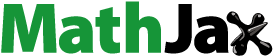
ABSTRACT
Exponential fertilization (EF) can cause seedlings to load more nutrients than they need to grow to establish reserves. Lighting spectrum adjustment may strengthen the growth and nutrient utilization of seedlings, which may modify seedling response to EF. In this study, containerized Prince Rupprecht’s larch (Larix principis-rupprechtii Mayr.) seedlings were cultured in commercial substrates (275.59 mg nitrogen [N] and 60.05 mg phosphorus [P] per plant) and received EF at 0 (control), low (88 mg N and 36 mg P per plant), and high (130 mg N and 54 mg P per plant) doses under continuous lighting for 18 h daily. Two light-emitting diode (LED) spectra with different red (R), green (G), and blue (B) ratios were used as R-tinted (R7BG1) and G + B-tinted (R3BG10) colours. Under the R7BG1 spectrum, seedlings receiving low-dose EF had the best growth in height of 29 cm (P = 0.0100) and root-collar diameter (RCD) of 4.7 mm (P < 0.0001) and the highest N (170 mg plant−1; P < 0.0001) and P contents (154 mg plant−1; P < 0.0001). These seedlings also had the greatest biomass of leaves (P = 0.0005), stems (P = 0.0062), and roots (P = 0.0016) in the high-dose EF treatment. This combined effect resulted in the highest N uptake efficiency of nearly 40%. High-dose EF increased the chlorophyll-a and -b contents, while light spectra modified leaf protein content. Therefore, the LED spectra had an interactive effect with the EF dose on nutrient uptake and utilization in Prince Rupprecht’s larch seedlings with a recommended regime of EF at 88 mg N and 36 mg P per plant under the R7BG1 LED spectrum.
1. Introduction
In the 1980s, the concept of ‘steady-state nutrition’ aroused attention on the importance of internal nutrient cycling in a target plant to overcome exogenous stress (Ingestad and Lund Citation1986). Based on this concept, constant-dose nutrient loading was invented and popularized for tree seedling culture because this fertilizer regime can load more nutrients to seedlings than traditional fertilization. Thereafter, it was found that nutrient loading should be delivered to seedlings at an exponentially increasing rate to induce the steady-state nutrient reserve because the seedling demand for nutrients increased with the exponential growth rate (Timmer Citation1997). Exponential fertilization (EF) has been intensively studied and identified to enhance nursery-stage internal nutrient reserves (Chen et al. Citation2012, Citation2018; Duan et al. Citation2013; Li et al. Citation2017) and promote transplant nutrient retranslocation (Pokharel and Chang Citation2016; Pokharel et al. Citation2017). According to current studies, the optimum amounts for high nutrient uptake and utilization through EF manipulation of seedlings ranged from 200 to 600 mg N seedling−1 for a wide range of tree species (Chen et al. Citation2012, Citation2018; Li et al. Citation2017; Pokharel and Chang Citation2016; Pokharel et al. Citation2017). However, for deciduous conifer species, the optimum dose of EF treatment was usually less than 200 mg N seedling−1 (Duan et al. Citation2013; Park et al. Citation2012), suggesting the potential to load seedlings of this species with more nutrients during seedling nursery culture.
Given that whole-seedling biomass determines the demand for the needed nutrient amount (Ingestad and Lund Citation1986), the effect of EF on the establishment of inherent nutrient reserves can be modified by controlling the biomass accumulation rate through regulating the growth rhythm. For example, Schott, Pinno, and Landhäusser (Citation2013) found that EF was not as efficient for loading nutrients of trembling aspen (Populus tremuloides Michx.) seedlings as for evergreen coniferous seedlings because of the fast growth rate. However, when trembling aspen seedlings were fed with a shoot growth inhibitor in addition to the EF treatment, a significant nutrient reserve was induced in their annual organs. This was because the premature shoot-growth treatment increased nutrient concentrations by retarding biomass accumulation with continuous nutrient uptake and accumulation. Knowledge about the combined effect of EF and other manipulations on seedling growth still needs to be expanded to more species with various growing conditions.
Light is one of the necessary resources for plant growth. The lighting condition determines plant growth and biomass accumulation by impacting photosynthesis. The photoperiod can significantly impact the growth and phenology of trees (Vitasse and Basler Citation2013). A short-day photoperiod induced the formation of apical buds and terminated growth in evergreen seedlings (Wallin et al. Citation2017), but a prolonged photoperiod promoted the growth rate in slowly growing seedlings (Wei et al. Citation2017; Zhu et al. Citation2016). In transplanted seedlings, extending the photoperiod during the cultural period can continuously depress shoot growth (Wang, Zhao, and Wei Citation2017; Zhao et al. Citation2017) and enhance root foraging precision (Wei et al. Citation2017). These can all be taken as the result of the increased demand for N and P in tree seedlings through promoted growth and biomass accumulation by continuous lighting. It was suggested that the EF regime should be modified to meet the seedling demand if the growth and subsequent nutrient demand were changed from the ordinary conditions. For example, it was suggested that the nutrient supply dose through EF be modified if the growth rate was promoted by a prolonged photoperiod (Li et al. Citation2017; Wei, Ren, and Zhou Citation2013a). In another study on oak seedlings, to meet the episodic growth pattern, it was suggested that the classical EF model with more initial nutrient input at the early growing stage should be modified to facilitate nutrient uptake by small root systems and to reduce the possible risk of overdose fertilization at the end of growing season (Birge, Salifu, and Jacobs Citation2006). In our study, larch seedlings were cultured under continuous lighting under LED panels; hence, their demand for N and P tended to be increased compared to those under ordinary conditions. Our seedlings should receive more nutrients than under ordinary conditions, but increasing the nutrient supply dose would also involve the risk of excessive toxicity through over-supplied nutrients and rhizosphere salt (Salifu and Timmer Citation2003). Therefore, we tested the EF at two rates in the acceptable range of the supply and replaced the substrate of poor-nutrient peat with fertile commercial peat. According to the results, this modification was successful because both seedling growth and nutrient efficiency were promoted by the interaction between the LED spectra and the EF regime.
Light spectrum is an important factor for morphological characteristics and transplant performance in tree seedlings (Montagnoli et al. Citation2018). Regarding the development of the light emitting diode (LED) technique, adjusting the lighting spectra can be achieved by changing the component ratio of red (R) (600–700 nm), green (G) (500–600 nm), and blue (B) (400–500 nm) lights in the visible light spectrum. The spectrum from high-pressure sodium (HPS) lamps is widely used for culturing both horticultural and forestry crops (An et al. Citation2018; Wei et al. Citation2013a; Zhu et al. Citation2016). With commercial plants under HPS lighting as the reference, it was found that using some specific light wavelengths from the LED spectra can effectively promote seedling growth and biomass accumulation in oak (Quercus ithaburensis var. macrolepis) (Smirnakou, Ouzounis, and Radoglou Citation2017), subalpine fir (Abies lasiocarpa [Hooker] Nuttall) (Chiang et al. Citation2018), Norway spruce (Picea abies [L.] Karst.), Scots pine (Pinus sylvestris L.) (Riikonen Citation2016; Riikonen et al. Citation2016), and fragrant rosewood (Dalbergia odorifera) seedlings (Li et al. Citation2018). According to the results of these studies, however, it is unlikely that the question of whether the empirically optimum dose of EF is sufficient for seedlings with an enlarged morphology in a changed lighting spectrum can be answered. Little is known about the combined effect of LED spectra and EF on tree seedlings.
