ABSTRACT
Microbial siderophore-chelated Fe(III) is suggested to be an important source of Fe for plants, although it is hardly reduced by plant roots. Here, we investigated the efficacy of the easily reducible artificial microbial siderophore tris[2-{(N-acetyl-N-hydroxy)glycylamino}ethyl]amine (TAGE)-Fe(III) as an alternative Fe source to correct Fe deficiency in rice plants, and compared it to that of the natural siderophore deferoxamine B (DFOB)-Fe(III). We also evaluated the absorption of Fe from TAGE-Fe(III) by the Strategy I-like system of gramineous plants using nicotianamine aminotransferase 1 (naat1) mutant rice, which does not synthesize phytosiderophores. Fe(III)-siderophores were synthesized in vitro. Nipponbare rice and its naat1 mutant were reared in soil and gel cultures to determine Fe availability. Hydroponically grown naat1 mutant seedlings were used for reducibility assays to determine the ability of rice roots to reduce Fe(III) chelated by TAGE or DFOB. The expression of a Fe-deficiency inducible gene was also determined, as well as chlorophyll and Fe concentrations. Reduci bility assays on naat1 mutant seedlings revealed that the reduction level of TAGE-Fe(III) was approximately three times higher than that of DFOB-Fe(III). Application of TAGE-Fe(III) to both culture medium and alkaline soil improved Fe chlorosis, growth, and Fe concentration in both naat1 and wild type plants, whereas application of DFOB-Fe(III) only did so in wild type plants. Easily reducible Fe(III)-chelates such as TAGE-Fe(III) can be a better source of Fe for rice plants than most natural microbial siderophores-Fe(III). Our study also demonstrated that rice plants have the ability to utilize microbial siderophores-Fe(III) as the Fe source through the Strategy I-like Fe acquisition system.
1. Introduction
Iron (Fe) is an essential micronutrient for almost all living organisms, including plants and microbes. It is the most abundant heavy metal in the earth crust; however, its low bioavailability in aerobic calcareous soil restricts the growth of many organisms. To cope with Fe-limited conditions, plants and microorganisms have evolved effective mechanisms to acquire Fe from the environment, including its solubilization, reduction, and absorption (Kobayashi and Nishizawa Citation2012; Ahmed and Holmström Citation2014).
Plants have developed at least two Fe acquisition systems, termed as Strategy I and Strategy II. Strategy I plants, including dicotyledons and non-gramineous monocotyledons, employ the following mechanisms: solubilization of insoluble Fe(III)-hydroxide and Fe(III)-oxide through soil acidification, chelation of Fe by phenolic compounds, reduction of Fe3+ to Fe2+ by plasma membrane-bound ferric reductase and non-membrane-bound agents (e.g. flavins), and Fe2+ uptake by Fe regulated transporter 1 (IRT1) (Kobayashi and Nishizawa Citation2012; Sisó-Terraza et al. Citation2016). Strategy II plants, including Gramineae, solubilize Fe(III) by chelation with phytosiderophores followed by the uptake of phytosiderophores-Fe(III) complexes via yellow stripe-like family transporters. To date, nine different phytosiderophores have been identified (Ueno et al. Citation2007). Although the type of phytosiderophores varies among species, tolerance to Fe deficiency depends on the total amount secreted rather than on the variety of phytosiderophores (Kobayashi, Nakanishi, and Nishizawa Citation2010).
Rice (Oryza sativa L.) is moderately sensitive to Fe deficiency owing to its low phytosiderophore productivity (Hansen et al. Citation2006; Kobayashi, Nakanishi, and Nishizawa Citation2010). Under Fe restriction, rice secretes a single phytosiderophore, 2′-deoxymugineic acid (DMA), which solubilizes Fe(III), and then absorbs DMA-Fe(III) via the yellow stripe-like 15 (OsYSL15) (Inoue et al. Citation2009). In contrast, in flooded paddy soil, where the reduced form of Fe (Fe2+) is abundant and available, rice absorbs Fe2+ mainly through OsIRT1 (Ishimaru et al. Citation2006). Thus, rice is able to acquire Fe through Strategy I processes despite being a Strategy II plant. However, the Strategy I-like system in rice is insufficient to absorb Fe(III) due to loss of function of its ferric reductase (Ishimaru et al. Citation2006). Alternatively, transgenic rice plants with enhanced activity of ferric reductase can successfully reverse their sensitivity to Fe deficiency and grow well in calcareous soil (Ishimaru et al. Citation2007; Masuda et al. Citation2017). In another gramineous, barley (Hordeum vulgare L.), HvIRT1 contributes to manganese (Mn) uptake, but it also shows Fe transport activity in yeast and its transcription is induced by both Fe and Mn deficiencies (Long et al. Citation2018; Pedas et al. Citation2008). Although its contribution is unclear, Fe acquisition by gramineous plants depends to some extent on a Strategy I-like system.
