ABSTRACT
Redox cycle of iron (Fe) is the central process in the biogeochemistry of paddy field soil. Although Fe(II)-oxidizing process is mediated by both abiotic and biotic reactions, microorganisms involved in the process have not been well studied in paddy field soil. The present study investigated the community structure of microaerophilic Fe(II)-oxidizing bacteria (FeOB) in the family Gallionellaceae in the plow layer of paddy fields located in the central (Anjo) and northeastern (Omagari) Japan since the members in the family are the typical FeOB in circumneutral freshwater environments and possibly have the significant role for Fe(II) oxidation in paddy field soils. A primer set targeting 16S rRNA gene of Gallionella-related FeOB was newly designed for the polymerase chain reaction-denaturing gradient gel electrophoresis (PCR-DGGE) and quantitative PCR (qPCR) analyses. DGGE analysis showed significant differences in the band patterns between the field sites. Besides, minor differences were observed in the patterns between the soil depths (0–1 cm and below 1 cm) in the Anjo field, while the patterns were relatively stable in the Omagari field during the annual rice cultivation practices. In total 54 bands were sequenced and clustered into 20 operational taxonomic units (OTUs) on the basis of the 97% similarity. Eighteen out of twenty OTUs (50 of 54 bands) were affiliated within the FeOB cluster of Gallionellaceae, some of which were clustered with known microaerophilic FeOB, Ferrigenium kumadai, Ferriphaselus amnicola, ‘Sideroxydans lithotrophicus’ and ‘S. paludicola’. The number of the 16S rRNA gene copies was 105–107 and 106–108 copies g−1 dried soil in the two paddy fields and negatively correlated to the contents of acetate-extractable Fe(II) in the soils during the rice cultivation period. These results suggested inhabitance of considerable number of diverse Gallionella-related FeOB and their potential involvement in the Fe(II)-oxidizing process of soil, especially during the rice cultivation period in the paddy field soils.
1. Introduction
Irrigated paddy field is flooded during rice cultivation, while the field is drained during the fallow or upland cropping periods, resulting that moisture and redox statuses of the soil drastically fluctuate in the annual cycle of the field operations. Inorganic substances (nitrate, manganese, iron, sulfate and CO2) are sequentially reduced under flooded, anoxic soil conditions because anaerobic microorganisms utilize them as electron acceptors in substitution for O2. Meanwhile, the reduced substances are re-oxidized at certain sites of the flooded soil, e.g., a few millimeters of the surface soil layer and some parts of rhizosphere soil, and under drained, oxic soil conditions.
Redox cycle of iron (Fe) is the central process in the biogeochemistry of paddy field soil and significantly affects the dynamics of organic and inorganic substances in the soil (Liesack, Schnell, and Revsbech Citation2000; Kyuma Citation2004). Fluctuation of redox status of Fe in the repeated cycles of flooding and draining affects dynamics and stability of dissolved organic matter in paddy field soil (Kögel-Knabner et al. Citation2010). Fe(III)-phosphate and phosphate occluded with hydrated Fe(III) oxides are the important sources for the plant-available phosphate in flooded paddy field soil (Kyuma Citation2004). Production of methane, a greenhouse gas, is suppressed in flooded paddy field soil during the Fe reduction process because the reaction of Fe reduction is thermodynamically more favorable than methanogenesis (Conrad Citation2002).
Besides, Fe dynamics in paddy field soil influences the productivity and quality of rice and environmental protection. For example, ‘Akiochi’ symptom of rice, which is attributed to toxicity of hydrogen sulfide produced in the process of sulfate reduction and some nutrient deficiencies, often emerges in Fe-deficient and leached paddy fields (Kyuma Citation2004). Fe toxicity of rice, observed as bronzing of rice leaves, frequently emerges in Fe-rich, strongly weathered lowland area and acid sulfate soil area because a massive amount of Fe(II) ion is released into the soil water and rice plant absorbs the Fe(II) excessively (Becker and Asch Citation2005). Fe(III) plaques precipitated on rice roots affect the uptake of arsenic by rice plants (Meharg Citation2004; Syu et al. Citation2013).
Reduction and oxidation processes of Fe are mediated by both abiotic and biotic reactions (Melton et al. Citation2014). In paddy field soil, Fe(III) reduction is predominantly driven via biologically mediated processes (Takai and Kamura Citation1966). On the other hand, it had been presumed that main process of Fe(II) oxidation is attributed to abiotic oxidation by molecular O2 probably as the half-life of Fe(II) in the abiotic oxidation is very short (<1 min) in water under an oxygenated, circumneutral condition (Emerson, Fleming, and McBeth Citation2010). Therefore, significance of biotic Fe(II)-oxidizing reaction has been overlooked in the biogeochemistry of paddy field soil. Only some studies reported anaerobic nitrate-dependent Fe(II) oxidation (Ratering and Schnell Citation2001; Li et al. Citation2016) and the number of microaerophilic FeOB estimated by using the MPN method (Chen et al. Citation2008) in a paddy field soil.
Nowadays, FeOB are classified into four physiological groups; (i) acidophilic, aerobic FeOB, (ii) neutrophilic, aerobic FeOB, which are also known as microaerophilic FeOB, (iii) neutrophilic, anaerobic (nitrate-dependent) FeOB and (iv) anaerobic photosynthetic FeOB (Hedrich, Schlömann, and Johnson Citation2011). Gallionella ferruginea (Ehrenberg Citation1836) and Leptothrix ochracea (Roth Citation1797; Kützing Citation1843) are the typical microaerophilic FeOB in freshwater environments, which have been recognized through the ages, while several novel microaerophilic FeOB have also been discovered in the past 20 years (Emerson, Fleming, and McBeth Citation2010; Hedrich, Schlömann, and Johnson Citation2011; Kato et al. Citation2014). In addition, microbial involvement in the cryptic Fe cycle in environments has been recently spotlighted (Kappler and Bryce Citation2017). In our previous studies, we showed that microbial colonies, in which Fe oxides were deposited, were formed on the surface of incubated paddy soils under the microoxic circumstances (Watanabe et al. Citation2013). These colonies were predominated by microaerophilic Fe(II)-oxidizing bacteria (FeOB) related to G. ferruginea and ‘Sideroxydans’ spp. in the family Gallionellaceae of the Betaproteobacteria (Watanabe et al. Citation2013). A novel microaerophilic FeOB of the family Gallionellaceae, Ferrigenium kumadai An22, isolated from the Fe oxides-deposited colony was recently described (Khalifa et al. Citation2018). These studies evidenced the inhabitance of microaerophilic FeOB, especially Gallionella-related FeOB, in paddy field soil and strongly suggested their involvement in the Fe(II)-oxidizing process under certain soil conditions. However, the structure (abundance and composition) and dynamics of the microaerophilic FeOB community in paddy soil under field conditions have been unknown. The knowledge of the ecology of microaerophilic FeOB community in the fields must be helpful to consider their roles and contributions not only in the redox cycle of Fe but also the related biogeochemistry of paddy field soil.
