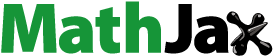
ABSTRACT
To investigate the influence of Azolla (A. filiculoides Lam.) incorporated as a green manure and its subsequent growth as a dual crop with rice on simultaneous methane (CH4) and nitrous oxide (N2O) emissions from a flooded alluvial soil planted with rice, a pot experiment with three treatments, chemical fertilizers (NPK) as the control, incorporation of Azolla as green manure (AGM), and AGM plus basal chemical fertilizers (NPK+AGM) was conducted in Tsuruoka, Yamagata, Japan in 2017. AGM and NPK+AGM treatments significantly increased CH4 emissions at early rice growth stages before 63 days after transplanting (DAT) by 123.0% and 176.7% compared to NPK, respectively. At late rice growth stages (after 63 DAT), only the NPK+AGM treatment significantly increased CH4 emission by 22.1% compared to NPK. However, percentage of CH4 emitted after 63 DAT relative to the seasonal CH4 emission followed the order of NPK (86.2%) > AGM (76.5%) > NPK+AGM (73.3%). Higher CH4 emissions from AGM and NPK+AGM before 63 DAT were attributed to the incorporated Azolla, while the higher emissions after 63 DAT in all treatment groups were ascribed to rice photosynthesis. AGM and NPK+AGM treatments significantly decreased N2O emissions by 71.6% and 81.1% compared to NPK, respectively, at early rice growth stages. Azolla incorporation may have restricted N2O emission from initial soil nitrate before 63 DAT and not have contributed to N2O emissions after 63 DAT. Significantly higher grain yields were observed under the AGM (32.5%) and NPK+AGM (36.3%) compared to NPK. Together, AGM and NPK+AGM treatments significantly increased seasonal CH4 emissions by 31.5% and 43.5%, and decreased seasonal N2O emissions 3.4- and 4.6- fold compared to NPK, respectively. There were no significant differences in the CH4 emissions per grain yield among the treatments; however compared to NPK, AGM and NPK+AGM treatments significantly reduced N2O emissions per grain yield by 78.7% and 84.1%, respectively.
1. Introduction
Methane (CH4) has a global warming potential (GWP) by mass that is 34 times higher than carbon dioxide (CO2) over 100 years and is estimated to account for 15–20% of atmospheric radiative forcing; while nitrous oxide (N2O) is 298 times more potent as a heat trapper than CO2 and may account for 6–8% of radiative forcing in the atmosphere (IPCC Citation2013). As the two most important trace greenhouse gases (GHG) contributing to global warming, and with the continued increase in global temperatures, CH4 and N2O gas emissions have necessitated more research, particularly in paddy ecosystems (Akiyama, Yan, and Yagi Citation2006; Yan et al. Citation2009; Yao et al. Citation2012; Gao et al. Citation2016; Kimani et al. Citation2018).
Rice paddy fields are one of the most important sources of atmospheric CH4 and N2O emissions, with an estimated global CH4 emission rate of 25–60 Tg yr−1 (Reay, Smith, and van Amstel Citation2010), and an annual global N2O contribution of 13–24% (Mosier et al. Citation1998; Olivier et al. Citation1998). Methane is produced through anaerobic degradation of organic matter by archaea and oxidized by methanotrophic bacteria, while N2O originates naturally in soils through microbial processes of nitrification and denitrification (Bouwman Citation1998; Inubushi et al. Citation2003; Cheng et al. Citation2004a, Citation2004b). Although the extent of N2O emissions from rice paddies is much lower than that of CH4, agricultural practices that add nitrogen (N) to soil, such as increased organic and inorganic fertilizers, are major drivers of agricultural soil N2O emissions (Zou et al. Citation2005). Additionally, CH4 and N2O gases are simultaneously emitted from paddy soils depending on the farming system, soil organic matter, soil moisture levels, soil microbial activities, reduction-oxidation status, and fertilizer management practices (i.e. exogenous application of N fertilizers and/or organic materials) (Snyder et al. Citation2009; Nishimura et al. Citation2011). With an estimated demand for increased rice production that must reach 828 million tons by 2025 to satisfy the needs of the rapidly growing population (Kubo and Purevdorj Citation2004), an accompanied increase in CH4 emissions as well as a proportionate increase in N2O emissions following increased N fertilizer use is likely (Bouwman Citation1991; Zou et al. Citation2009). Agricultural GHG fluxes are complex and heterogeneous, and active management strategies to mitigate the combined (or total) CH4 and N2O emissions from paddy fields are needed (Li Citation2007).