Microorganisms release microbial siderophores under Fe-starvation primarily to solubilize Fe (Schwyn and Neilands Citation1987). Almost 500 compounds have been identified as siderophores, which are divided into three main families depending on their characteristic functional group: hydroxamates (e.g. ferrioxamine B and ferrichrome), catecholates (e.g. enterobactin), and carboxylates (e.g. rhizobactin) (Ahmed and Holmström Citation2014; Jin, Ye, and Zheng Citation2014). There are also certain siderophores that contain more than one kind of functional group (e.g. pyoverdine) (Cornelis Citation2010). The redox potential (e.g. E1/2 = ca. −400 ~ −500 mV vs. normal hydrogen electrode – NHE – for Fe(III)-trihydroxamate siderophores, Hider and Kong Citation2010) of Fe(III) captured by microbial siderophores is too low to be directly reduced by plant roots. Thus, siderophore-Fe(III) is hardly available for plants unless the microbial siderophore is substituted with phytosiderophores. However, this reaction depends on several parameters, including stability constants and concentrations of both microbial siderophores and phytosiderophores, and the pH and redox conditions of the root environment (Ahmed and Holmström Citation2014; Crowley Citation2006). In this Matsumoto et al. (Citation2001), (Citation2004) reported an artificial siderophore, tris[2-{(N-acetyl-N-hydroxy)glycylamino}ethyl]amine (TAGE) with a high stability constant (log β = 28.6) for Fe(III), which is comparable to that of natural siderophores, and a more positive redox potential (E1/2 = −230 mV vs. NHE) than ferric natural siderophore complexes. This redox property of TAGE-Fe(III) indicates that the complex can be easily reduced by weak reductants, such as ascorbate, and potentially enables Strategy I plants to absorb Fe.
Solubilization of Fe by siderophores in the rhizosphere has been proposed to be the key microbial function that favors Fe acquisition by plants (Jin, Ye, and Zheng Citation2014), even though Fe(III) chelated by siderophores exhibits low reducibility. The application of microorganism-derived Fe-siderophores to foliage and roots has been proposed to alleviate Fe chlorosis in an environmentally friendly manner. The reason is that these microbial chelates are more biodegradable than synthetic chelates such as ethylenediamine-N,N′-bis(2-hydroxyphenylacetic acid)-Fe(III) and ethylenediaminetetraacetic acid-Fe(III) (Römheld and Nikolic Citation2007). Hydroponic studies demonstrated that application of Fe-pyoverdine to Arabidopsis thaliana and Solanum lycopersicum roots ameliorates Fe deficiency (Vansuyt et al. Citation2007; Nagata, Oobo, and Aozasa Citation2013). Microbial Fe-siderophores have also been shown to be good Fe sources for gramineous plants, for example, Fe-erobactin for oat (Chen et al. Citation1998), and Fe-rhizoferrin for barley and corn (Yehuda et al. Citation2000). However, the contributions of Strategy II and Strategy I-like systems for Fe acquisition from Fe-siderophores in gramineous plants have not been explored so far.
Therefore, the present study aimed to (i) evaluate the efficacy of TAGE-Fe(III) as an alternative Fe source to correct Fe deficiency, (ii) compare the effect of TAGE-Fe(III) administration to that of a natural siderophore-Fe(III), and (iii) assess the availability of Fe provided as TAGE-Fe(III) and absorbed by Strategy I-like system of gramineous plants. To do so, we used a mutant rice strain that does not synthesize phytosiderophores. Moreover, we also discuss possible modifications in the structure of the artificial siderophore toward general use.
2. Materials and methods
2.1. In vitro synthesis of Fe(III)-siderophores
The artificial siderophore TAGE was synthesized according to the previously published method (Matsumoto et al. Citation2004). An aqueous solution (30 mL) containing 0.80 mmol of TAGE was mixed with an ethyl acetate solution (30 mL) of tris(acetylacetonato)Fe(III) (Fe(acac)3; 0.80 mmol), and then the two-layered solution was vigorously stirred for 6 h at room temperature. The aqueous layer was separated from the reaction solution. The resulting organic layer was extracted with water (20 mL). Both aqueous layers were combined and washed with ethyl acetate (30 mL, three times). The aqueous solution was dried and a small amount of diethyl ether was added to the residue. The deep red precipitate that was formed was then filtered, and the filtrate was washed with a small volume of methanol and vacuum-dried (0.65 mmol, yield 81%).
The Fe(III) complex containing the natural siderophore deferoxamine B (DFOB), was prepared according to a procedure similar to that used for TAGE-Fe(III), but using DFOB mesylate instead of TAGE. DFOB mesylate was purchased from Sigma-Aldrich. An aqueous solution (15 mL) containing 0.50 mmol of DFOB mesylate was mixed with an ethyl acetate solution (15 mL) of Fe(acac)3 (0.50 mmol), and then the two-layered solution was vigorously stirred for 4.5 h at room temperature. The aqueous layer was separated from the reaction solution, washed with ethyl acetate (15 mL, three times), and then dried. A small amount of diethyl ether was added to the residue, and the deep red precipitate that was formed was filtered and vacuum-dried (0.44 mmol, yield 88%).