In the past decade, some studies investigated the community structure of microaerophilic FeOB in the family Gallionellaceae in wetland soils by using polymerase chain reaction (PCR)-based molecular techniques (e.g., Wang et al. Citation2009, Citation2011, Citation2012). However, the primers targeting 16S rRNA genes of Gallionella-related FeOB in these studies have included some mismatched bases to the sequences of known FeOB and clones obtained from Fe oxides-deposited microbial colonies in our previous study (Watanabe et al. Citation2013). Therefore, the present study designed a new primer set for PCR-denaturing gradient gel electrophoresis (PCR-DGGE) and quantitative PCR (qPCR) analyses of microaerophilic FeOB community in the family Gallionellaceae and revealed the community structure and their relation to the redox fluctuation of Fe in two Japanese paddy field soils.
2. Materials and methods
2.1. Study fields
Paddy fields located in the Aichi Agricultural Research Center (Anjo; latitude 34°58ʹ20ʹ’N, longitude 137°04ʹ28ʹ’E) and NARO Tohoku Agricultural Research Center (Omagari; latitude 39°29ʹ28ʹ’N, longitude 140°29ʹ47ʹ’E) were investigated in 2015.
Two replicate plots (B4 and B5) of the Anjo field were managed as a double-cropping system of rice (Oryza sativa L. Aichinokaori SBL) and wheat (Triticum aestivum L. Iwainodaichi) in summer and winter, respectively. The area of the B4 and B5 plots was 2.2 × 103 and 1.6 × 103 m2, respectively. The Anjo soil was classified as the Anthraquic Yellow Soil (Oxyaquic Dystrudepts; Soil Survey Staff Citation1999) with Light Clay texture. Chemical properties of the soil were as follows: total carbon, 22.5 g kg−1; total nitrogen, 1.9 g kg−1; pH(H2O) of air-dried soil, 5.28; dithionite reducible Fe (Fed), 11.7 g kg−1; oxalate (pH 3.0) extractable Fe (Feo), 4.99 g kg−1. Field operations are shown in . Basal fertilization (N, 42 kg ha−1; P2O5, 39 kg ha−1; K2O, 12 kg ha−1) and the first (N, 16 kg ha−1; K2O, 8 kg ha−1) and second (N, 24 kg ha−1; K2O, 12 kg ha−1) top-dressings were performed on 17 June, 6 August and 17 August, respectively.
Table 1. Field operations and sampling date of the Anjo and Omagari paddy fields
Long-term fertilizer experimental plots of chemical fertilizer (NPK-1 and NPK-6) and rice straw compost (RSC-14 and RSC-19) were selected in the Omagari field. The experiment has been continued since 1968. Rice (O. sativa L. Kiyonishiki) was cultivated in summer, and the field was fallow after rice harvesting under drained conditions. The area of the each plot was 40 m2. Chemical fertilizer was applied to the NPK plots, while rice straw compost was applied to the RSC plots in addition to chemical fertilizer. The Omagari soil was classified as Gray Lowland Soil (Typic Fluvaquents; Soil Survey Staff Citation1999) with Silty Clay Loam texture. Chemical properties of the soils were as follows: total carbon, 18.0 g kg−1 (NPK) and 22.3 g kg−1 (RSC); total nitrogen, 1.6 g kg−1 (NPK) and 2.0 g kg−1 (RSC); pH(H2O) of air-dried soils, 4.92 (NPK) and 4.89 (RSC); Fed, 6.43 g kg−1 (NPK) and 6.79 g kg−1 (RSC); Feo, 4.90 g kg−1 (NPK) and 4.97 g kg−1 (RSC). Field operations are shown in . Basal fertilization (N, 60 kg ha−1; P2O5, 80 kg ha−1; K2O, 60 kg ha−1) and top-dressing (N, 20 kg ha−1; K2O, 20 kg ha−1) were performed on 15 May and 10 July, respectively. The RSC plots were amended with 10 t ha−1 of rice straw compost on 10 April.
2.2. Soil sampling
Soil samples were taken from the fields before flooding (Anjo, 8 June; Omagari, 7 April), at one month after flooding (Anjo, 17 July; Omagari, 25 June), at the end of midsummer drainage (Anjo, 30 July; Omagari, 2 July), at flowering stage (Anjo, 2 September; Omagari, 12 August) and after rice harvesting (Anjo, 27 October; Omagari, 9 October) in 2015 (). Each 100 cm3 of bulk soil was collected from 6 spots in the respective plots by using a soil core sampler (height, 5 cm; volume, 100 cm3). The sampling spots were randomly selected from the undisturbed zones in the center of four rice hills. The core samplers were closed with caps and immediately brought back to an on-site experimental room. The soil of each core was divided into 0–1 and 1–5 cm depths by a knife. Afterward a composite soil sample was prepared by mixing well for each depth from the 6 cores of each plot: in total, each 4 composite samples (2 depths × 2 replicate plots) were prepared for the Anjo and Omagari NPK and RSC fields. Only for the soil samples at the end of midsummer drainage, the soil samples were divided into 0–1, 1–2 and 2–5 cm depths under the assumption of extended gradient redox conditions in the soils during the drainage. A part of the composite soil samples was immediately subjected to the extraction of Fe(II), and some portions of the other part were frozen at −20°C for DNA extraction. The moisture content of the soil samples was determined by oven drying at 105°C for more than 16 h with two replications.
2.3. Measurement of ferrous iron
As an indicator of Fe redox status of the soil, Fe(II) was extracted from a composite soil sample with 1M acetate buffer (pH 3.0) with triplicate replications, in accordance with the method described by Takai, Kamura, and Adachi (Citation1958). The suspension was filtered with a filter paper (No. 6, Advantec, Tokyo, Japan). The concentrations of Fe(II) ion in the filtrates were measured by using the colorimetric analysis with o-phenantroline.
2.4. DNA extraction
Total DNA was extracted from 0.5 g of a composite soil sample with triplicate replications by using the ISOIL for beads beating kit (Nippon Gene, Tokyo, Japan), in accordance with the kit procedure. A FastPrep 24 Instrument (MP Biomedicals, Solon, OH, USA) was used to disrupt microbial cells in the soil samples under the condition at 6 m/s for 40 s. Extracted DNA was dissolved in 100 μl of TE buffer (10 mM Tris-HCl, 1.0 mM ethylenediaminetetraacetic acid [EDTA]; pH 8.0) and checked by using agarose electrophoresis with ethidium bromide.