Larch species (Larix spp.) have significant value in both ecology and commerce for restoration, afforestation, and landscaping (Wei and Guo Citation2016). Larch species are also typical conifers indigenous to the cool temperate regions in the northern hemisphere (Gower and Richards Citation1990). Increasing anthropogenic-driven N deposition has been imposed on the natural development of the larch population, but the response of larch populations varied among studies depending on different soil types and larch genotypes (Fujita et al. Citation2018; Watanabe et al. Citation2012). Simulated experiments revealed that mimicking a N-depositing load adversely impacts growth and photosynthetic rates in cut seedlings of hybrid F1 larch (Larix gmelinii var. japonica × L. kaempferi) in serpentine soils (Watanabe et al. Citation2012). In contrast, another group of seedlings of this larch cultivar showed increased aboveground growth with N loading regardless of whether P was added to mixed Akadama and Kanuma pumice soils (Fujita et al. Citation2018). The effect of simulated N deposition on ectomycorrhizal structure in F1 plants was intermediate between effects on that in Dahurian larch (L. gmelinii var. japonica) and Japanese larch (L. kaempferi) in volcanic ash soils. At the early stage after transplant, larch seedlings may suffer a short-term shock from nutrient depletion (Wei et al. Citation2013b). Therefore, EF was recommended for the culture of larch seedlings to elevate nutrient uptake and utilization through controlled leaching loss (Park et al. Citation2012) and enhanced nutrient storage (Duan et al. Citation2013; Wei et al. Citation2013b). However, the effect of lighting spectra or its combination with EF has rarely been studied on larch seedlings.
Prince Rupprecht’s larch (Larix principis-rupprechtii Mayr.) is one of the dominant tree species of temperate coniferous forests in North China. The natural Prince Rupprecht’s larch forests are mainly distributed around an elevation range between 1400 m and 2800 m in mountainous areas with a total area of approximately 140,000 ha. Natural soils for Prince Rupprecht’s larch trees are usually alkaline and of low available N in northwest China, which limited the N uptake and utilization (Meng et al. Citation2016). The natural self-thinning of Prince Rupprecht’s larch forests was aggravated on steep slopes (Xue et al. Citation1999). The over-exploitation of timber from Prince Rupprecht’s larch forests has resulted in the decline of the natural distribution of the species and degradation of site quality. According to the national standard for seedling quality, the culture of Prince Rupprecht’s larch seedlings takes up to two years to meet the basic standard of 20 cm in height and 0.4 cm in root-collar diameter (RCD) (National Forestry Administration of China Citation1999). Practical culture aimed at passing this quality standard usually requires up to at least two years of propagation with bare-root stocks. Based on the current understanding of LED lighting effects on tree seedling growth (Li et al. Citation2017, Citation2018; Zhu et al. Citation2016), a proper spectrum may exist to promote the growth of Prince Rupprecht’s larch seedlings to pass the national standard of seedling quality in a shorter time, e.g., one growing season, than the conventional regime. In addition, EF needs to be combined with spectral treatment to further promote growth with a sufficient nutrient supply and to eliminate the risk of nutrient reserve decline.
Shanxi Province is well known as ‘the Hometown of the Prince Rupprecht’s Larch’ in China (Di et al. Citation2014). In the present study, Prince Rupprecht’s larch seeds were collected from Shanxi, and seedlings were cultured with a combination of two LED spectra and three EF regimes. We hypothesized that the interactive effects of the LED spectra and EF promotes the growth of Prince Rupprecht’s larch seedlings to pass the quality standard in a single growing season, thereby enhancing nutrient uptake and nutrient utilization.
2. Materials and methods
2.1. Seedling material
Prince Rupprecht’s larch seeds were collected from mature trees distributed in Guanqinshan (38°48ʹ N, 112°05ʹ E), Shanxi, China. Seeds were sterilized by potassium permanganate at a concentration of 0.5% (v/v) for two hours on 2 March 2018. Seeds were cleaned by distilled water and sown in sand with a moisture content of 60% at a constant temperature of 20°C in the Laboratory of Combined Manipulation of Illumination and Fertility on Plant Growth (Zhilunpudao® Agric. For. Sci. Ltd., Changchun, China). Sunlight was avoided thoroughly by blackout curtains. Hence, artificial lighting was the only illumination source under this condition. No interloper was allowed during the experiment to keep temperature and relative humidity (RH) stable and enable controlled-condition plant propagation.
2.2. Seedling culture
One week after seeding, seeds were moved to plastic seedling trays. The commercial substrate of mixed peat, spent-mushroom-residue, and perlite (55:20:25, v/v/v) (Mashiro-DustTM, Zhiluntuowei® Agric. For. Sci. Ltd., Changchun, Jilin, China) was used to fill the planting holes (7 cm × 13 cm, top-diameter × height) in the tray as the cultural substrate. The initial substrates were determined to have an ammonium N content of 79.99 ± 8.23 mg kg−1 (three replicated observations; the same below), nitrate N content of 1.67 ± 0.78 g kg−1, available P content of 0.36 ± 0.05 g kg−1, organic matter content of 12.9 ± 0.15%, pH of 4.82 ± 0.02, and electrical conductivity (EC) of 1.62 ± 0.15 mS cm−1. There were 32 holes (4 × 8) in one tray, but only half of them (every two holes) were used in this study to eliminate the effect of high density on stem growth. In the centre of the hole, a stick (0.5 cm in diameter) was inserted to the depth of 3 cm in fully watered substrate. Six seeds were transplanted to one planting hole in the tray filled with the commercial substrate. In total, eighteen trays were employed for seeding. On 2 April 2018, germinated seedlings were thinned to leave one individual in one planting plug, resulting in 288 uniform-sized seedlings used in this study.
2.3. Illumination treatments
In this study, trays were placed on the floors of metallic frames in the laboratory and isolated from sunlight to ensure that the seedlings received solely artificial LED lighting as the growth illumination. Six metallic frames (height × width × length, 2 m × 0.5 m × 1.5 m) were employed with three floors of cultural cells (height × width × length, 0.5 m × 0.5 m × 1.5 m) within each frame. An LED panel (height × width × length, 0.10 m × 0.4 m × 1.2 m) was fixed to the ceiling of each frame cell. Therefore, the initial distance between the LED panel and the seedlings was 37 cm with regard to the frame height of 50 cm and tray-height of 13 cm. The final distance was 8 ~ 22 cm depending on the height growth the of seedlings receiving different treatments. One hundred lighting diodes were placed on the downward surface of the LED panel at a spacing of 2 cm × 2 cm. Every diode was covered by a filter to control the light-power loss by lateral scattering. Every three adjacent diodes were designed to emit R, G, and B light, whereas the electric flow of R light was controlled by a 200-W electrical transformer and the electric flows of G and B lights were together controlled by a 135-W electrical transformer. All electric flows for R and G + B lights were regulated by far infrared remote. Hence, the light spectrum could be modified by regulating the ratio of R and G + B light intensities by controlling the electric flows.