2.2. Plant materials and growth conditions
The availability of Fe in rice plants treated with Fe(III)-siderophores was examined using gel and soil cultures. Rice seeds of the Nipponbare cultivar and its nicotianamine aminotransferase 1 (naat1) mutant, which expresses the fourth-exon-deleted NAAT1 mRNA and is sensitive to Fe deficiency due to the lack of phytosiderophore synthesis (Cheng et al. Citation2007), were surface sterilized with 70% ethanol for 1 min followed by 2.5% (v/v) NaHClO for 20 min. The seeds were thoroughly rinsed with sterile distilled water and put on agar plates containing half strength Murashige and Skoog (MS, pH 5.8) medium (Murashige and Skoog Citation1962) without any Fe source. The seeds were germinated for 2 days at 30°C in the dark. Seedlings were then transferred to pots (seven plants/pot), which were 9–11 cm in diameter and 14 cm tall, filled with 150 mL MS agar medium with or without supplementation of 100 µM TAGE-Fe(III) or DFOB-Fe(III). The pots were maintained in a controlled growth chamber (CFH-415, TOMY, Tokyo, Japan; 30°C, 14 h, light/25°C, 10 h, dark) for 8 or 12 days for wild type or naat1 mutant plants, respectively.
For soil culture, germinating naat1 seeds were transferred to pots (three plants/pot), which were 4.5–6 cm in diameter and 5 cm tall, filled with 100 g shelly fossil soil (pH 9.1, 15 g Fe kg−1 soil dry weight) purchased from Nihonkai Hiryo Co., Ltd., Japan. The soil was fertilized with N-P-K fertilizer (6–40-9; MAGAMP K, HYPONeX, Osaka, Japan) at 1 g kg−1 soil dry weight, and supplemented with 35 mL of 1 mM TAGE-Fe(III) or DFOB-Fe(III) (approximately 20 mg Fe kg−1 soil dry weight). Soil without Fe supplementation was used as control. Plants were grown for 23 days under upland conditions in the growth chamber, and the soil was watered daily with tap water. After this period, shoots and roots were separated, washed twice with distilled water, and Fe concentration was determined by atomic absorption spectrometry as described in Section 2.5.
2.3. Reducibility assay
To investigate the reducibility of Fe(III) chelated by TAGE or DFOB in rice roots, seedlings were grown hydroponically with half strength Kimura B nutrient solution containing: 0.28 mM MgSO4, 0.18 mM (NH4)2SO4, 0.18 mM Ca(NO3)2, 90 µM KNO3, 90 µM KH2PO4, 0.2 µM CuSO4, 20 µM Fe(III)-EDTA, 0.3 µM H3BO3, 0.5 µM MnCl2, 0.4 µM ZnSO4, and 0.2 µM (NH4)6Mo7O24. The pH of the nutrient solution was adjusted to 5.4 with 1 M NaOH and 1 M HCl. The nutrient solution was replenished every two days. The roots of 16-day-old seedlings were rinsed with nutrient solution without Fe for 1 h, and used for Fe(III)-chelate reducibility assays according to Romera et al. (Citation1996), with some modifications. Briefly, roots were exposed to 40 mL of assay solution [0.2 mM CaSO4, 5 mM 2-morpholinoethanesulfonic acid at pH 5.5, 0.1 mM TAGE-Fe(III) or DFOB-Fe(III), and 0.2 mM bathophenanthroline disulfonic acid (BPDS)] for 3 h at 30°C. The solution was read at 535 nm using a spectrophotometer (V-630Bio, Jasco, Tokyo, Japan). The BPDS-Fe(II) concentration was calculated using the extinction coefficient of 22.14 mM−1 cm−1. The fresh weight of the roots was also recorded.
2.4. Determination of chlorophyll concentration
Shoots were ground in 80% (v/v) acetone solution with quartz sand and Na2CO3. The extraction was repeated until no green material was left in the residue. Total chlorophyll concentration was determined as described by Arnon (Citation1949). In the youngest expanded leaf blades, chlorophyll concentration was also measured using a chlorophyll meter (SPAD-502Plus, Konica Minolta, Tokyo, Japan).
2.5. Determination of fe concentration
To determine Fe concentrations by atomic absorption spectrometry (AA-6800, Shimadzu, Kyoto, Japan), harvested samples were dried at 70°C, weighed, digested with 60% (v/v) HNO3 at 140°C, and then diluted with distilled water to appropriate concentrations.
2.6. Expression of the Fe deficiency inducible gene OsYSL15
To estimate Fe status in plants, the expression of the Fe deficiency inducible gene OsYSL15 (Acc. No. Os02g0650300, http://rapdb.dna.affrc.go.jp/), was measured. Wild type rice was grown on MS medium containing varying concentrations (0, 50, and 100 µM) of TAGE- Fe(III) or DFOB-Fe(III) for 8 days. Total RNA was then extracted from roots with the Plant total RNA Purification Kit (GeneMark, Atlanta, GA, USA), treated with DNase I, and converted to cDNA using ReverTra Ace (Toyobo, Osaka, Japan). The expression of OsYSL15 was analyzed using quantitative RT-PCR with the primers 5′-ACTGGTTACCCTGCAAACATAC-3′ and 5′-GCAATGATGCTTAGCAAGAAG-3′. Histone H3 (Acc. No. Os01g0907400) was selected as the internal control and its expression was evaluated using the primers 5′-GGTCAACTTGTTGATTCCCCTCT-3′ and 5′-AACCGCAAAATCCAAAGAACG-3′. Quantitative RT-PCR was conducted using a KOD SYBR qPCR mix (Toyobo) on a Prism 7300 Real-time PCR System (Applied Biosystems, Foster City, CA, USA).