2.5. Primer design
The primers M122F and Beta3R were used for the PCR amplification of partial 16S rRNA gene sequences (ca. 541 bp without the primer sequences) of microaerophilic FeOB in the family Gallionellaceae. The sequence of forward primer M122F (5ʹ-TAT CGG AAC RTR TCC GGA-3ʹ; Escherichia coli position, 123–141) was modified from the primer 122f (Wang et al. Citation2009). To design M122F, 16S rRNA gene sequences of the reference strains (44 sequences) and environmental clones (54 sequences) in the Betaproteobacteria were aligned on the ClustalW in the software MEGA5.2.2. The reference strains and environmental clones used for the alignment were shown in our previous study (Watanabe et al. Citation2013). The sequences of the target region of the primer 122f were manually confirmed between the Gallionella-related group and the other sequences, and the primer M122F was newly designed. The Gallionella-related group consisted of known FeOB, ‘S. lthotrophicus’ ES-1 (accession no., DQ386264), ‘S. lthotrophicus’ LD-1 (DQ386859), ‘S. paludicola’ BrT (DQ386858), G. ferruginea Johan (L07897) and G. capsiferriformans ES-2 (DQ386858), some clones obtained from the Fe-deposited microbial colonies (AB748619 – AB748622, AB748624, AB748625, AB748630, AB748638, AB748641 – AB748645, AB748647, AB748648, AB748654, AB748657 and AB748659; Watanabe et al. Citation2013) and some environmental clones (GQ388919, EF520478, AB240323 and FN430669). A GC clamp (40 bp; Muyzer, de Waal, and Uitterlinden Citation1993) was added to the forward primer (M122F-GC) for the PCR-DGGE. The reverse primer Beta3R (5ʹ-ACG CAT TTC ACT GCT ACA CG-3ʹ; E. coli position, 682–701) was the same as the probe Beta3, which was designed for Betaproteobacteria in silico by the PRIMROSE (Ashelford et al., Citation2002).
2.6. Polymerase chain reaction-denaturing gradient gel electrophoresis analysis
Each 50 μl of PCR reaction mixture contained 5 μl of 10 × ExTaq buffer (TaKaRa, Kusatsu, Japan), 4 μl of dNTP (2.5 mM each, TaKaRa), each 0.5 μl of M122F-GC and Beta3R (each 50 μM), 0.25 μl of ExTaq polymerase (5 U μl−1, TaKaRa), 0.5 μl of bovine serum albumin (20 mg ml−1, Roche, Basel, Switzerland) and 1 μl of extracted DNA. The program of PCR consisted of initial denaturation at 95°C for 90 s, 35 cycles of denaturation at 95°C for 30 s, annealing at 55°C for 30 s and elongation at 72°C for 90 s, followed by final elongation at 72°C for 6 min. Amplified products were confirmed by using agarose electrophoresis with ethidium bromide.
PCR products amplified with the primers M122F-GC and Beta3R were subjected to DGGE. DGGE was performed with Dcode Universal Mutation Detection System (Bio-Rad Laboratories, Hercules, CA, USA) under the run condition in 1 × TAE buffer (40 mM Tris-acetate, 1.0 mM EDTA) at 60°C and 100 V for 14 h. Denaturing gradient ranged from 32 to 62% was prepared in 8% (w/v) polyacrylamide gel including 1 × TAE buffer, in which 100% denaturant included 7 M urea and 40% (v/v) formamide. 8 μl of PCR amplicons were applied onto 32 wells of the gel. The gel after electrophoresis was stained with SYBR Green I nucleic acid gel stain (Lonza, Rockland, ME, USA) and photographed on the Safe Imager (Invitrogen, Carlsbad, CA, USA) with the AMZ Limited-STAGE (AMZ System Science, Osaka, Japan).
2.7. Phylogenetic analysis
The observed DGGE bands were subjected to sequencing analysis. DGGE bands were excised from the gel and the gene fragments were reamplified with M122F-GC and Beta3R. Band purity and mobility of the reamplified products were confirmed by comparing with the original band patterns on a same DGGE gel. The purification step was repeated two times. The gene fragments in purified bands were amplified with M122F (without GC clamp) and Beta3R, and the amplicons were purified with the NucleoSpin Gel and PCR Clean-up kit (Macherey-Nagel, Düren, Germany), followed by outsourcing to the Eurofins DNA sequencing service (Eurofins Genomics, Tokyo, Japan) for the cycle sequencing reaction and sequencing analysis. Closest relatives of the band sequences obtained were searched on the BLAST program. To show the phylogenetic relationship of the representative sequences on a tree, the obtained sequences were aligned on the SINA (SILVA Incremental Aligner) program (Pruesse, Peplies, and Glöckner Citation2012) and the evolutionary distances among the sequences were calculated on the software package ARB (Ludwig et al. Citation2004) with the Jukes-Cantor model. And then the sequences were clustered into operational taxonomic units (OTUs) on the basis of the distance matrix with 97% similarity on the software mothur (Schloss et al. Citation2009). A neighbor-joining tree of reference bacteria (ca. 1400 bp), which were also aligned on the SINA, was constructed first on the ARB with the Jukes-Cantor model and 1,000 resampling bootstrap analysis. After that, the each one sequence from the OTUs was positioned onto the tree by using the parsimony method.
2.8. Quantitative PCR
The copy number of 16S rRNA genes of Gallionella-related FeOB was quantified by using real-time PCR (Thermal Cycler Dice Real Time System II, TaKaRa). The primers were the same as the primers used for PCR-DGGE analysis, but GC clump was not attached to the M122F. Each 25 μl of reaction mixture contained 12.5 μl of SYBR Premix ExTaq, each 0.1 μl of M122F (without GC clamp) and Beta3R (each 50 μM), 2 μl of 20-fold diluted DNA. The program for the real-time PCR consisted of initial denaturation at 95°C for 10 s, 45 cycles of denaturation at 95°C for 40 s, annealing at 55°C for 30 s and elongation at 72°C for 1 min, followed by melting curve analysis at 95°C for 15 s, 65°C for 30 s and 95°C for 15 s. Fluorescence signal was detected at the end of each elongation step. Serial dilutions (101–107 copies μl−1) of 16S rRNA gene fragments (1,501 bp including the primer sequences) of F. kumadai An22 (Khalifa et al. Citation2018) were used as the reference standard, which were amplified from the genomic DNA with the primers 27f and 1492r (Lane Citation1991). Threshold cycles (Ct values) were calculated by using the second derivative maximum method. PCR efficiency was more than 80%, which were calculated from the formula; e = 10−1/slope − 1, where slope indicates the slope of calibration curve. To take account of PCR inhibitors in DNA solution, e.g., humic substances, the recovery ratios were confirmed by adding the standard references to the template DNA (Watanabe, Kimura, and Asakawa Citation2007). The recovery was almost 100%.
2.9. Statistical analysis
The copy numbers of the 16S rRNA genes were logarithmically transformed. The differences of the moisture contents, the contents of acetate-extractable Fe(II) and the copy numbers of 16S rRNA genes were examined with the Mann-Whitney U test or Wilcoxon signed-rank test, using the Ekuseru-Toukei 2012 (Social Survey Research Information, Tokyo, Japan). Pearson correlation coefficients or Spearman’s rank correlation coefficients were obtained between the moisture and acetate-extractable Fe(II) contents, and the copy numbers of the 16S rRNA genes and acetate-extractable Fe(II) contents after confirming the normality of the data. Principal component analysis (PCA) of DGGE band patterns was performed with the function ‘prcomp’ on the statistical soft R to characterize the community structures. DGGE bands were classified into four categories (0–3) depending on the relative intensities by visual evaluation. The variance-covariance matrix was subjected to the PCA.