Two light spectra were employed in this study. One spectrum was set to emit R-tinted light, while the other spectrum was set to emit G + B-tinted light. The R-tinted light was generated by controlling the R light electrical flow at 70% and the G + B light electrical flow at 10% (R7BG1). G + B light was generated by controlling the R light electrical flow at 30% and the G + B- light flow at 100% (R3BG10). The spectra for the two types of lighting are shown in . At a height of 25 cm above the floor of each cell room, the photosynthetic photon flux rate (PPFD) was measured to be 200 μmol m−2 s−1. Three metallic frames were arranged to raise seedlings with R-tinted light, while the other three frames were arranged with G + B-tinted light. A couple of R light and G + B light frames were placed together as a block; hence, three couples of frames were set as three replicated blocks.
All electrical flows were turned on once the seedlings were thinned on 2 April 2018. According to results from previous studies (Li et al. Citation2017; Wei, Ren, and Zhou Citation2013a, Citation2013b), the daily photoperiod was set to 18 h per day from 6:00 a.m. to 24:00 p.m. This photoperiod was used for the culture of coniferous seedlings from different latitudinal populations (Apostol et al. Citation2015). Temperature and RH on the floor of each room were monitored throughout the entire experiment ().
2.4. Fertilizer treatments
Three seedling trays were randomly placed on one of the three floors in one frame. Every tray of seedlings received one of the fertilizer treatments. Fertilizer treatment also started the day the seedlings were thinned on 2 April 2018. Seedlings received EF at low and high rates of N and P with an unfertilized control. Fertilizers were delivered through 28 applications, while control seedlings only received water irrigation at each fertilizer application. In the low rate fertilizer treatment, nutrients were delivered at the dose of 88 mg N seedling−1 and 36 mg P seedling−1, while in the high rate fertilizer treatment, N and P were supplied at 130 mg seedling−1 and 54 mg seedling−1, respectively. Rates of N application under the EF regime were employed according to the classical model frequently used in relevant studies (Duan et al. Citation2013; Wei, Ren, and Zhou Citation2013a):
where r is the relative addition rate required to increase NS to a final N content (NT + NS) over the number of fertilizer applications (t = 28). NS and NT are the initial N content in seedlings and the desired amount to be added, respectively. Nutrients were added to seedlings twice a week, and fertilization lasted for four months until early August 2018.
2.5. Experimental design
The experiment was designed as a split-block arrangement. The main blocks were split by the two spectra treatments, and the sub-plot included the three fertilizer regimes. Every combined treatment was replicated for three blocks. Sixteen seedlings per tray were taken as one observation object, and six trays were taken in one block. Hence, a total of 288 seedlings (two photoperiods × three fertilizer regimes × three replicated blocks × sixteen seedlings per block) were used in all.
2.6. Seedling sampling, measurement, and chemical analysis
Height and root-collar diameter (RCD) were measured for four randomly sampled seedlings on 5 August 2018. Thereafter, four seedlings were randomly sampled for chlorophyll and protein analyses (Nosheen et al. Citation2018). Because the place to culture seedlings was quite close to the laboratory, seedlings were sampled and transported to the laboratory in an ice chamber (0 ~ 2°C). As soon as sampled seedlings arrived at the laboratory, they were determined for the first time. Briefly, chlorophyll was determined using a leaf sample of 0.05 g which was previously soaked in 2.5 mL of dimethyl suofoxidein the test tubes, heated at 65°C for 1 h in a water bath, and held for 24 h. The extraction was measured by a spectrophotometer at 663 nm and 645 nm for chlorophyll a and b contents, respectively. The carotenoid amount was then estimated by the equation using a spectrophotometer at 470 nm. Protein content was determined using fresh leaf sample (0.1 g) which was ground in 1 mL of phosphate buffer of pH 7.5, centrifuged at 3000 rpm for 10 min, treated by 0.1 mL of Folin’s reagent and determined at 650 nm. Another four sampled seedlings were divided into leaves, stems, and roots. In this study, leaves included acicular needles at the tip of each branch and young twigs with green colour and a stem contained all woody aerial organs of the main stem and brown branches. Seedling parts were oven-dried at 70°C for 3 d, ground to powder, and used for digestion. Briefly, a sample of 0.2 g dried power was digested in 5 ml of H2SO4-H2O2 (7/3, v/v). Fully digested samples were collected in plastic bottles in a volume of 50 ml and used for chemical analysis in two days. N and P were analysed by the same method of Wei et al. (Citation2017). The N concentration was determined using the Kjeldahl method (UDK 152 automatic N analyser, VELP® Co., Usmate, MB, Italy), and the P concentration was determined by ICP-OES (Vista-MPX, Varian®, USA).
2.7. Data calculation
Nutrient uptake efficiency (UE) was calculated by the model:
where NFertilized is the nutrient content in fertilized seedlings, NControl is the nutrient content in the control, and Ninput is the total amount of nutrients absorbed by seedlings, including nutrients from both fertilizers and container substrate. Aside from fertilizer addition, the initial substrate supplied each seedling with 275.59 mg N and 60.05 mg P by 1.647 mg g−1 of hydrolysed N and 0.359 mg g−1 of available P in the volume of a 480 cm3 container filled with substrate at the bulk density of 0.349 g cm−3. Thus, UE can be calculated for either N (NUE) or P (PUE). The nutrient utilization index (UI) was calculated according to the model by Hawkins (Citation2007):
where BWP is the total biomass accumulation in the whole plant (g), and %NFoliar is the foliar nutrient concentration (%). Again, UI can be calculated for either N (NUI) or P (PUI).
2.8. Statistical analysis
Data were analysed using SAS (SAS Institute Inc., NC, USA). A normality test was conducted for data for every parameter, and no transformation was necessary. Data were first analysed by ANOVA to detect the combined effects of spectra and fertilizer regime on indices according to the general linear model. When a significant effect was indicated, the means were ranked according to Tukey’s studentized range test at the α = 0.05 level.
3. Results
3.1. Seedling growth and biomass accumulation
Treatments of lighting spectra and fertilizer regime had combined effects on all parameters of seedling growth and biomass accumulation except for the root to shoot biomass ratio (R/S) (). Seedling height was higher in the low- and high-dose fertilizer treatment under the R7BG1 spectrum than in the other treatments. The maximum height tended to be higher than 24 cm. RCD was highest in the low-dose fertilizer treatment under the R7BG1 spectrum, which was higher than 0.4 cm. RCD failed to reach 0.4 cm in all other treatments. Biomass accumulation was highest in leaves, stems, roots, and whole plants in the high-dose fertilizer treatment under the R7BG1 spectrum. R/S was higher under the R3BG10 spectrum (0.60 ± 0.12, mean±SE, the same below) than under the R7BG1 spectrum (0.39 ± 0.08).
Table 1. Growth and biomass accumulation in Prince Rupprecht’s larch (Larix principis-rupprechtii Mayr.) seedlings exposed to the control, low, and high dose of fertilizer treatments (F) under two lighting spectra treatments (L) of R3BG10 and R7BG1
3.2. N and P concentrations
Treatments of lighting spectra and fertilizer regime had an interactive effect on N concentration in leaves and roots (). Seedlings in the control under the R3BG10 spectrum had the lowest N concentration in both leaves and roots, while the high-dose fertilizer treatment under the R7BG1 resulted in the highest root N concentration ()).