2.7. Statistical analysis
Data were analyzed using the Student’s t-test or Tukey’s test. Significance (P < 0.05) is indicated by asterisks or by different letters.
3. Results and discussion
In the present study, we used naat1 mutant rice to evaluate the efficacy of TAGE-Fe(III) as the Fe source. Nicotianamine aminotransferase is required to transfer the amino group from nicotianamine to form the 3′′-oxo intermediate of the phytosiderophore DMA (Shojima et al. Citation1990). Disruption of NAAT1 in rice results in Fe deficiency in aerobic soil conditions owing to the lack of DMA synthesis (Cheng et al. Citation2007). This phenotype was useful to avoid chelation of Fe(III) with DMA so that we could properly evaluate the availability of TAGE-Fe(III) for Strategy I processes in rice plants. The reducibility of Fe(III)-chelates by rice roots showed that the rate of TAGE-Fe(III) reduction was approximately three times higher than that of DFOB-Fe(III) (). Although reductase activity of rice roots is low (Ishimaru et al. Citation2011), TAGE-Fe(III) can be reduced by the activity of phenolic compounds, such as protocatechuic acid and caffeic acid, which are secreted by rice roots to utilize the Fe precipitated in the root apoplast (Bashir et al. Citation2011; Ishimaru et al. Citation2011) and have the ability to reduce Fe(III) to Fe(II) in addition to chelate and solubilize Fe(III) (Yoshino and Murakami Citation1998; Römheld and Nikolic Citation2007).
Figure 1. Reducibility of Fe from Fe(III)-chelates by rice roots. Roots of naat1 mutants grown hydroponically for 16 days were used. The reducibility was examined with the BPDS assay. Data are presented as means ± standard deviations (n = 3). * P < 0.05, Student’s t-test
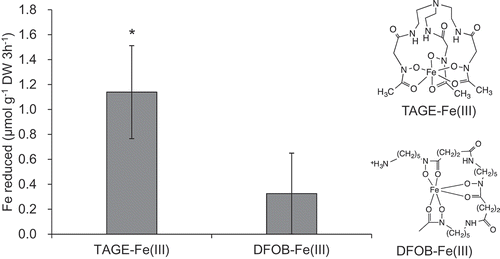
Application of TAGE-Fe(III) to the medium ameliorated the chlorosis and growth of naat1 mutant rice (). Shoot chlorophyll concentration in the TAGE-Fe(III) treatment was three times higher than that in the DFOB-Fe(III) and -Fe treatments (). The dry weight of shoots and roots in the TAGE-Fe(III) treatment was also higher than that in the DFOB-Fe(III) treatment, while there was no significant difference between -Fe and DFOB-Fe(III) treatments (). Iron concentration, in both shoot and root, was several times higher under the TAGE-Fe(III) treatment than under the other treatments (). Moreover, total Fe amount in plants treated with TAGE-Fe(III) was approximately seven times higher than that in plants under the other treatments (). These results indicate that Fe derived from TAGE-Fe(III) is easily absorbed by rice via the Strategy I pathway in comparison with Fe derived from DFOB-Fe(III). Once Fe3+ was reduced from TAGE-Fe(III), probably by phenolics, Fe2+ might have entered root cells through OsIRT1 (Ishimaru et al. Citation2006). Although TAGE-Fe(III) might have been absorbed by DMA-Fe(III) transporters, such as OsYSL15, the contribution of such transporters to microbial siderophore-Fe(III) uptake remains unclear.
