclude it from leaf photosynthetic tissues, and the stems thus accumulated Na transported from the roots and excluded from the leaf blades. This salt tolerance mechanism was not seen in the other species (). Attia et al. (Citation2009) related Na+ transport to leaf blades with salt sensitivity, and reported that basil could transport Na+ downward from upper young leaf blades. The lower Na/K ratio in leaf blades of basil than in the other species indicates that basil had a superior capacity to transport K to leaf blades ().
In sage also, Na accumulated in roots in the control, but also in leaf blades under salinity, unlike in basil (). The explanation is that its capacity to retain Na in stems and roots was exceeded, and Na was transported to leaf blades. However, the K content was maintained in leaf blades and decreased in roots, suggesting K selectivity in leaf blades ().
On the other hand, thyme and oregano showed salt sensitivity, consistent with a large flow of Na into the shoots, especially in oregano (). It seems that oregano is unable to retain Na in its roots, and instead relies on the stem as a sink, although the Na content in leaf blades was the highest among species. In contrast, however, the K content in leaf blades of oregano nearly doubled in the salinity treatment (). This result suggests active K transport to the leaf blades as a response to salinity to maintain the optimal ion balance in the face of excessive influx of Na (Maathuis and Amtmann Citation1999). In thyme, although salinity treatment did not change the Na distribution (), it greatly increased the Na content in all parts, and the excessive inflow of Na into the photosynthetic parts may have suppressed dry matter production. As only thyme showed a significant decrease in the K content of leaf blades, while the K distribution rate was unchanged, thyme might have experienced K deficiency and been unable to transport K to leaf blades from the other parts.
The leaf chlorophyll contents of the salt-tolerant basil and sage were unchanged, whereas those of the susceptible thyme and oregano were significantly decreased (). This could have been caused by a lack of essential elements such as Mg for synthesizing chlorophyll due to competition with excessive Na in the medium (Gomes et al. Citation2011). Indeed, the Mg content of the leaf blades of thyme was significantly lower (). That in basil and sage also was decreased, though by not as much. In oregano, in contrast, it was significantly increased in the salinity treatment (), so this cannot explain the lower chlorophyll content of oregano, which might instead be explained by the decomposition of photosynthetic pigments by salt-induced oxidative stress.
MDA, an indicator of lipid peroxidation, decreased slightly in basil and significantly in sage, but increased slightly in thyme and significantly in oregano (). These results indicate that the two salt-sensitive species experienced salt-induced oxidative stress damage, as NaCl treatment induced oxidative stress in pea leaf blades, resulting in lipid peroxidation (Hernández and Almansa Citation2002).
H2O2, a typical ROS, can inhibit CO2 fixation (Kaiser Citation1979), and is a stress signaling molecule (Bhattacharjee Citation2005). We measured H2O2 to gauge the generation of ROS. Unexpectedly, its concentration in leaf blades was not significantly increased, even in thyme and oregano (), in which the MDA content rose (). This stability was probably due to maintenance of the balance between the generation and decomposition of H2O2 by the activation of antioxidative enzymes, which decompose H2O2 to H2O. Although the activity of SOD, which produces H2O2, in both species tended to increase in the salinity treatment (), the activities of CAT in thyme and of APX and GR in oregano, which decompose H2O2, were significantly increased (, C, D). Therefore, lipid peroxidation is likely to have been caused by other ROS such as singlet oxygen (1O2), not by H2O2. 1O2 is generated through the decomposition of protein hydroperoxide in PSII (Pathak et al. Citation2017), and is decomposed principally by carotenoids (Ramel et al. Citation2012), not by antioxidative enzymes. 1O2 is more reactive than H2O2 and must be removed quickly to avoid damage. Thus, ROS such as 1O2 may have caused lipid peroxidation and resultant oxidative damage.
Salinity treatment significantly increased the activity of CAT in the salt-tolerant basil (), but there was no change in the other antioxidative enzymes (). Thus, CAT may play an important role in the salt stress response by efficiently removing H2O2, avoiding lipid peroxidation ( and ). In maize, CAT is activated the most in response to salt stress (Azevedo Neto et al. Citation2005). We assume that the activation of CAT in basil also is an important contributor to salt tolerance. On the other hand, sage had higher antioxidative enzyme activities than the other species (), and salinity treatment marginally increased the activities of SOD and GR (), but it did not change the activities of CAT and APX, which decompose H2O2, and the concentration of H2O2 tended to rise. In light of the remarkable decrease of MDA in sage (), this H2O2 might have worked as a signaling molecule (Bhattacharjee Citation2005). Salt-induced oxidative stress in rosemary (Rosmarinus officinalis L., Labiatae) does not cause oxidative damage but might induce defense mechanisms (Tounekti et al. Citation2011). Salt stress significantly increased CAT activity in the salt-sensitive thyme and APX and GR activities in the salt-sensitive oregano (), and marginally increased SOD activity in thyme and oregano (). These results indicate that antioxidative enzymes were activated in both species in response to salt stress. However, as seen in the accumulation of MDA (), salt-induced oxidative stress due to excessive Na inflow to all parts () was more severe, and reduced growth ().