Table 2. P values from ANOVA on effects of lighting spectra (L), fertilizer (F), and the interaction (L × F) on N and P concentrations and contents in Prince Rupprecht’s larch (Larix principis-rupprechtii Mayr.) seedlings
Figure 3. Nitrogen (N) (a) and phosphorus (P) concentrations (b) in organs of leaves, stem, and root in Prince Rupprecht’s larch (Larix principis-rupprechtii Mayr.) seedlings cultured with exponential fertilization at doses of 0 (control), low, and high under two lighting spectra of R3BG10 and R7BG1 treatments. Bars are the means of three replicated blocks (n = 3). Different letters indicate significant difference among combined treatments. Lower case letters of a and b label the difference for leaves, and letters of x, y, and z label the difference for root
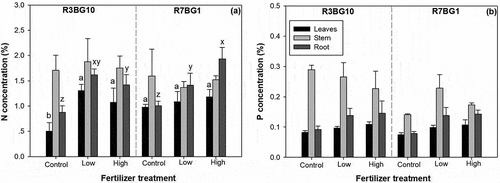
Although no interactive effect was found on P concentration in any seedling organs, the EF treatment had a significant effect on P concentration in leaves and roots, while the spectral treatment had a significant effect on P concentration in stems (). EF increased the P concentration in both leaves and roots under both conditions, and the difference between the two doses was not statistically significant. In leaves, the P concentration in the EF treatment ranged between 0.98 ± 0.06 mg g−1 at the low dose and 1.08 ± 0.12 mg g−1 at the high dose, which was higher than that in the control (0.78 ± 0.07 mg g−1) by 38% and 25%, respectively ()). In the roots, the P concentration was 1.38 ± 0.22 mg g−1 and 1.44 ± 0.26 mg g−1 in the low- and high-dose EF treatments, which were higher than that in the control (0.85 ± 0.10 mg g−1) by 62% and 69%, respectively ()). The P concentration in stems was higher under the R3BG10 spectrum (1.31 ± 0.22 mg g−1) than under the R7BG1 spectrum (0.91 ± 0.20 mg g−1).
3.3. N and P contents
Treatments of lighting spectra and fertilizer regime had an interactive effect on N and P contents in all seedling organs (). Generally, the high-dose fertilizer treatment under R7BG1 resulted in the highest N and P contents in all seedling organs (). Control seedlings tended to have the lowest N and P contents regardless of the lighting spectrum.
Figure 4. Nitrogen (N) (a) and phosphorus (P) contents (b) in organs of leaves, stem, and root in Prince Rupprecht’s larch (Larix principis-rupprechtii Mayr.) seedlings cultured with exponential fertilization at doses of 0 (control), low, and high under two lighting spectra of R3BG10 and R7BG1 treatments. Bars are the means of three replicated blocks (n = 3). Different letters indicate significant difference among combined treatments. Lower case letters of a, b, c, and d label the difference for leaves; letters of α, β, and χ label the difference for stem; letters of x, y, and z label the difference for root
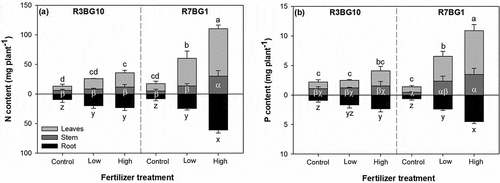
3.4. Chlorophyll and protein contents
Lighting spectra had no effect on chlorophyll content, but the fertilizer regime did affect chlorophyll content (). The chlorophyll-a content was increased by the EF, but the difference between the two fertilizer regimes was not significant (). The low-dose fertilizer treatment resulted in the highest chlorophyll-b content. In contrast, the spectrum treatment had a significant effect on leaf protein content. Seedlings under the R3BG10 spectrum had higher leaf protein content than those under the R7BG1 spectrum.
Table 3. Chlorophyll and protein contents in needles of Prince Rupprecht’s larch (Larix principis-rupprechtii Mayr.) seedlings exposed to the control, low, and high dose of fertilizer treatments (F) under two lighting spectra treatments (L) of R3BG10 and R7BG1
3.5. Nutrient uptake and utilization efficiencies
The spectra and fertilizer regime treatments had an interactive effect on NUE (F = 26.96; P = 0.0008). NUE was highest in the high-dose EF treatment under the R7BG1 spectrum and lower in the low-dose EF treatment under the R3BG10 treatment than under the R7BG1 spectrum ()). The two types of treatments had no interactive effect on PUE (F = 2.86; P = 0.1292). However, either lighting spectra treatments or EF had a significant main effect on PUE (spectra, F = 86.48, P < 0.0001; EF, F = 15.35, P = 0.0044). Compared to PUE under the RGBG10 spectrum (2.01 ± 1.04%), that under the R7BG1 spectrum (9.49 ± 2.97%) was higher by 370% ()). Compared to PUE in the low-dose EF treatment (4.17 ± 3.18%), that in the high-dose EF treatment (7.31 ± 4.62%) was higher by 75% ()).
Figure 5. Nutrient uptake (top) and utilization efficiencies (bottom) in Prince Rupprecht’s larch (Larix principis-rupprechtii Mayr.) seedlings cultured with exponential fertilization at low and high doses under two lighting spectra of R3BG10 and R7BG1 treatments. Bars are the means of three replicated blocks (n = 3). Different letters indicate significant difference among combined treatments. NUE, nitrogen (N) uptake efficiency; PUE, phosphorus (P) uptake efficiency; NUI, N utilization efficiency; PUI, P utilization efficiency
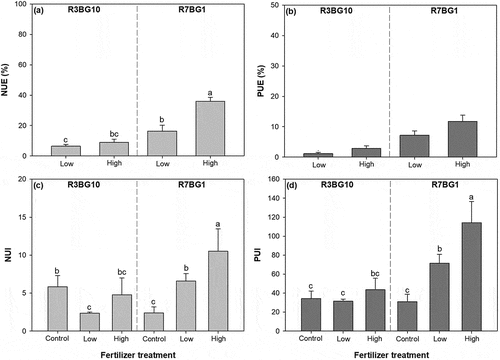
The two types of treatments also had an interactive effect on both NUI (F = 11.40; P = 0.0017) and PUI (F = 12.78; P = 0.0011). The high-dose fertilizer treatment resulted in the highest NUI and PUI under the R7BG1 spectrum, while both NUI and PUI were not significantly different between the low- and high-dose EF treatments under the R3BG10 spectrum ().
4. Discussion
Biomass allocation is one of the important mechanisms that regulates a plant’s response to changes in substrate fertility. Previous studies on tree seedlings found that the R/S ratio can be promoted by a lower dose EF treatment (Chen et al. Citation2012; Uscola et al. Citation2015). A study on bare-root Changbai larch (L. olgensis) seedlings revealed that EF at the rate of 135 mg plant−1 induced a higher root biomass ratio than that at the rate of 500 mg plant−1 (Duan et al. Citation2013). However, in our study, it was surprising that the fertilizer regime had no effect on the R/S ratio, suggesting that EF had no effect on biomass allocation regardless of the dose. This was caused by the usage of substrate in our study. The initial substrate had already provided Prince Rupprecht’s larch seedlings with 275.59 mg N and 60.05 mg P per plant before the supply of treatment fertilizers. Thus, seedlings in the control received these nutrients and did not like have a ‘nutrient deficient status’ (Salifu and Timmer Citation2003) because control seedlings already had heights of approximately 15 cm and RCD of approximately 2.6 mm. In addition, the low-dose EF treatment supplied 88 mg N and 36 mg P per plant, generating a total amount of 363.59 mg N and 96.0 mg P per plant; the high-dose EF treatment supplied 130 mg N and 54 mg P per plant, generating a total amount of 405.59 mg N and 114.05 mg P per plant. Therefore, the unchanged R/S among EF treatments probably occurred because all seedlings in our study fell into the nutritional status of ‘luxury consumption’ where biomass allocation was unchanged by additional nutrient supply (Salifu and Timmer Citation2003). This was reasonable because the proper nutrient supplies in most studies of EF fell in the range of 100 to 150 mg N per plant (including both nutrients from the substrate and fertilizer) under natural lighting conditions (Chen et al. Citation2012; Duan et al. Citation2013; Uscola et al. Citation2015), and our nutrient supply was much higher than those of previous studies.