2.10. Accession numbers
The sequences determined in the present study were deposited in the DDBJ/EMBL/Genbank database under accession numbers from LC455878 to LC455931.
3. Results
3.1. Changes in the acetate-extractable iron (II) contents in the paddy field soils
Drastic changes in the redox status of Fe were observed in the both fields in the annual cycle of the field operations. The acetate-extractable Fe(II) content in the soils was low before flooding in the both fields. And then the content largely increased and decreased under flooded (17 July and 2 September in Anjo and 25 June and 12 August in Omagari) and drained (27 October in Anjo and 9 October in Omagari) conditions, respectively (): Mann-Whitney U test between rice cultivation period vs. non-rice cultivation period; Anjo, p < 0.05; Omagari NPK and RSC, p < 0.001). However, the impact of midsummer drainage was different between the Anjo and Omagari fields. In the Anjo field, the Fe(II) content drastically decreased after 9 days of midsummer drainage (30 July), while the Fe(II) content in both the Omagari NPK and RSC plots was almost kept after one week of midsummer drainage (2 July). In the both Anjo and Omagari fields, no significant difference was observed in the Fe(II) contents between the soil depths (0–1 cm vs. below 1 cm) although the Fe(II) contents in the surface soil tended to be lower during the rice cultivation periods.
Figure 1. Acetate-extractable Fe(II) (a) and moisture contents (b) of the soils during a year of rice cropping season. Bars indicate the difference in the values of Fe(II) and moisture contents between two replicate plots, in which each plot has three (Fe) and two (moisture) replications. Asterisks (* and **) indicate significant differences between the rice cultivation period vs. the non-rice cultivation period and 0 − 1 cm depth vs. below 1cm depth (p < 0.05 and p < 0.01, respectively). Gray zones in the dates indicate the rice cultivation period
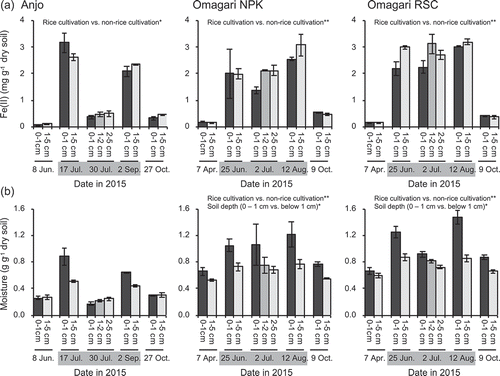
The changes in the moisture conditions were similar to those in the acetate-extractable Fe(II) contents ()). Significant positive correlations were observed between the moisture and Fe(II) contents in both the Anjo and Omagari fields (Anjo, r = 0.72 [p < 0.01]; Omagari NPK, r = 0.47 [p < 0.05]; Omagari RSC, r = 0.50 [p < 0.05]). The moisture contents were higher in the Omagari field than the Anjo field (Wilcoxon signed-rank test, p < 0.001 for Anjo vs. Omagari NPK or RSC).
3.2. Changes in the community structure of Gallionella-related iron-oxidizing bacteria (FeOB) revealed by DGGE analysis
Designed new primer set, M122F-GC and Beta3R, was successfully applied to the community analysis of microaerophilic FeOB in the family Gallionellaceae. In the preliminary experiments, different pairs of some candidate primers, M122F (present study)/517r (Muyzer, de Waal, and Uitterlinden Citation1993), and Gal221 (Fleming et al. Citation2014)/517r, which produced shorter PCR-amplicons (412 and 314 bp, respectively), were also examined in addition to the pair of M122F/Beta3R (data not shown). However, nonspecific PCR products were obtained, or the phylogenetic positions of the band and reference sequences were not always consistent when the primer sets other than M122F/Beta3R were used (data not shown). Therefore, the primer set M122F/Beta3R was selected to analyze Gallionella-related FeOB community in paddy field soil, as described below.
Approximately 620 bp of PCR amplicons including the primer regions and GC clump were obtained from all the samples with the primer set M122F-GC/Beta3R. When these amplicons were applied to the DGGE, 22–32 and 25–36 bands were observed from the Anjo and Omagari samples, respectively, in which 15 bands were commonly observed from the both fields ()). However, the band patterns were largely different between the fields, as also revealed by the PCA of the patterns ()).
Figure 2. DGGE band patterns of Gallionella-related Fe-oxidizing bacterial 16S rRNA genes obtained from soil samples of three paddy fields (a) and principal component analysis of the patterns (b and c). The denaturant gradient range is 32–62%. The nucleotide sequences of the bands with closed symbols were determined. The numbers with the symbols corresponds to the OTU numbers in . Open symbols indicate the bands that sequences were not obtained. The numbers below the each lane shows the number of the bands observed from the each sample. PC, principal component. Percentages in parentheses show the contribution of each principal component. Diamond, triangle and square symbols indicate the samples obtained from the Anjo and Omagari NPK and RSC fields, respectively. Biplots of PC1 vs. PC2 (b) and PC1 vs. PC3 (c) are shown to explain the differences between the Anjo and Omagari fields and between the NPK and RSC plots in the Omagari field
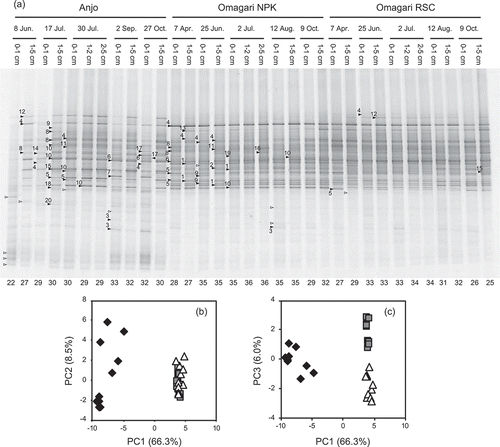
In the Anjo field, the patterns before the midsummer drainage (8 Jun. and 17 Jul.) were different between the soil depths: the intensity of the bands obtained from the surface soil layer was weak. However, the band intensity in the patterns from the midsummer drainage (30 Jul.) increased even at the surface soil layer, and the patterns were similar among the soil depths. Afterward, the patterns showed some differences again between the soil depths (2 Sep. and 27 Oct.). On the other hand, changes in the patterns were few during the annual field operations in the Omagari field. When the patterns were compared between the NPK and RSC plots in the Omagari field, where the fertilization managements were different between them, most of the bands were commonly observed, while minor differences were also observed ()). The similar differences (Anjo vs. Omagari and Omagari NPK vs. RSC) were observed when only the bands derived from Gallionella-related FeOB were subjected to the PCA (data not shown and see below).