Figure 2. Effect of TAGE-Fe(III) on the growth and chlorophyll concentration of naat1 mutant rice. Mutant rice was cultured in nutrient medium with or without supplementation of TAGE-Fe(III) or DFOB-Fe(III) for 12 days. (a) Plant growth, (b) chlorophyll concentration in shoots, and (c) dry weight of shoots and roots. Data are presented as means ± standard deviations (n = 4). Different letters indicate significant differences (P < 0.05) using Tukey’s test
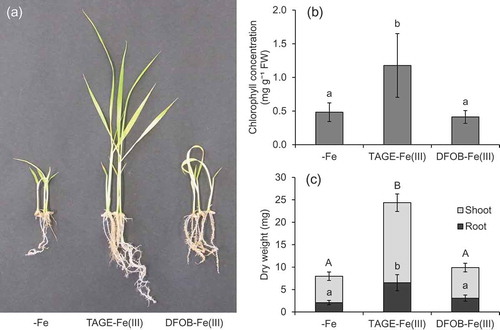
Figure 3. Effect of TAGE-Fe(III) on Fe accumulation in naat1 mutant rice. Mutant rice was cultured in nutrient medium with or without supplementation of TAGE-Fe(III) or DFOB-Fe(III) for 12 days. Iron concentration in shoots (a) and roots (b), and the amount of Fe accumulated (c) are shown. Data are presented as means ± standard deviations (n = 4). Different letters indicate significant differences (P < 0.05) using Tukey’s test
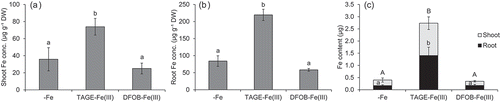
The TAGE-Fe(III) treatment also improved Fe chlorosis in wild type plants (). In contrast to naat1 mutants, chlorophyll concentration in wild type rice was higher under the DFOB-Fe(III) treatment than under the -Fe treatment, but lower than that under the TAGE-Fe(III) treatment (). Although it was not significantly different, dry weight tended to increase in the presence of TAGE-Fe(III) (). Shoot Fe concentration in the TAGE-Fe(III) treatment was the highest, but that in the DFOB-Fe(III) treatment was also significantly higher than that in the -Fe treatment (), suggesting that the ligand of Fe(III) was substituted from DFOB to DMA, followed by Fe uptake in the form of DMA-Fe(III) through the Strategy II pathway. In wild type rice, root Fe concentration in the TAGE-Fe(III) treatment was higher than that in the other treatments (). Total Fe content in shoots and roots was highest in the TAGE-Fe(III) treatment, followed by the DFOB-Fe(III) treatment, and lowest in the -Fe treatment (). These results suggest that Fe from TAGE-Fe(III) is also more available than that from DFOB-Fe(III) in rice, even in the presence of the Strategy II system. This hypothesis was supported by gene expression analysis; 50 µM TAGE-Fe(III) treatment was sufficient to decrease the expression of the Fe deficiency-inducible gene OsYSL15 (Inoue et al. Citation2009) in relation to that in the absence of Fe, whereas 100 µM was required to achieve similar downregulation in the DFOB-Fe(III) treatment ().
Figure 4. Effect of TAGE-Fe(III) on the growth and chlorophyll concentration of wild type rice plants. Wild type rice was cultured in nutrient medium with or without supplementation of TAGE-Fe(III) or DFOB-Fe(III) for 8 days. (a) Plant growth, (b) chlorophyll concentration in shoots, and (c) dry weight of shoots and roots. Data are presented as means ± standard deviations (n = 4). Different letters indicate significant differences (P < 0.05) using Tukey’s test. n.s., not significant
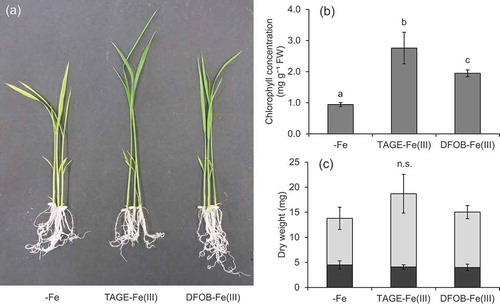
Figure 5. Effect of TAGE-Fe(III) on Fe accumulation in wild type rice plants. Wild type rice was cultured in nutrient medium with or without supplementation of TAGE-Fe(III) or DFOB-Fe(III) for 8 days. Iron concentration in shoots (a) and roots (b), and amount of Fe accumulated (c) are shown. Data are presented as means ± standard deviations (n = 4). Different letters indicate significant differences (P < 0.05) using Tukey’s test
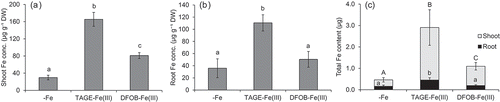
Figure 6. Effect of TAGE-Fe(III) on Fe status in wild type rice plants. Wild type rice plants were treated with varying concentrations (0, 50, and 100 µM) of TAGE-Fe(III) or DFOB-Fe(III) for 8 days. Relative expression levels of yellow stripe-like 15 (OsYSL15) compared with plants grown under 100 µM TAGE-Fe(III) treatment are shown. Histone was used as an internal control. Data are presented as means ± standard deviations (n = 3). Different letters indicate significant differences (P < 0.01) using Tukey’s test
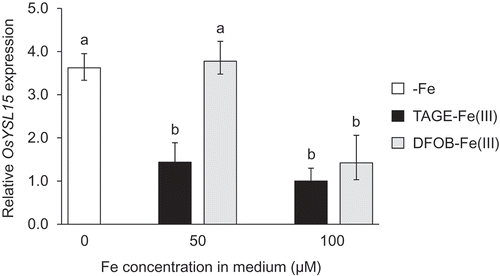
Although TAGE-Fe(III) application significantly enhanced the growth of naat1 mutant plants, these were still chlorotic even when Fe concentration in shoots exceeded the critical level for deficiency (< 50 μg g−1, Dobermann and Fairhurst Citation2000). This might have been caused by a secondary effect of NAAT1 disruption, which is the decreased intercellular Fe mobility due to the lack of DMA. In the root xylem, Fe is precipitated as an insoluble form unless it is chelated by organic ligands (Green and Rogers Citation2004). In rice, disruption of ferric reductase defective-like 1 (OsFRDL1), coding a citric acid transporter, resulted in the precipitation and accumulation of Fe in the root’s stele (Yokosho et al. Citation2009). In previous studies, DMA-Fe(III) was detected in xylem sap (Ariga et al. Citation2014) and DMA concentration in xylem sap increased in response to Fe deficiency (Kakei et al. Citation2009). These findings suggest that DMA, as well as citric acid, intermediates the solubilization and long-distance transport of Fe in the xylem. In fact, in the present study, about half of the Fe content in whole naat1 mutant plants was located in the roots (), whereas a large part of the Fe content of wild type plants was located in the shoots (), suggesting that Fe may accumulate in xylem vessel in the absence of DMA.