In our study, 4-month culture under a controlled environment can achieve the goal of shaping the target shoot morphologies. This achievement occurred in seedlings receiving low-dose fertilizers under the R7BG1 spectrum. The total inputs of N and P in the low-dose EF treatment were 360 mg and 96 mg per seedling, respectively, derived from both fertilizer and the substrate. This dose was higher than all those of the current studies but did not cause toxicity. This can be attributed to the effect of continuous LED lighting, which increased the nutrient demand. Nevertheless, seedlings under the R3BG10 spectrum all failed to pass the morphological standard in both height and RCD regardless of the dose of fertilizers delivered to them. Actually, under this lighting spectrum, seedlings had very rare responses of growth and biomass accumulation to the EF treatment. This was mostly because the R3BG10 spectrum was relatively high in the R and B ratio and low in the R light ratio, which limited photosynthetic production and morphological growth even under different fertile conditions. It was summarized that the G light can be a signal to slow down or stop plant growth (Folta and Maruhnich Citation2007). Another study on lettuce cultivars demonstrated that increasing the B-to-R ratio negatively impacts growth and biomass (Son and Oh Citation2013). Under the R7GB1 spectrum, although seedlings with high-dose fertilization (130 mg N and 54 mg P per seedling) also grew to a height greater than 20 cm, their diameter growth failed to reach 0.4 cm. This can be caused by the over-fertilization to 405.6 mg N and 114.05 mg P per seedling with nutrients from both fertilizer and the substrate. Duan et al. (Citation2013) reported that a dose of 500 mg N seedling−1 caused toxic symptoms in bare-root L. olgensis seedlings. Thus, seedlings in this high dose EF treatment accumulated more biomass in leaves and stems than those in the low dose EF treatment, and the biomass did not account for shoot morphological traits, suggesting that seedlings did not need much biomass with a higher dose of fertilizer supply. Therefore, we can accept our first hypothesis.
The use of 18 h of daily photoperiod in this study was longer than that for Dalbergia odorifera seedlings (14 ~ 17 h) (Li et al. Citation2017, Citation2018) but shorter than that for Podocarpus macrophyllus seedlings (Wei et al. Citation2013a). However, the 18-h photoperiod was used for the culture of conifer seedlings from three latitudinal populations for 17 weeks (Apostol et al. Citation2015). Our results regarding seedling growth illustrated that the 18-h photoperiod was effective for Prince Rupprecht’s larch seedlings. Because plants need some dark time to accumulate carbohydrates every 24 hours, a longer time of illumination would occupy this ‘rest time’ and restrict growth (Li et al. Citation2017).
Among the lighting spectrum for plant growth, B (400–500 nm) and R (600–700 nm) lights are two specific lights that attract the most attention as growth simulating LED light sources, mostly because they are considered to cover the range with maximal chlorophyll a and b absorbance at 430–660 nm (Taiz and Zeiger Citation2010; Montagnoli et al. Citation2018). In addition, the G (500–600 nm) light can also lead to adaptive changes for optimal growth in the morphology and physiology of crops (Zhang et al. Citation2016) because G light in strong white light can penetrate into the leaf through the surface chloroplasts and increase leaf photosynthesis compared to R and B lights (Terashima et al. Citation2009). Although these conclusions seemed to be reasonable as the framework to support the hypothesis for photobiology, their objectivity may be not as strong as needed to be verified. In our study, we found that lighting spectra had no effect on chlorophyll-a, chlorophyll-b, and carotenoid contents in Prince Rupprecht’s larch seedlings. In Quercus ithaburensis var. macrolepis seedlings, Smirnakou, Ouzounis, and Radoglou (Citation2017) also found a similar chlorophyll content index among different lighting spectra. Apostol et al. (Citation2015) reported that Douglas-fir (Pseudotsuga menziesii [Mirb.] Franco) and Engelmann spruce (Picea engelmannii Parry ex Engelm.) seedlings from all seed sources did not respond to lighting spectra . Riikonen et al. (Citation2016) found that the spectra effect was significant for Scots pine (Pinus sylvestris) seedlings but not for Norway spruce (Picea abies) seedlings. In our study, the N concentration in the leaves of Prince Rupprecht’s larch seedlings was also irrelevant to the main effect of the spectra. Instead, we found that EF had a significant effect on chlorophyll content, and the low-dose fertilizer treatment tended to result in the highest contents of chlorophyll-a and chlorophyll-b. These results suggested that EF can promote chlorophyll content and lead to an interactive effect with lighting spectra on shoot growth. EF was also found to promote chlorophyll content with an appropriate fertilizer dose in Mytilaria laosensis seedlings (Chen et al. Citation2012). In contrast to the chlorophyll results, foliar soluble protein content was modified by the spectrum treatment, and higher values were found by the lower R:B ratio spectrum treatment of R3BG10. In Chinese cabbage (Brassica campestris L.) seedlings, both Avercheva et al. (Citation2009) and Fan et al. (Citation2013) found a significant response of protein content to LED treatment as well. However, in these two studies, the R/B ratio of LED lighting was set to be higher than 5:1. In lettuce (Lactuca sativa L. var. capitata), Lin et al. (Citation2013) failed to find any significant response of protein content to spectra treatment. These results suggest a gross trend of species-specific responses of protein content to lighting spectra, but the further mechanism for protein synthesis and accumulation needs to be determined in the future.
In studies of EF of tree seedlings, leaves were used for biomass accumulation and nutrient content analysis instead of the whole plant because authors considered that nutritional status in leaves can reflect the nutritional state at the whole-plant scale (Timmer and Stone Citation1978; Close et al. Citation2005; Wang et al. Citation2016; Li et al. Citation2017). In Prince Rupprecht’s larch seedlings, whole-plant biomass did not change in response to different fertilizer regimes under the R3BG10 spectrum. In contrast, whole-plant biomass increased with the dose of nutrient supplies in EF under the R7BG1 spectrum. The response of leaf biomass to interactive effects of spectra and EF treatments was similar to that of whole-plant biomass. Under the R3BG10 spectrum, leaf biomass was unchanged with rising EF doses, but leaf N concentration was increased. Under the R7BG1 spectrum, leaf biomass accumulated to increase with the rising dose of EF treatment, but leaf N concentration was not significantly different among treatments. These results suggested that the leaf biomass response to EF treatment across doses can affect N content in terms of units per dry mass by modifying the demand for N. Wei, Ren, and Zhou (Citation2013a) also found that leaf N concentration in Buddhist pine (Podocarpus macrophyllus) seedlings was unaffected by EF because the extended photoperiod stimulated biomass accumulation and diluted the N content per dry mass.