3.3. Phylogenetic diversity of Gallionella-related FeOB in the paddy field soils
Nucleotide sequences (541–543 bp) of each 27 bands were successfully obtained from the Anjo and Omagari fields, respectively, of which 50 of 54 bands were affiliated within the Fe(II)-oxidizing group in the family Gallionellaceae (). The 54 sequences were clustered into 20 OTUs on the basis of the 97% similarity. Some of them were clustered with known microaerophilic FeOB, i.e., F. kumadai (OTU11), Ferriphaselus amnicola (OTU18 and 20), ‘S. lithotrophicus’ (OTU6, 10, 15, 16 and 17) and ‘S. paludicola’ (OTU1 and 2). Meanwhile, the others were independently clustered (e.g., OTU9 and 14 and OTU4, 5, 7 and 13). OTU18 and 20, which were related to F. amnicola, were obtained only from the Anjo field, while OTU1 and 2, which were clustered with ‘S. paludicola’, were obtained only from the Omagari fields. OTU19 was clustered with ‘Candidatus Nitrotoga arctica’, known as a nitrite oxidizer. OTU3 was not related to Gallionella-related FeOB, but an acidophilic FeOB, ‘Ferrovum myxofaciens’, although the similarity was not greater than 90%.
Figure 3. Phylogenetic relationships of 16S rRNA gene sequences retrieved from DGGE bands. Nucleotide sequences (541–543 bp) obtained in the present study were clustered into operational taxonomic units on the basis of 97% similarity. One sequence from each OTU was added in a neighbor-joining tree of the reference sequences (ca. 1400bp) by using the parsimony method of the software package ARB. The numbers in parentheses of each OTU indicate the number of sequences obtained from the Anjo and Omagari fields. Bootstrap values are shown as closed (>75%) and open circles (50–75%). Accession numbers of the references are inserted in parentheses. Nitrosomonas europaea ATCC 25978 is used as the outgroup. Bar, 0.1 substitutions per nucleotide sequence position
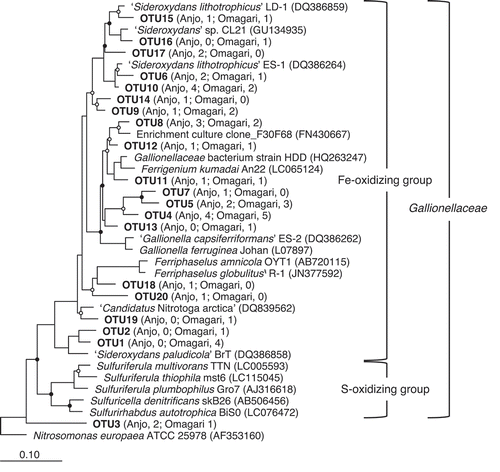
3.4. Abundance of Gallionella-related FeOB in paddy field soil
The copy number of FeOB 16S rRNA genes was approximately 105–107 and 106–108 copies g−1 dry soil in the Anjo and Omagari fields, respectively (). The copy numbers of 16S rRNA genes tended to peak at the midsummer drainage (30 July) in the Anjo field. On the other hand, the copy numbers of 16S rRNA genes were relatively stable in the Omagari field although the numbers tended to decrease after rice harvesting (9 October). Significant differences in the copy numbers of 16S rRNA genes were observed between the rice cultivation period (from 17 July to 2 September in Anjo and from 25 June to 12 August in Omagari) and non-rice cultivation period (8 Jun and 27 October in Anjo and 7 April and 9 October in Omagari) only in the Anjo field (Mann-Whitney U test, p < 0.05) and Omagari RSC plot (Mann-Whitney U test, p < 0.01). Significant differences in the copy numbers of 16S rRNA genes between the soil depths were observed only in the Anjo and Omagari RSC fields (Mann-Whitney U test, p < 0.05).
Figure 4. Copy numbers of 16S rRNA genes of Gallionella-related FeOB in the soils during a year of rice cropping season. Bars indicate the difference in the values of the copy numbers between two replicate plots, in which three DNA replicates were prepared in each plot. Asterisks (* and **) indicate significant differences between the rice cultivation period vs. the non-rice cultivation period and 0 − 1 cm depth vs. below 1cm depth (p < 0.05 and p < 0.01, respectively). Gray zones in the dates indicate the rice cultivation period
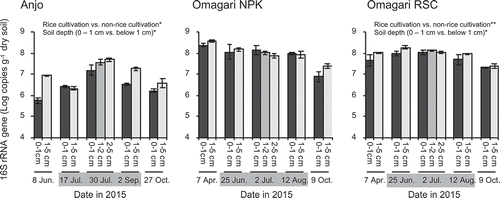
Correlations between the copy numbers of 16S rRNA genes () and acetate-extractable Fe(II) contents () were shown in . Significant negative correlations were observed during the rice cultivation period in both the Anjo and Omagari fields although the correlation was not significant when focusing on only the Omagari RSC plot. In the Omagari field, significant negative correlations between the copy numbers of 16S rRNA genes and acetate-extractable Fe(II) contents were observed even during the non-rice cultivation period.
Figure 5. Correlations between the contents of acetate-extractable Fe(II) and the copy numbers of Gallionella-related Fe-oxidizing bacterial 16S rRNA genes. Diamonds and triangles are the values obtained from rice cultivation (from 17 July to 2 September and from 25 June to 12 August in the Anjo and Omagari fields, respectively) and non-rice cultivation periods (8 Jun and 27 October and 7 April and 9 October in the Anjo and Omagari fields, respectively), respectively. Asterisks (* and **) indicate that the values are significantly correlated (p < 0.05 and p < 0.01, respectively)
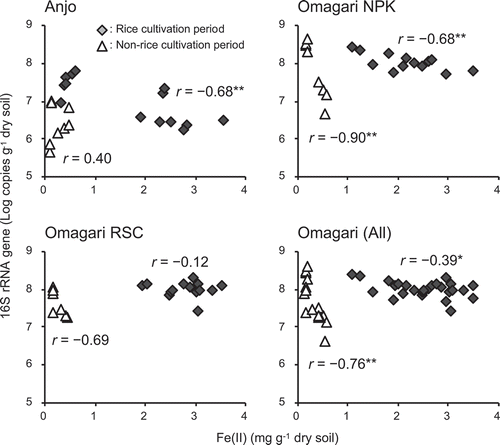
4. Discussion
4.1. Specificity of the designed primers for Gallionella-related FeOB community in paddy field soil
The present study designed a new primer set to detect microaerophilic FeOB in the family Gallionellaceae in paddy field soil. By using the new primer set, 22–36 DGGE bands were detected and 20 OTUs were obtained by using the DGGE method. Eighteen out of twenty OTUs belong to the FeOB groups in the family Gallionellaceae. The family Gallionellaceae is now composed of FeOB and sulfur-oxidizing bacterial groups (Boden, Hutt, and Rae Citation2017), of which bacterial 16S rRNA gene sequences related to Ferrigenium, Ferriphaselus, ‘S. lithotrophicus’ and ‘S. paludicola’ were obtained from the paddy field soils. Since common characteristics of the known FeOB in the Gallionellaceae are the ability of Fe(II) oxidation under microoxic conditions, the Gallionellaceae detected in the present study as the 18 OTUs were expected to have an ability to oxidize Fe(II) under microoxic conditions, that is microaerophilic FeOB. The exception is that the sequences of a few numbers (4 of 50 bands) were related to bacteria other than Gallionella-related FeOB, ‘Candidatus Nitrotoga arctica’ (Alawi et al. Citation2007) and ‘Ferrovum myxofaciens’ (Johnson, Hallberg, and Hedrich Citation2014). Therefore, some attentions for amplifying bacterial 16S rRNA genes other than Gallionella-related FeOB are needed. Although all of the known Gallionella-related FeOB grow under circumneutral pH conditions, the growth and physiological characteristics such as growth temperature and pH ranges, substrate availability other than Fe(II) and ability of twisted stalk formation, which is unique structure of Fe oxides, are dependent on the strains. Therefore, physiological characteristics of the respective putative microaerophilic FeOB in the paddy field soils cannot be speculated from the current knowledge. More knowledge about the relations between the phylogeny and physiology of the microaerophilic FeOB is needed.