The availability of Fe from TAGE-Fe(III) in soil was examined under upland conditions to avoid the reduction of Fe and its release as Fe2+ caused by flooding. Similar to agar medium assays, Fe chlorosis and growth were ameliorated more effectively by TAGE-Fe(III) than by DFOB-Fe(III) (), although shoot Fe concentration in the TAGE-Fe(III) treatment was still below the deficiency threshold (). The amount of Fe tended to be higher in the TAGE-Fe(III) treatment than in the control and DFOB-Fe(III) treatment (). These results suggest that TAGE-Fe(III) can be a good Fe source for Strategy I-like processes in rice cultured in alkaline soil, and that Fe availability from TAGE complexes needs to be improved. Prior to its absorption by the roots, Fe2+ must be released from TAGE-Fe(II), which is produced by the reduction of TAGE-Fe(III). Because TAGE-Fe(II) is still relatively stable (log β = 12.4, Matsumoto et al. Citation2001), changing the structure of the artificial microbial siderophore-Fe(III) to decrease its affinity for Fe(II) and increase Fe releasability is required for general use. This could be achieved by, for example, the elongation of the methylene length between the amide and hydroxamate groups of TAGE because that breaks the intramolecular hydrogen bonding networks to stabilize TAGE-Fe (Matsumoto et al. Citation2004).
Figure 7. Effect of TAGE-Fe(III) on the growth, chlorophyll concentration, and Fe accumulation of naat1 mutant rice in alkaline soil. Mutant rice was grown for 23 days under upland conditions. (a) Plant growth, (b) chlorophyll concentration (SPAD value), (c) shoot dry weight, (d) shoot Fe concentration, and (e) amount of Fe in shoots. Data are presented as means ± standard deviations (n = 4). Different letters indicate significant differences (P < 0.05) using Tukey’s test. n.s., not significant
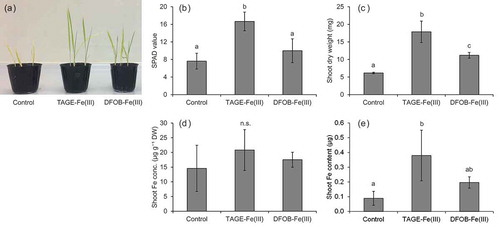
In conclusion, the artificial microbial siderophore TAGE-Fe(III) can be readily reduced by rice roots followed by Fe uptake and improve Fe status more effectively than the natural microbial siderophore DFOB-Fe(III). Our findings also indicate that rice plants have ability to utilize microbial siderophore-Fe(III), or at least TAGE-Fe(III), as the Fe source through the Strategy I-like Fe acquisition system. Although there is room for improvement of their structures, this study demonstrates that artificial microbial siderophores-Fe(III) with high reducibility can be effective Fe sources for plants.
Acknowledgments
We thank Yuta Tsunemitsu and Toru Terauchi for their technical assistance.
Disclosure statement
No potential conflict of interest was reported by the authors.
Additional information
Funding
References
- Ahmed, E., and S. J. Holmström. 2014. “Siderophores in Environmental Research: Roles and Applications.” Microbial Biotechnology 7: 196–208. doi:10.1111/1751-7915.12117.
- Ariga, T., K. Hazama, S. Yanagisawa, and T. Yoneyama. 2014. “Chemical Forms of Iron in Xylem Sap from Graminaceous and Non-graminaceous Plants.” Soil Science and Plant Nutrition 60: 460–469. doi:10.1080/00380768.2014.922406.
- Arnon, D. I. 1949. “Copper Enzymes in Isolated Chloroplasts. Polyphenoloxidase in Beta Vulgaris.” Plant Physiology 24: 1–15. doi:10.1104/pp.24.1.1.
- Bashir, K., Y. Ishimaru, H. Shimo, Y. Kakei, T. Senoura, R. Takahashi, Y. Sato, et al. 2011. “Rice Phenolics Efflux Transporter 2 (PEZ2) Plays an Important Role in Solubilizing Apoplasmic Iron.” Soil Science and Plant Nutrition 57: 807–813. doi:10.1080/00380768.2011.637305.
- Chen, L. M., W. A. Dick, J. G. Streeter, and H. A. J. Hoitink. 1998. “Fe Chelates from Compost Microorganisms Improve Fe Nutrition of Soybean and Oat.” Plant and Soil 200: 139–147. doi:10.1023/A:1004375430762.