The P reserves are as important as those of N in seedling post-transplant performance (Uscola et al. Citation2015). Soil P is easily fixed and difficult for roots to absorb. EF was proven to load P as reserves in Mediterranean oak (Quercus ilex) (Uscola et al. Citation2015), Mytilaria laosensis (Chen et al. Citation2012), fragrant rosewood (Li et al. Citation2017) and Betula alnoides (Chen et al. Citation2018) seedlings. We also found that EF can increase the P concentration in both leaves and roots of Prince Rupprecht’s larch seedlings compared to the control. In another larch species of L. leptolepis, Park et al. (Citation2012) reported that no difference in P concentration occurred among the treatments of EF, constant loading, and three-stage fertilization. This contradiction might be generated because Park et al. (Citation2012) supplied much higher P fertilizer through EF (195 mg P plant−1) to seedlings compared to the other studies (approximately 50‒200 mg P plant−1). In addition, because larch is an ectomycorrhizae-dependent species, EF may also promote P concentration by modifying the fungal community (Wang et al. Citation2018). In this study, we did not observe any effect of lighting spectra on P concentration in Prince Rupprecht’s larch seedlings. In line with our results, Li et al. (Citation2018) also did not find any effect of light spectra on P concentration in fragrant rosewood seedlings. Both our study and that by Li et al. (Citation2018) employed continuous lighting for at least 15 h per day for seedling culture. However, Li et al. (Citation2017) found that supplementary lighting for 3‒6 h per day can cause a significant response of P concentration in fragrant rosewood seedlings. Perhaps continuous lighting led to an excessive length of daily photoperiod for P concentration in the leaves and roots of Prince Rupprecht’s larch seedlings. The higher P concentration in Prince Rupprecht’s larch seedlings under the R3BG10 spectrum than under the R7BG1 spectrum occurred because shoot growth and greater biomass accumulation in stems under the R7BG1 spectrum diluted the P concentration therein. Therefore, it is reasonable to accept our second hypothesis.
Most nutrient content results followed the trend of biomass response. Nutrient content tended to increase with the dose of fertilizer supply in EF treatment under the R7GB1 spectrum, while most nutrient content results were not statistically different between the two EF treatments under the R3BG10 spectrum. Accordingly, a higher dose of EF treatment resulted in higher NUE under the R7BG1 spectrum. The results of NUE and PUE both ranged between 15% and 40% in our study; therefore, our PUE results were similar to those of other studies, but our NUE results tended to be lower than those of other studies (Dumroese et al. Citation2005; Chen et al. Citation2012; Park et al. Citation2012; Uscola et al. Citation2015). However, Prince Rupprecht’s larch seedlings generally had higher NUI and comparable PUI compared to other studies (Hawkins Citation2007; Li et al. Citation2017, Citation2018). These results together suggest that the growth of Prince Rupprecht’s larch seedlings did not heavily depend on the nutrient supply due to the high nutrient use efficiency, but their response to EF was sensitive to the lighting environment. Hence, we can accept our third hypothesis.
5. Conclusion
In the control environment, containerized Prince Rupprecht’s larch seedlings reached the target morphology (20 cm in height and 0.4 cm in RCD) in a 4-month time, which usually requires up to at least two years of propagation as bare-root stocks. Our study determined a methodology for elevating Prince Rupprecht’s larch seedling quality by testing the combined effects of lighting spectra and EF treatment. As a result, we found that seedling production under the R7BG1 spectrum with a low-dose EF regime of 88 mg N and 36 mg P per seedling could be sufficient if target morphology was taken as the cultural goal. However, when the aim is to achieve the highest nutrient uptake and utilization, a higher dose of 130 mg N and 54 mg P per seedling is suggested. Our study is more valuable for the methodological layout than for determining the optimum combination of light spectra and fertilization for the culture of Prince Rupprecht’s larch seedlings. Our results can be referred to by relevant works on the culture of other larch seedlings under controlled plant propagation conditions. A much wider range of spectra and more EF doses may generate much better interactive effects on nutrient utilization in seedlings of other species in future work.
Disclosure statement
No potential conflict of interest was reported by the authors.
Additional information
Funding
References
- An, B. Y., H. X. Wei, L. L. Li, and P. Guo. 2018. “Nutrient Uptake and Utilization and Antioxidants of Fruits in Red Raspberry (Rubus Idaeus L.) Cultivar ‘Autumn Bliss’ in Response to Fertilization under Extended Photoperiod.” Notulae Botanicae Horti Agrobotanici Cluj-Napoca 46: 440–448. doi:10.15835/nbha46211065.
- Apostol, K. G., R. K. Dumroese, J. R. Pinto, and A. S. Davis. 2015. “Response of Conifer Species from Three Latitudinal Populations to Light Spectra Generated by Light-Emitting Diodes and High-Pressure Sodium Lamps.” Canadian Journal of Forest Research. Journal Canadien De La Recherche Forestiere 45: 1711–1719. doi:10.1139/cjfr-2015-0106.
- Avercheva, O. V., Y. A. Berkovich, A. N. Erokhin, T. V. Zhigalova, S. I. Pogosyan, and S. O. Smolyanina. 2009. “Growth and Photosynthesis of Chinese Cabbage Plants Grown under Light-Emitting Diode-Based Light Source.” Russian Journal of Plant Physiology: a Comprehensive Russian Journal on Modern Phytophysiology 56: 14–21. doi:10.1134/S1021443709010038.
- Birge, Z. K. D., K. F. Salifu, and D. F. Jacobs. 2006. “Modified Exponential Nitrogen Loading to Promote Morphological Quality and Nutrient Storage of Bareroot-Cultured Quercus Rubra and Quercus Alba Seedlings.” Scandinavian Journal of Forest Research 21: 306–316. doi:10.1080/02827580600761611.
- Chen, L., C. S. Wang, B. Dell, Z. G. Zhao, J. J. Guo, D. P. Xu, and J. Zeng. 2018. “Growth and Nutrient Dynamics of Betula Alnoides Seedlings under Exponential Fertilization.” Journal of Forestry Research 29: 111‒119. doi:10.1007/s11676-017-0427-2.
- Chen, L., J. Zeng, H. Y. Jia, J. Zeng, W. F. Guo, and D. X. Cai. 2012. “Growth and Nutrient Uptake Dynamics of Mytilaria Laosensis Seedlings under Exponential and Conventional Fertilizations.” Soil Science and Plant Nutrition 58: 618‒626. doi:10.1080/00380768.2012.708879.
- Chiang, C., O. T. Aas, M. R. Jetmundsen, Y. K. Lee, S. Torre, I. S. Fløistad, and J. E. Olsen. 2018. “Day Extension with Far-Red Light Enhances Growth of Subalpine Fir (Abies Lasiocarpa [Hooker] Nuttall) Seedlings.” Forests 9: 175. doi:10.3390/f9040175.
- Close, D. C., I. Bail, S. Hunter, and C. L. Beadle. 2005. “Effects of Exponential Nutrient-Loading on Morphological and Nitrogen Characteristics and on After-Planting Performance of Eucalyptus Globulus Seedlings.” Forest Ecology and Management 205: 397–403. doi:10.1016/j.foreco.2004.10.041.