Quantification of the copy number of Gallionella-related FeOB 16S rRNA genes was also succeeded and 105–108 copies g−1 dry soil were detected from the paddy field soils. Although the experimental conditions (e.g., primers and PCR conditions) were different from the conditions applied in the present study, the copy numbers of Gallionella-related FeOB 16S rRNA genes in Fe-rich, metal-contaminated creek sediments, which is a typical habitat of Gallionella-related FeOB, were reported to be ca. 109 copies g−1 wet sediment (Fabisch et al. Citation2013). The copy numbers in some freshwater environments were 105–107 copies g−1 dry soil (an irregularly flooded, riparian wetland; Wang et al. Citation2012) and <105 copies g−1 dry weight (a tidal freshwater marsh; Wang et al. Citation2011). Given the orders of magnitude in these previous studies, the present study suggests that considerable number of Gallionella-related FeOB inhabits paddy field soil as well as other freshwater environments where Fe(II) is exposed to oxic conditions.
4.2. Dynamics of the Gallionella-related FeOB community in paddy field soils
We expected that community structure of Gallionella-related FeOB would change depending on the soil conditions, especially redox status of Fe, and be different between the surface and deeper soils (0–1 cm vs. below 1 cm) under flooded conditions. Community analysis with the DGGE and qPCR techniques partly supported the hypothesis and showed a relationship between the dynamics of Gallionella-related FeOB and redox status of Fe during the rice cultivation period.
4.2.1. Relation to the changes in the Fe(II) contents in paddy field soils in annual cycle of field management
qPCR analysis showed that some relationships between the dynamics of Gallionella-related FeOB and redox status of Fe in the annual cycle of the field operations. The abundances of Gallionella-related FeOB were negatively correlated to the contents of acetate-extractable Fe(II) during the rice cultivation period (), suggesting that Gallionella-related FeOB would have been activated (i.e., oxidizing Fe(II)) when the soil conditions changed from reduced to oxic conditions and contributed to the Fe(II) oxidation. However, no correlation was observed between the abundances of Gallionella-related FeOB and the contents of acetate-extractable Fe(II) in both the fields when comparing all the values from both the rice cultivation and non-rice cultivation periods, suggesting that redox status in the soils and the responses of the Gallionella-related FeOB were largely different between the rice cultivation and non-rice cultivation periods.
Since optimal ranges of O2 concentration for the growth of microaerophilic FeOB were quite limited (<50 μM) to outcompete abiotic Fe(II) oxidation (Druschel et al. Citation2008; Lueder et al. Citation2018), Gallionella-related FeOB would have been activated with traces of O2 not only at the surface soil but also the rhizosphere soil during the rice cultivation period, where substrate for Fe(II) oxidation (i.e., Fe(II)) is abundant, while O2 availability is limited. Meanwhile, the apparent redox cycle of Fe was probably almost inactive in the soils at macro scale during the non-rice cultivation period under drained conditions because both moisture and Fe(II) contents decreased and large portions of the plowed soil layer must change to oxic conditions. Differences in these physicochemical conditions between the rice cultivation and non-rice cultivation periods likely influence the dynamics of Gallionella-related FeOB community in the soil.
In the Omagari field, however, a significant negative correlation was observed between the abundances of Gallionella-related FeOB and the contents of acetate-extractable Fe(II) even during the non-rice cultivation period under drained conditions and the slope of the regression line was steep, compared with that during the rice cultivation period (p < 0.01). Because the field is covered by snow from November or December to late March or early April, melting of the snow and increasing temperature in the early spring might influence the redox cycle of Fe and the activity of FeOB at microsites in the soils even under drained and low Fe(II) contents conditions. These results suggest that not only apparent Fe(II) contents but also other factors (e.g., soil water conditions, O2 availability and temperature change) must affect the net redox cycle of Fe and dynamics of microaerophilic FeOB in paddy field soil. Further studies for long-term monitoring for years and intensive monitoring in a short period will help to clarify the contribution of microaerophilic FeOB to the Fe oxidation process in paddy field soil.
4.2.2. Community dynamics at the surface soil under flooded conditions
Dynamics of the community structure of Gallionella-related FeOB at the surface soil under flooded conditions was varied. In the Anjo field, the DGGE band patterns were slightly different between the soil depths except for the patterns in the midsummer drainage. In contrast, the band patterns in the Omagari field were not different between the soil depths. The abundance of Gallionella-related FeOB was not different between the soil depths in both the fields when only focusing on the rice cultivation period. In a flooded paddy soil without rice plants, O2 was depleted within the surface 3–5 mm, while O2 was detectable down to at least 40 mm when rice plants were planted (Frenzel, Rothfuss, and Conrad Citation1992). Since the O2 profiles in flooded soil is changeable, the depth of 1 cm used for the analysis might be not appropriate on the scale to observe the differences in the DGGE band patterns in the Omagari field.
Furthermore, anaerobic nitrate-dependent Fe oxidation by the consortium of ‘Sideroxydans’ sp. (= clone F30F68 in ) and other heterotorphic denitrifying bacteria has been reported (He et al. Citation2016). A similar microbial consortium possibly developed in the paddy field soils under anoxic conditions, resulting that the composition of Gallionella-related FeOB in the anoxic soil may be different from that in the surface oxic soil as seen in the Anjo, while the population may be maintained even below the soil surface. Further studies that focus on the dynamics of Gallionella-related FeOB as well as the physiological versatility and their relation of the development of surface oxic soil layer are needed.