The contributions of nutrient uptake and antioxidative response to salt tolerance varied among the species. The high tolerance of basil was due to aspects of both nutrient uptake and antioxidative capacity. That of sage was due mainly to antioxidative capacity. The salt sensitivity of thyme may have been due to K and Mg deficiency as a result of Na competition, resulting in poor chlorophyll synthesis. That of oregano was due to damage caused by salt-induced oxidation due to excessive Na inflow into leaf blades. The vulnerability of their nutrient uptake ability might have made thyme and oregano sensitive to salt, in spite of their antioxidative enzyme activities.
Additional information
Funding
References
- Asada K 2006: Production and scavenging of reactive oxygen species in chloroplasts and their functions. Plant Physiol, 141, 391–396. doi:10.1104/pp.106.077693
- Attia H, Karray N, Ellili A, Msilini N, Lachaâl M 2009: Sodium transport in basil. Acta Physiol, 31, 1045–1051. doi:10.1007/s11738-009-0324-1
- Azevedo Neto AD, Tarquinio PJ, Enéas-Filho J, Medeiros JVR, Gomes-Filho E 2005: Hydrogen peroxide pre-treatment induces salt- stress acclimation in maize plants. J. Plant Physiol., 162, 1114–1122. doi:10.1016/j.jplph.2005.01.007
- Baâtour O, Nasri-Ayachi MB, Mahmoudi H et al. 2012: Salt effect on physiological, biochemical and anatomical structures of two Origanum majorana varieties (Tunisian and Canadian). Afr. J. Biotechnol., 11, 7109–7118.
- Bhattacharjee S 2005: Reactive oxygen species and oxidative burst: roles in stress, senescence and signal transduction in plants. Curr. Sci., 89, 1113–1121.
- Bradford MM 1976: A rapid and sensitive method for the quantitation of microgram quantities of protein utilizing the principle of protein–dye binding. Ann. Biochem., 72, 248–254. doi:10.1016/0003-2697(76)90527-3
- Cordovilla MP, Bueno M, Aparicio C, Urrestarazu M 2016: Effects of salinity and the interaction between Thymus vulgaris and Lavandula angustifolia on growth, ethylene production and essential oil contents. J. Plant Nutr., 37, 875–888. doi:10.1080/01904167.2013.873462
- Dumbravă DG, Moldovan C, Raba DN, Popa MV 2012: Vitamin C, chlorophylls, carotenoids and xanthophylls content in some basil (Ocimum basilicum L.) and rosemary (Rosmarinus officinalis L.) leaves extracts. J. Agroalimentary Proc. Technol., 18, 253–258.
- Ferguson IB, Watkins CB, Harman JE 1983: Inhibition by calcium of senescence of detached cucumber cotyledons: effect on ethylene and hydroperoxide production. Plant Physiol, 71, 182–186. doi:10.1104/pp.71.1.182
- Foyer CH, Noctor G 2000: Tansley Review No. 112 Oxygen processing in photosynthesis: regulation and signalling. New Phytol, 146, 359–388. doi:10.1046/j.1469-8137.2000.00667.x
- Ghanem ME, Albacete A, Martines-Andujar C, Acosta M, Romero-Aranda R, Dodd IC, Lutts S, Perez-Alfocea F 2008: Hormonal changes during salinity-induced leaf senescence in tomato (Solanum lycopersicum L.). J. Exp. Bot., 59, 3039–3050. doi:10.1093/jxb/ern153
- Gill SS, Tuteja N 2010: Reactive oxygen species and antioxidant machinery in abiotic stress tolerance in crop plants. Plant Physiol. Biochem., 48, 909–930. doi:10.1016/j.plaphy.2010.08.016
- Gomes MAC, Suzuki MS, Cunha M, Tullii CF 2011: Effect of salt stress on nutrient concentration, photosynthetic pigments, proline and foliar morphology of. Salvinia Auriculata. Acta Limnol., 23, 164–176. doi:10.1590/S2179-975X2011000200007
- Hasanuzzaman M, Nahar K, Alam MM, Bhowmik PC, Hossain MA, Rahman MM, Prasad MNV, Ozturk M, Fujita M 2014: Potential use of halophytes to remediate saline soils. BioMed Res. Int, 2014, 12. doi:10.1155/2014/589341
- Hernández JA, Almansa MS 2002: Short-term effects of salt stress on antioxidant systems and leaf water relations of pea leaves. Physiol. Plant., 115, 251–257. doi:10.1034/j.1399-3054.2002.1150211.x
- Kaiser WM 1979: Reversible inhibition of the Calvin cycle and activation of oxidative pentose phosphate cycle in isolated intact chloroplasts by hydrogen peroxide. Planta, 145, 377–382. doi:10.1007/BF00388364
- Maathuis FJM, Amtmann A 1999: K+ Nutrition and Na+ toxicity: the basis of cellular K+/Na+ ratios. Ann. Bot., 84, 123–133.