- Di, X. Y., X. N. Lii, Q. X. Wang, and M. B. Wang. 2014. “Genetic Diversity of Natural Populations of Larix Principis-Rupprechtii in Shanxi Province, China.” Biochemical Systematics and Ecology 54: 71‒77. doi:10.1016/j.bse.2013.12.035.
- Duan, J., C. Y. Xu, D. F. Jacobs, L. Y. Ma, H. X. Wei, L. N. Jiang, and J. Ren. 2013. “Exponential Nutrient Loading Shortens the Cultural Period of Larix Olgensis Seedlings.” Scandinavian Journal of Forest Research 28: 409‒418. doi:10.1080/02827581.2013.778328.
- Dumroese, R. K., D. S. Page-Dumroese, K. F. Salifu, and D. F. Jacobs. 2005. “Exponential Fertilization of Pinus Monticola Seedlings: Nutrient Uptake Efficiency, Leaching Fractions, and Early Outplanting Performance.” Canadian Journal of Forest Research. Journal Canadien De La Recherche Forestiere 35: 2961–2967. doi:10.1139/x05-226.
- Fan, X. X., J. Zang, Z. G. Xu, S. R. Guo, X. L. Jiao, X. Y. Liu, and Y. Gao. 2013. “Effects of Different Light Quality on Growth, Chlorophyll Concentration and Chlorophyll Biosynthesis Precursors of Non-Heading Chinese Cabbage (Brassica Campestris L.).” Acta Physiologiae Plantarum / Polish Academy of Sciences, Committee of Plant Physiology Genetics and Breeding 35: 2721–2726. doi:10.1007/s11738-013-1304-z.
- Folta, K. M., and S. A. Maruhnich. 2007. “Green Light: A Signal to Slow down or Stop.” Journal of Experimental Botany 58: 3099–3111. doi:10.1093/jxb/erm130.
- Fujita, S., X. N. Wang, K. Kita, and T. Koike. 2018. “Effects of Nitrogen Loading under Low and High Phosphorus Conditions on Above- and Below-Ground Growth of Hybrid Larch F1 Seedlings.” iFor. Biogeosci. Forestry 11: 32‒40.
- Gower, S. T., and J. H. Richards. 1990. “Larches: Deciduous Conifers in an Evergreen World.” BioScience 40: 818‒826. doi:10.2307/1311484.
- Hawkins, B. J. 2007. “Family Variation in Nutritional and Growth Traits in Douglas-Fir Seedlings.” Tree Physiology 27: 911‒919. doi:10.1093/treephys/27.4.519.
- Ingestad, T., and A. B. Lund. 1986. “Theory and Techniques for Steady-State Mineral Nutrition and Growth of Plants.” Scandinavian Journal of Forest Research 1: 439‒453. doi:10.1080/02827588609382436.
- Li, X. W., Q. X. Chen, H. Q. Lei, J. W. Wang, S. Yang, and H. X. Wei. 2018. “Nutrient Uptake and Utilization by Fragrant Rosewood (Dalbergia Odorifera) Seedlings Cultured with Oligosaccharide Addition under Different Lighting Spectra.” Forests 9: 29. doi:10.3390/f9010029.
- Li, X. W., Y. Gao, H. X. Wei, H. T. Xia, and Q. X. Chen. 2017. “Growth, Biomass Accumulation and Foliar Nutrient Status in Fragrant Rosewood (Dalbergia Odorifera TC Chen) Seedlings Cultured with Conventional and Exponential Fertilizations under Different Photoperiod Regimes.” Soil Science and Plant Nutrition 63: 153‒162. doi:10.1080/00380768.2017.1312518.
- Lin, K. H., M. Y. Huang, W. D. Huang, M. H. Hsu, Z. W. Yang, and C. M. Yang. 2013. “The Effects of Red, Blue, and White Light-Emitting Diodes on the Growth, Development, and Edible Quality of Hydroponically Grown Lettuce (Lactuca Sativa L. Var. Capitata).” Scientia Horticulturae 150: 86–91. doi:10.1016/j.scienta.2012.10.002.
- Meng, S., C. X. Zhang, Y. M. Li, and Y. Cao. 2016. “Zhao Z Distinct Effect of pH on N Uptake and Assimilation in Two Conifer Species.” Trees 30: 1607‒1618. doi:10.1007/s00468-016-1394-5.
- Montagnoli, A., R. K. Dumroese, M. Terzaghi, J. R. Pinto, N. Fulgaro, G. S. Scippa, and D. Chiatante. 2018. “Tree Seedling Response to LED Spectra: Implications for Forest Restoration.” Plant Biosystems - an International Journal Dealing with All Aspects of Plant Biology 152: 515‒523. doi:10.1080/11263504.2018.1435583.
- National Forestry Administration of China 1999. “Tree Seedling Quality Grading of Major Species for Afforestation (GB 6000‒1999).” Authorized by Quality and Technology Supervision Bureau of China. April 1, 2000.
- Nosheen, A., H. Yasmin, R. Naz, A. Bano, R. Keyani, and I. Hussain. 2018. “Pseudomonas Putida Improved Soil Enzyme Activity and Growth of Kasumbha under Low Input of Mineral Fertilizers.” Soil Science and Plant Nutrition 64 (4): 520–525. doi:10.1080/00380768.2018.1461002.
- Park, B. B., M. S. Cho, S. W. Lee, R. D. Yanai, and D. K. Lee. 2012. “Minimizing Nutrient Leaching and Improving Nutrient Use Efficiency of Liriodendron Tulipifera and Larix Leptolepis in a Container Nursery System.” New Forests 43: 57–68. doi:10.1007/s11056-011-9266-8.
- Pokharel, P., and S. X. Chang. 2016. “Exponential Fertilization Promotes Seedling Growth by Increasing Nitrogen Retranslocation in Trembling Aspen Planted for Oil Sands Reclamation.” Forest Ecology and Management 372: 35‒43. doi:10.1016/j.foreco.2016.03.034.
- Pokharel, P., W. J. Choi, G. M. Jamro, and S. X. Chang. 2017. “Weed Control Increases Nitrogen Retranslocation and Growth of White Spruce Seedlings on a Reclaimed Oil Sands Soil.” New Forests 48: 699‒717. doi:10.1007/s11056-017-9593-5.
- Riikonen, J. 2016. “Pre-Cultivation of Scots Pine and Norway Spruce Transplant Seedlings under Four Different Light Spectra Did Not Affect Their Field Performance.” New Forests 47: 607‒619. doi:10.1007/s11056-016-9533-9.
- Riikonen, J., N. Kettunen, M. Gritsevich, T. Hakala, L. Särkkä, and R. Tahvonen. 2016. “Growth and Development of Norway Spruce and Scots Pine Seedlings under Different Light Spectra.” Environmental and Experimental Botany 121: 112–120. doi:10.1016/j.envexpbot.2015.06.006.
- Salifu, K. F., and V. R. Timmer. 2003. “Optimizing Nitrogen Loading of Picea Mariana Seedlings during Nursery Culture.” Canadian Journal of Forest Research. Journal Canadien De La Recherche Forestiere 33: 1287–1294. doi:10.1139/x03-057.