5. Conclusions
The present study showed inhabitance of diverse microaerophilic FeOB belonging to the family Gallionellaceae in paddy field soils for the first time. DGGE analysis showed that the community structure was distinguished between the field sites. In the Anjo field, the community structure of the surface soil layer was different from that of the lower soil layer. On the other hand, the community structure of the Omagari field was relatively stable in the annual cycle of the field operations. Not only apparent redox status of Fe in the soil but also other factors such as water and temperature conditions and rice rhizosphere possibly affected the community structure. Nonetheless, significant negative correlations between Fe(II) contents and Gallionella-related FeOB abundances were observed during the rice cultivation period in both the fields, suggesting the involvement of Gallionella-related FeOB in the Fe(II)-oxidizing process in the soils although the contribution remains to be evaluated.
Fe oxidation in paddy fields must be intricately regulated by not only microaerophilic FeOB but also abiotic oxidation, nitrate-dependent anaerobic FeOB and possibly anaerobic phototrophic FeOB. The present study opened up the new ideas and further studies on the ecophysiological characteristics of microaerophilic FeOB, factors affecting the community structure and relations to the soil and climatic conditions will be needed to understand the roles of microaerophilic FeOB in the Fe cycle of paddy field soil.
Disclosure statement
No potential conflict of interest was reported by the authors.
Additional information
Funding
References
- Alawi, M., A. Lipski, T. Sanders, E. M. Pfeiffer, and E. Spieck. 2007. “Cultivation of a Novel Cold-adapted Nitrite Oxidizing Betaproteobacterium from the Siberian Arctic.” The ISME Journal 1: 256–264. doi:10.1038/ismej.2007.34.
- Ashelford, K. E., A. J. Weightman, and J. C. Fry. 2002. “PRIMROSE: A Computer Program for Generating and Estimating the Phylogenetic Range of 16S rRNA Oligonucleotide Probes and Primers in Conjunction with the RDP-II Database.” Nucleic Acids Research 30: 3481–3489. doi:10.1093/nar/gkf450.
- Becker, M., and F. Asch. 2005. “Iron Toxicity in Rice–Conditions and Management Concepts.” Journal of Plant Nutrition and Soil Science 168: 558–573. doi:10.1002/jpln.200520504.
- Boden, R., L. P. Hutt, and A. W. Rae. 2017. “Reclassification of Thiobacillus Aquaesulis (wood & Kelly, 1995) as Annwoodia Aquaesulis Gen. Nov., Comb. Nov., Transfer of Thiobacillus (beijerinck, 1904) from the Hydrogenophilales to the Nitrosomonadales, Proposal of Hydrogenophilalia Class. Nov. Within the ‘proteobacteria’, and Four New Families within the Orders Nitrosomonadales and Rhodocyclales.” International Journal of Systematic and Evolutionary Microbiology 67: 1191–1205. doi:10.1099/ijsem.0.001927.
- Chen, X. P., W. D. Kong, J. Z. He, W. J. Liu, S. E. Smith, F. A. Smith, and Y. G. Zhu. 2008. “Do Water Regimes Affect Iron-plaque Formation and Microbial Communities in the Rhizosphere of Paddy Rice?” Journal of Plant Nutrition and Soil Science 171: 193–199. doi:10.1002/jpln.200700018.
- Conrad, R. 2002. “Control of Microbial Methane Production in Wetland Rice Fields.” Nutrient Cycling in Agroecosystems 64: 59–69. doi:10.1023/A:1021178713988.
- Druschel, G. K., D. Emerson, R. Sutka, P. Suchecki, and G. W. Luther III. 2008. “Low-oxygen and Chemical Kinetic Constraints on the Geochemical Niche of Neutrophilic iron(II) Oxidizing Microorganisms.” Geochimica et Cosmochimica Acta 72: 3358–3370. doi:10.1016/j.gca.2008.04.035.
- Ehrenberg, C. G. 1836. “Vorläufige Mittheilungen Über Das Wirkliche Vorkommen Fossiler Infusorien Und Ihre Grosse Verbreitung.” Annals of Physics 114: 213–227. (in German). doi:10.1002/andp.18361140520.
- Emerson, D., E. J. Fleming, and J. M. McBeth. 2010. “Iron-oxidizing Bacteria: An Environmental and Genomic Perspective.” Annual Review of Microbiology 64: 561–583. doi:10.1146/annurev.micro.112408.134208.
- Fabisch, M., F. Beulig, D. M. Akob, and K. Küsel. 2013. “Surprising Abundance of Gallionella-related Iron Oxidizers in Creek Sediments at pH 4.4 Or at High Heavy Metal Concentrations.” Frontiers in Microbiology 4: 390. doi:10.3389/fmicb.2013.00390.
- Fleming, E. J., I. Cetinić, C. S. Chan, D. W. King, and D. Emerson. 2014. “Ecological Succession among Iron-oxidizing Bacteria.” The ISME Journal 8: 804–8415. doi:10.1038/ismej.2013.197.
- Frenzel, P., F. Rothfuss, and R. Conrad. 1992. “Oxygen Profiles and Methane Turnover in a Flooded Rice Microcosm.” Biology and Fertility of Soils 14: 84–89. doi:10.1007/BF00336255.
- He, S., C. Tominski, A. Kappler, S. Behrens, and E. E. Roden. 2016. “Metagenomic Analyses of the Autotrophic Fe(II)-oxidizing, Nitrate-reducing Enrichment Culture KS.” Applied and Environmental Microbiology 82: 2656–2668. doi:10.1128/AEM.03493-15.
- Hedrich, S., M. Schlömann, and D. B. Johnson. 2011. “The Iron-oxidizing Proteobacteria.” Microbiology 157: 1551–1564. doi:10.1099/mic.0.045344-0.
- Johnson, D. B., K. B. Hallberg, and S. Hedrich. 2014. “Uncovering a Microbial Enigma: Isolation and Characterization of the Streamer-generating, Iron-oxidizing, Achidophilic Bacterium “ferrovum Myxofaciens”.” Applied and Environmental Microbiology 80: 672–680. doi:10.1128/AEM.03230-13.
- Kappler, A., and C. Bryce. 2017. “Cryptic Biogeochemical Cycles: Unravelling Hidden Redox Reactions.” Environmental Microbiology 19: 842–846. doi:10.1111/1462-2920.13687.
- Kato, S., S. Krepski, S. Chan, T. Itoh, and M. Ohkuma. 2014. “Ferriphaselus Amnicola Gen. Nov., Sp. Nov., A Neutrophilic, Stalk-forming, Iron-oxidizing Bacterium Isolated from an Iron-rich Groundwater Seep.” International Journal of Systematic and Evolutionary Microbiology 64: 921–925. doi:10.1099/ijs.0.058487-0.
- Khalifa, A., Y. Nakasuji, N. Saka, H. Honjo, S. Asakawa, and T. Watanabe. 2018. “Ferrigenium Kumadai Gen. Nov., Sp. Nov., A Microaerophilic Iron-oxidizing Bacterium Isolated from a Paddy Field Soil.” International Journal of Systematic and Evolutionary Microbiology 68: 2587–2592. doi:10.1099/ijsem.0.002992.