- Cheng, L., F. Wang, H. Shou, F. Huang, L. Zheng, F. He, J. Li, et al. 2007. “Mutation in Nicotianamine Aminotransferase Stimulated the Fe(II) Acquisition System and Led to Iron Accumulation in Rice.” Plant Physiology 145: 1647–1657. doi:10.1104/pp.107.107912.
- Cornelis, P. 2010. “Iron Uptake and Metabolism in Pseudomonads.” Applied Microbiology and Biotechnology 86: 1637–1645. doi:10.1007/s00253-010-2550-2.
- Crowley, D. A. 2006. “Microbial Siderophores in the Plant Rhizosphere.” In Iron Nutrition in Plants and Rhizospheric Microorganisms, edited by L. L. Barton and J. Abadía, 169–189. Netherlands: Springer.
- Dobermann, A., and T. H. Fairhurst. 2000. Rice: Nutritional Disorder & Nutrient Management. Potash & Phosphate Institute (PPI), Potash & Phosphate Institute of Canada (PPIC) and International Rice Research Institute (IRRI), Singapore and Los Baños.
- Green, L., and E. E. Rogers. 2004. “FRD3 Controls Iron Localization in Arabidopsis.” Plant Physiology 136: 2523–2531. doi:10.1104/pp.104.045633.
- Hansen, N. C., B. G. Hopkins, J. W. Ellsworth, and V. D. Jolley. 2006. “Iron Nutrition in Field Crops.” In Iron Nutrition in Plants and Rhizospheric Microorganisms, edited by L. L. Barton and J. Abadia, 23–59. Dordrecht: Springer.
- Hider, R. C., and X. Kong. 2010. “Chemistry and Biology of Siderophores.” Natural Product Reports 27: 637–657. doi:10.1039/b906679a.
- Inoue, H., T. Kobayashi, T. Nozoye, M. Takahashi, Y. Kakei, K. Suzuki, M. Nakazono, H. Nakanishi, S. Mori, and N. K. Nishizawa. 2009. “Rice OsYSL15 Is an Iron-regulated Iron (iii)-deoxymugineic Acid Transporter Expressed in the Roots and Is Essential for Iron Uptake in Early Growth of the Seedlings.” Journal of Biological Chemistry 284: 3470–3479. doi:10.1074/jbc.M806042200.
- Ishimaru, Y., Y. Kakei, H. Shimo, K. Bashir, Y. Sato, Y. Sato, N. Uozumi, H. Nakanishi, and N. K. Nishizawa. 2011. “A Rice Phenolic Efflux Transporter Is Essential for Solubilizing Precipitated Apoplasmic Iron in the Plant Stele.” Journal of Biological Chemistry 286: 24649–24655. doi:10.1074/jbc.M111.221168.
- Ishimaru, Y., S. Kim, T. Tsukamoto, H. Oki, T. Kobayashi, S. Watanabe, S. Matsuhashi, et al. 2007. “Mutational Reconstructed Ferric Chelate Reductase Confers Enhanced Tolerance in Rice to Iron Deficiency in Calcareous Soil.” Proceedings of the National Academy of Sciences of the United States of America 104: 7373–7378. doi:10.1073/pnas.0610555104.
- Ishimaru, Y., M. Suzuki, T. Tsukamoto, K. Suzuki, M. Nakazono, T. Kobayashi, Y. Wada, et al. 2006. “Rice Plants Take up Iron as an Fe3+-phytosiderophore and as Fe2+.” The Plant Journal 45: 335–346. doi:10.1111/j.1365-313X.2005.02624.x.
- Jin, C. W., Y. Q. Ye, and S. J. Zheng. 2014. “An Underground Tale: Contribution of Microbial Activity to Plant Iron Acquisition via Ecological Processes.” Annals of Botany 113: 7–18. doi:10.1093/aob/mct249.
- Kakei, Y., I. Yamaguchi, T. Kobayashi, M. Takahashi, H. Nakanishi, T. Yamakawa, and N. K. Nishizawa. 2009. “A Highly Sensitive, Quick, and Simple Quantification Method for Nicotianamine and 2′-deoxymugineic Acid from Minimum Samples Using LC/ESI-TOF-MS Achieves Functional Analysis of These Components in Plants.” Plant and Cell Physiology 50: 1988–1993. doi:10.1093/pcp/pcp141.
- Kobayashi, T., H. Nakanishi, and N. K. Nishizawa. 2010. “Recent Insights into Iron Homeostasis and Their Application in Graminaceous Crops.” Proceedings of the Japan Academy, Series B 86: 900–913. doi:10.2183/pjab.86.900.
- Kobayashi, T., and N. K. Nishizawa. 2012. “Iron Uptake, Translocation, and Regulation in Higher Plants.” Annual Review of Plant Biology 63: 131–152. doi:10.1146/annurev-arplant-042811-105522.
- Long, L., D. P. Persson, F. Duan, K. Jørgensen, L. Yuan, J. K. Schjoerring, and P. R. Pedas. 2018. “The Iron-regulated Transporter 1 Plays an Essential Role in Uptake, Translocation and Grain-loading of Manganese, but Not Iron, in Barley.” New Phytologist 217: 1640–1653. doi:10.1111/nph.14930.