- Schott, K. M., B. D. Pinno, and S. M. Landhäusser. 2013. “Premature Shoot Growth Termination Allows Nutrient Loading of Seedlings with an Indeterminate Growth Strategy.” New Forests 44: 635–647. doi:10.1007/s11056-013-9373-9.
- Smirnakou, S., T. Ouzounis, and M. K. Radoglou. 2017. “Continuous Spectrum LEDs Promote Seedling Quality Quality Traits and Performance of Querces Ithaburensis Var.” Macrolepis Frontiers in Plant Science 8: 188.
- Son, K. H., and M. M. Oh. 2013. “Leaf Shape, Growth, and Antioxidant Phenolic Compounds of Two Lettuce Cultivars Grown under Various Combinations of Blue and Red Light-Emitting Diodes.” Hortscience 48: 988–995. doi:10.21273/HORTSCI.48.8.988.
- Taiz, L., and E. Zeiger. 2010. Plant Physiology. 5th ed. Sunderland (MA): Sinauer Associates.
- Terashima, I., T. Fujita, T. Inoue, W. S. Chow, and R. Oguchi. 2009. “Green Light Drives Leaf Photosynthesis More Efficiently than Red Light in Strong White Light: Revisiting the Enigmatic Question of Why Leaves are Green.” Plant & Cell Physiology 50: 684–697. doi:10.1093/pcp/pcp034.
- Timmer, V. R. 1997. “Exponential Nutrient Loading: A New Fertilization Technique to Improve Seedling Performance on Competitive Sites.” New Forests 13: 279‒299. doi:10.1023/A:1006502830067.
- Timmer, V. R., and E. L. Stone. 1978. “Comparative Foliar Analysis of Young Balsam Fir Fertilized with Nitrogen, Phosphorus, Potassium, and Lime.” Soil Science Society of America Journal 42: 125–130. doi:10.2136/sssaj1978.03615995004200010027x.
- Uscola, M., K. F. Salifu, J. A. Oliet, and D. F. Jacobs. 2015. “An Exponential Fertilization Dose-Response Model to Promote Restoration of the Mediterranean Oak Quercus Ilex.” New Forests 46: 795–812. doi:10.1007/s11056-015-9493-5.
- Vitasse, Y., and D. Basler. 2013. “What Role for Photoperiod in the Bud Burst Phenology of European Beech.” European Journal of Forest Research 132: 1‒8. doi:10.1007/s10342-012-0661-2.
- Wallin, E., D. Grans, D. F. Jacobs, A. Lindstrom, and N. Verhoef. 2017. “Short-Day Photoperiods Affect Expression of Genes Related to Dormancy and Freezing Tolerance in Norway Spruce Seedlings.” Annals of Forest Science 74: 59. doi:10.1007/s13595-017-0655-9.
- Wang, X. N., E. Agathokleous, L. Y. Qu, S. Fujita, M. Watanabe, Y. Tamai, Q. Z. Mao, A. Koyama, and T. Koike. 2018. “Effects of Simulated Nitrogen Deposition on Ectomycorrhizae Community Structure in Hybrid Larch and Its Parents Grown in Volcanic Ash Soil: The Role of Phosphorus.” The Science of the Total Environment 618: 905–915. doi:10.1016/j.scitotenv.2017.08.283.
- Wang, Z., L. Y. Ma, Z. K. Jia, H. X. Wei, and J. Duan. 2016. “Interactive Effects of Irrigation and Exponential Fertilization on Nutritional Characteristics in Populus × Euramericana Cv ’74/76ʹ Cuttings in an Open-Air Nursery in Beijing, China.” Journal of Forestry Research 27: 569–582. doi:10.1007/s11676-015-0203-0.
- Wang, Z., Y. Zhao, and H. X. Wei. 2017. “Chitosan Oligosaccharide Addition Affects Current-Year Shoot of Post-Transplant Buddhist Pine (Podocarpus Macrophyllus) Seedlings under Contrasting Photoperiods.” iFor. Biogeosci. Forestry 10: 715‒721.
- Watanabe, M., K. Ryu, K. Kita, K. Takagi, and T. Koike. 2012. “Effect of Nitrogen Load on Growth and Photosynthesis of Seedlings of the Hybrid Larch F1 (Larix Gmelinii Var. Japonica × L. Kaempferi) Grown on Serpentine Soil.” Environmental and Experimental Botany 83: 73‒81. doi:10.1016/j.envexpbot.2012.04.011.
- Wei, H. X., and P. Guo. 2016. “Carbohydrate Metabolism during New Root Growth in Transplanted Larix Oglensis Seedlings: Post-Transplant Response to Nursery-Applied Inorganic Fertilizer and Organic Amendment.” iFor. Biogeosci. Forestry 10: 15‒22.
- Wei, H. X., P. Guo, H. F. Zheng, X. Y. He, P. J. Wang, Z. B. Ren, and C. Zhai. 2017. “Micro-Scale Heterogeneity in Urban Forest Soils Affects Fine Root Foraging by Ornamental Seedlings of Buddhist Pine and Northeast Yew.” Urban Forestry & Urban Greening 28: 63‒72. doi:10.1016/j.ufug.2017.10.006.
- Wei, H. X., J. Ren, and J. H. Zhou. 2013a. “Effect of Exponential Fertilization on Growth and Nutritional Status in Buddhist Pine (Podocarpus Macrophyllus [Thunb.] D. Don) Seedlings Cultured in Natural and Prolonged Photoperiods.” Soil Science and Plant Nutrition 59: 933‒941. doi:10.1080/00380768.2013.864957.
- Wei, H. X., C. Y. Xu, J. Ren, L. Y. Ma, J. Duan, and L. N. Jiang. 2013b. “Newly Transplanted Larix Olgensis Henry Stock with Greater Root Biomass Has Higher Early Nitrogen Flux Rate.” Soil Science and Plant Nutrition 59: 740‒749. doi:10.1080/00380768.2013.816977.
- Xue, L., K. Ogawa, A. Hagihara, S. L. Liang, and J. P. Bai. 1999. “Self-Thinning Exponents Based on the Allometric Model in Chinese Pine (Pinus Tabulaeformis Carr.) And Prince Rupprecht’s Larch (Larix Principis-Rupprechtii Mayr) Stands.” Forest Ecology and Management 117: 87‒93. doi:10.1016/S0378-1127(98)00472-1.
- Zhang, S. X., D. D. Huang, X. Y. Yi, S. Zhang, R. Yao, C. G. Li, A. Z. Liang, and X. P. Zhang. 2016. “Rice Yield Corresponding to the Seedling Growth under Supplemental Green Light in Mixed Light-Emitting Diodes.” Plant Soil and Environment 62: 222–229. doi:10.17221/783/2015-PSE.
- Zhao, Y., Z. Wang, H. X. Wei, Y. J. Bao, and P. Gu. 2017. “Effect of Prolonged Photoperiod on Morphology, Biomass Accumulation and Nutrient Utilization in Post-Transplant Taxus Cuspidate Seedlings.”
- Zhu, K. Y., H. C. Liu, H. X. Wei, J. H. Zhou, Q. C. Zou, G. Y. Ma, and J. Q. Zhang. 2016. “Prediction of Nutrient Leaching from Culture of Containerized Buddhist Pine and Japanese Maple Seedlings Exposed to Extended Photoperiod.” International Journal of Agriculture and Biology 18: 425‒434.