- Mouna BT, Kamel M, Karim H, Mohamed H, Mohamed EK, Brahim M 2009: Plant growth, essential oil yield and composition of sage (Salvia officinalis L.) fruits cultivated under salt stress conditions. Industrial Crops and Products, 30, 333–337. doi:10.1016/j.indcrop.2009.06.001
- Parida AK, Das AB 2005: Salt tolerance and salinity effects on plants: a review. Ecotoxicol. Environ. Safety, 60, 324–349. doi:10.1016/j.ecoenv.2004.06.010
- Pathak V, Prasad A, Pospíšil P 2017: Formation of singlet oxygen by decomposition of protein hydroperoxide in photosystem II. PLoS ONE, 12, e0181732. doi:10.1371/journal.pone.0181732
- Raja RR 2012: Medicinally potential plants of Labiatae (Lamiaceae) family: an overview. Res. J. Med. Plant, 6, 203–213. doi:10.3923/rjmp.2012.203.213
- Ramel F, Bitrtic S, Cuiné S, Triantaphylidés C, Ravanat JL, Havaux M 2012: Chemical quenching of singlet oxygen by carotenoids in plants. Plant Physiol, 158, 1267–1278. doi:10.1104/pp.111.189621
- Roby MHH, Sarhan MA, Selim KA, Khalel KI 2012: Evaluation of antioxidant activity, total phenols and phenolic compounds in thyme (Thymus vulgaris L.), sage (Salvia officinalis L.), and marjoram (Origanum majorana L.) extracts. Ind. Crops Products, 43, 827–831. doi:10.1016/j.indcrop.2012.08.029
- Sahay R, Patra DD 2014: Identification and performance of sodicity tolerant phosphate solubilizing bacterial isolates on Ocimum basilicum in sodic soil. Ecol. Eng., 71, 639–643. doi:10.1016/j.ecoleng.2014.08.007
- Said-Al Ahl HAH, Hussein MS 2010: Effect of water stress and potassium humate on the productivity of oregano plant using saline and fresh water irrigation. Ozean J. Appl. Sci., 3, 125–141.
- Said-Al Ahl HAH, Omer EA 2011: Medicinal and aromatic plants production under salt stress: a review. Herba Polonica, 57, 72–86.
- Shabala S, Cuin TA 2007: Potassium transport and plant salt tolerance. Physiol. Plant., 133, 651–669. doi:10.1111/j.1399-3054.2007.01008.x
- Siyal AA, Siyal AG, Abro ZA 2002: Salt affected soils their identification and reclamation. Pak. J. App. Sci., 2, 537–540. doi:10.3923/jas.2002.537.540
- Taarit MB, Msaada K, Hosni K, Marzouk B 2009: Changes in fatty acid and essential oil composition of sage (Salvia officinalis L.) leaves under NaCl stress. Food Chem, 119, 951–956. doi:10.1016/j.foodchem.2009.07.055
- Tanaka K, Otubo T, Kondo N 1982: Participation of hydrogen peroxide in the inactivation Calvin-cycle SH enzymes in SO2-fumigated spinach leaves. Plant Cell Physiol, 36, 1089–1095.
- Tanaka K, Sugahara K 1980: Role of superoxide dismutase in defense against SO2 toxicity and an increase in superoxide dismutase activity with SO2 fumigation. Plant Cell Physiol, 21, 601–611. doi:10.1093/oxfordjournals.pcp.a076035
- Tounekti T, Vadel AM, Oñate M, Khemira H, Munne-Bosch S 2011: Salt-induced oxidative stress in rosemary plants: damage or protection? Environ. Exp. Bot., 71, 298–305. doi:10.1016/j.envexpbot.2010.12.016
- Wintermans J, De Mots A 1965: Spectrophotometric characteristics of Chlorophyll a and b their pheophytins in ethanol. Biochim. Biophys. Acta, 109, 448–453. doi:10.1016/0926-6585(65)90170-6
- Wu S, Sun Y, Niu G, Grimaldo-Pantoja GL, Castro-Rocha A 2016: Responses of six Lamiaceae landscape species to saline water irrigation. J. Environ. Hort., 34, 30–35.