- Kögel-Knabner, I., W. Amelung, Z. Cao, S. Fiedler, P. Frenzel, R. Jahn, K. Kalbitz, A. Kölbl, and M. Schloter. 2010. “Beogeochemistry of Paddy Soils.” Geoderma 157: 1–14. doi:10.1016/j.geoderma.2010.03.009.
- Kützing, F. T. 1843. PhycologiaGeneralis: order, Anatomie, Physiologie und Systemkunde der Tange. (in German). Leipzig, Germany: F. A. Brockhaus.
- Kyuma, K. 2004. Paddy Soil Science. Kyoto, Japan: Kyoto University Press.
- Lane, D. J. 1991. “16S/23S rRNA Sequencing.” In Nucleic Acid Techniques in Bacterial Systematics, edited by E. Stackebrandt and M. Goodfellow, 115–175. Hoboken, NJ, USA: Wiley.
- Li, X., W. Zhang, T. Liu, L. Chen, P. Chen, and F. Li. 2016. “Changes in the Composition and Diversity of Microbial Communities during Anaerobic Nitrate Reduction and Fe(II) Oxidation at Circumneutral pH in Paddy Soil.” Soil Biology & Biochemistry 94: 70–79. doi:10.1016/j.soilbio.2015.11.013.
- Liesack, W., S. Schnell, and N. P. Revsbech. 2000. “Microbiology of Flooded Rice Paddies.” FEMS Microbiology Reviews 24: 625–645. doi:10.1111/j.1574-6976.2000.tb00563.x.
- Ludwig, W., O. Strunk, R. Westram, L. Richter, H. Meier, B. A. Yadhukumar, T. Lai, et al. 2004. “ARB: A Software Environment for Sequence Data.” Nucleic Acids Research 32: 1363–1371. doi:10.1093/nar/gkh293.
- Lueder, U., G. Druschel, D. Emerson, A. Kappler, and C. Schmidt. 2018. “Quantitative Analysis of O2 and Fe2+ Profiles in Gradient Tubes for Cultivation of Microaerophilic iron(II)-oxidizing Bacteria.” FEMS Microbiology Ecology 94. fix177. doi:10.1093/femsec/fix177.
- Meharg, A. A. 2004. “Arsenic in Rice – Understanding a New Disaster for South-East Asia.” Trends in Plant Science 9: 415–417. doi:10.1016/j.tplants.2004.07.002.
- Melton, E. D., E. D. Swanner, S. Behrens, C. Schmidt, and A. Kappler. 2014. “The Interplay of Microbially Mediated and Abiotic Reactions in the Biogeochemical Fe Cycle.” Nature Reviews. Microbiology 12: 797–808. doi:10.1038/nrmicro3347.
- Muyzer, G., E. C. de Waal, and A. G. Uitterlinden. 1993. “Profiling of Complex Microbial Populations by Denaturing Gradient Gel Electrophoresis Analysis of Polymerase Chain Reaction-amplified Genes Coding for 16S rRNA.” Applied and Environmental Microbiology 59: 695–700.
- Pruesse, E., J. Peplies, and F. O. Glöckner. 2012. “SINA: Accurate High-throughput Multiple Sequence Alignment of Ribosomal RNA Genes.” Bioinformatics 28: 1823–1829. doi:10.1093/bioinformatics/bts252.
- Ratering, S., and S. Schnell. 2001. “Nitrate-dependent iron(II) Oxidation in Paddy Soil.” Environmental Microbiology 3: 100–109. doi:10.1046/j.1462-2920.2001.00163.x.
- Roth, A. W. 1797. Catalecta Botanica Quibus Plantae Novae Et Minus Cognitae Describuntur Atque Illistrantur. (in Latin). Leipzig, Germany: I.G. Mülleriano.
- Schloss, P. D., S. L. Westcott, T. Ryabin, J. R. Hall, M. Hartmann, E. B. Hollister, R. A. Lesniewski, et al. 2009. “Introducing Mothur: Open-source, Platform-independent Community-supported Software for Describing and Comparing Microbial Communities.” Applied and Environmental Microbiology 75: 7537–7541. doi:10.1128/AEM.01541-09.
- Soil Survey Staff. 1999. Soil Taxonomy, A Basic System of Soil Classification for Making and Interpreting Soil Surveys. 2nd ed. Washington DC, USA: United States Department of Agriculture Natural Resources Conservation Service.
- Syu, C. H., P. Y. Jiang, H. H. Huang, W. T. Chen, T. H. Lin, and D. Y. Lee. 2013. “Arsenic Sequestration in Iron Plaque and Its Effect on as Uptake by Rice Plants Grown in Paddy Soils with High Contents of As, Iron Oxides, and Organic Matter.” Soil Science and Plant Nutrition 59: 463–471. doi:10.1080/00380768.2013.784950.
- Takai, Y., and T. Kamura. 1966. “The Mechanisms of Reduction in Waterlogged Paddy Soil.” Folia Microbiologica 11: 304–313. doi:10.1007/BF02878902.
- Takai, Y., T. Kamura, and I. Adachi. 1958. “Dynamic Behavior of Iron Compounds in Paddy Soils (part 2): An Improved Method for Determination of FeII.” Journal of the Science of Soil and Manure, Japan 29: 216–220. in Japanese.
- Wang, J., S. Krause, G. Muyzer, M. Meima-Franke, H. J. Laanbroek, and P. L. E. Bodelier. 2012. “Spatial Patterns of Iron- and Methane-oxidizing Bacterial Communities in an Irregularly Flooded, Riparian Wetland.” Frontiers in Microbiology 3 (64). doi:10.3389/fmicb.2012.00064.
- Wang, J., G. Muyzer, P. L. E. Bodelier, and H. J. Laanbroek. 2009. “Diversity of Iron Oxidizers in Wetland Soils Revealed by Novel 16S rRNA Primers Targeting Gallionella-related Bacteria.” The ISME Journal 3: 715–725. doi:10.1038/ismej.2009.7.
- Wang, J., S. Vollrath, T. Behrends, P. L. E. Bodelier, G. Muyzer, M. Meima-Franke, F. D. Oudsten, P. Van Cappellen, and H. J. Laanbroek. 2011. “Distribution and Diversity of Gallionella-like Neutrophilic Iron Oxidizers in a Tidal Freshwater Marsh.” Applied and Environmental Microbiology 77: 2337–2344. doi:10.1128/AEM.02448-10.
- Watanabe, T., M. Kimura, and S. Asakawa. 2007. “Dynamics of Methanogenic Archaeal Communities Based on rRNA Analysis and Their Relation to Methanogenic Activity in Japanese Paddy Field Soils.” Soil Biology & Biochemistry 39: 2877–2887. doi:10.1016/j.soilbio.2007.05.030.
- Watanabe, T., H. Sumida, N. M. Do, K. Yano, S. Asakawa, and M. Kimura. 2013. “Bacterial Consortia in Iron-deposited Colonies Formed on Paddy Soil Surface under Microaerobic Conditions.” Soil Science and Plant Nutrition 59: 337–346. doi:10.1080/00380768.2013.791807.