- Masuda, H., E. Shimochi, T. Hamada, T. Senoura, T. Kobayashi, M. S. Aung, Y. Ishimaru, Y. Ogo, H. Nakanishi, and N. K. Nishizawa. 2017. “A New Transgenic Rice Line Exhibiting Enhanced Ferric Iron Reduction and Phytosiderophore Production Confers Tolerance to Low Iron Availability in Calcareous Soil.” PloS One 12: e0173441. doi:10.1371/journal.pone.0173441.
- Matsumoto, K., T. Ozawa, K. Jitsukawa, H. Einaga, and H. Masuda. 2001. “Crystal Structure and Redox Behavior of a Novel Siderophore Model System: A trihydroxamato-iron(III) Complex with Intra- and Interstrand Hydrogen Bonding Networks.” Inorganic Chemistry 40: 190–191. doi:10.1021/ic0007411.
- Matsumoto, K., T. Ozawa, K. Jitsukawa, and H. Masuda. 2004. “Synthesis, Solution Behavior, Thermal Stability, and Biological Activity of an Fe(III) Complex of an Artificial Siderophore with Intramolecular Hydrogen Bonding Networks.” Inorganic Chemistry 43: 8538–8546. doi:10.1021/ic048761g.
- Murashige, T., and F. Skoog. 1962. “A Revised Medium for Rapid Growth and Bio Assays with Tobacco Tissue Cultures.” Physiologia Plantarum 15: 473–479. doi:10.1111/j.1399-3054.1962.tb08052.x.
- Nagata, T., T. Oobo, and O. Aozasa. 2013. “Efficacy of a Bacterial Siderophore, Pyoverdine, to Supply Iron to Solanum Lycopersicum Plants.” Journal of Bioscience and Bioengineering 115: 686–690. doi:10.1016/j.jbiosc.2012.12.018.
- Pedas, P., C. K. Ytting, A. T. Fuglsang, T. P. Jahn, J. K. Schjoerring, and S. Husted. 2008. “Manganese Efficiency in Barley: Identification and Characterization of the Metal Ion Transporter HvIRT1.” Plant Physiology 148: 455–466. doi:10.1104/pp.108.118851.
- Romera, F. J., R. M. Welch, W. A. Norvell, and S. C. Schaefer. 1996. “Iron Requirement for and Effects of Promoters and Inhibitors of Ethylene Action on Stimulation of Fe(III)-chelate Reductase in Roots of Strategy I Species.” BioMetals 9: 45–50. doi:10.1007/BF00188089.
- Römheld, V., and M. Nikolic. 2007. “Iron.” In Handbook of Plant Nutrition, edited by A. V. Barker and D. J. Pilbeam, 351–374. Boca Raton: CRC Press.
- Schwyn, B., and J. B. Neilands. 1987. “Universal Chemical Assay for the Detection and Determination of Siderophores.” Analytical Biochemistry 160: 47–56. doi:10.1016/0003-2697(87)90612-9.
- Shojima, S., N. K. Nishizawa, S. Fushiya, S. Nozoe, T. Irifune, and S. Mori. 1990. “Biosynthesis of Phytosiderophores: In Vitro Biosynthesis of 2ʹ-deoxymugineic Acid from L-methionine and Nicotianamine.” Plant Physiology 93: 1497–1503. doi:10.1104/pp.93.4.1497.
- Sisó-Terraza, P., J. J. Rios, J. Abadía, A. Abadía, and A. Álvarez-Fernández. 2016. “Flavins Secreted by Roots of Iron-deficient Beta Vulgaris Enable Mining of Ferric Oxide via Reductive Mechanisms.” New Phytologist 209: 733–745. doi:10.1111/nph.13633.
- Ueno, D., A. D. Rombolà, T. Iwashita, K. Nomoto, and J. F. Ma. 2007. “Identification of Two New Phytosiderophores Secreted by Perennial Grasses.” New Phytologist 174: 304–310. doi:10.1111/j.1469-8137.2007.02056.x.
- Vansuyt, G., A. Robin, J. F. Briat, C. Curie, and P. Lemanceau. 2007. “Iron Acquisition from Fe-pyoverdine by Arabidopsis Thaliana.” Molecular Plant-Microbe Interactions 20: 441–447. doi:10.1094/MPMI-20-4-0441.
- Yehuda, Z., M. Shenker, Y. Hadar, and Y. Chen. 2000. “Remedy of Chlorosis Induced by Iron Deficiency in Plants with the Fungal Siderophore Rhizoferrin.” Journal of Plant Nutrition 23: 1991–2006. doi:10.1080/01904160009382160.
- Yokosho, K., N. Yamaji, D. Ueno, N. Mitani, and J. F. Ma. 2009. “OsFRDL1 Is a Citrate Transporter Required for Efficient Translocation of Iron in Rice.” Plant Physiology 149: 297–305. doi:10.1104/pp.108.128132.
- Yoshino, M., and K. Murakami. 1998. “Interaction of Iron with Polyphenolic Compounds: Application to Antioxidant Characterization.” Analytical Biochemistry 257: 40–44. doi:10.1006/abio.1997.2522.