Azolla (A. filiculoides) is a free-floating aquatic fern common in lowland rice fields that has been used effectively in paddy fields in Vietnam and southern China as a biofertilizer due to its unique symbiotic relationship with the N-fixing cyanobacteria Anabaena azollae. Azolla is still cultivated in organically managed paddy fields, especially in rice-fish-Azolla or rice-duck-Azolla multi-eco-production systems in Asia (Cagauan, Branckaert, and van Hove Citation2000; Lu and Xia Citation2006; Cheng et al. Citation2015). Additionally, Azolla has recently gained considerable importance for its multifaceted uses (Yadav et al. Citation2014; Kollah, Patra, and Mohanty Citation2016; Shukla et al. Citation2018; Chakraborty et al. Citation2019). Use of Azolla as green manure can either be through its incorporation into paddy soil at the beginning of land preparation before rice transplanting or grown as a dual crop along with rice plants (Xu et al. Citation2017). Dual cropping of Azolla with rice was reported to either increase (Chen et al. Citation1997; Ying et al. Citation2000) or decrease (Bharati et al. Citation2000; Kimani et al. Citation2018) CH4 emission fluxes from flooded rice paddies with varying observations of N2O emissions reported as well (Chen et al. Citation1995; Zou et al. Citation2005; Kimani et al. Citation2018). The lack of a consensus between all these studies is partly attributed to differences in experimental conditions, including different rice cultivars and soil properties. Recent studies have reported the influence of leguminous crop incorporation (e.g. Chinese milk vetch) on CH4 and N2O emissions in paddy fields (Zhu et al. Citation2012; Tang et al. Citation2015); however, the influence of Azolla on simultaneous CH4 and N2O emissions from a flooded paddy ecosystem when incorporated as a green manure or as a dual crop with rice in conjunction with and without chemical fertilizers is not well understood, perhaps only with the exception of Bharati et al. (Citation2000), who reported cumulative CH4 flux in the order of urea > Azolla (incorporated) + urea > Azolla (incorporated + dual crop) > no N control > urea + Azolla (dual crop).
Results from our previous study showed that when Azolla is as a cover crop, CH4 production from submerged rice soil increased but CH4 emissions were significantly suppressed, likely due to increased dissolved oxygen concentration and redox potential at the soil–water interface, which stimulated CH4 oxidation (Kimani et al. Citation2018). Additionally, Azolla cover did not affect N2O emissions due to the denitrification of the initial soil (Kimani et al. Citation2018). Here, to investigate the influence of Azolla incorporation as a green manure and its subsequent growth as a dual crop in conjunction with chemical fertilizers at basal and rice booting stages, on simultaneous CH4 and N2O emissions from a flooded paddy ecosystem, we conducted a pot experiment in the summer of 2017 in Tsuruoka, Yamagata, Japan.
2. Material and methods
2.1. Experiment site, design, and management
This research was conducted in 2017 at the Experiment Farm, Faculty of Agriculture, Yamagata University, Tsuruoka, Yamagata Prefecture located in northeastern Japan (38º44´N, 139º50´E, 16 m elevation). According to the Japan Meteorological Agency database for Tsuruoka Meteorological Observatory (http://www.data.jma.go.jp/obd/stats/etrn/index.php), which is located inside the Experiment Farm, the climatic condition during the rice growth season in 2017 (7 June to 20 September) was 0.1°C hotter and sunnier than the historic average 1981–2010 (Figure S1). The daily average air temperature and sunshine time during the rice growth season were 22.70°C and 6.38 h, while the historic averages of 1981–2010 were 22.60°C and 5.75 h, respectively.
In situ pots were used with three treatments in four replications (Table S1). Azolla filiculoides Lam. was grown in multiplication tanks and incorporated as fresh green manure at 12.2 g Azolla dry weight pot−1 (equivalent to 0.40 g N pot−1) to experimental pots in the AGM and NPK+AGM treatment groups 1 day before rice transplanting. One week after Azolla incorporation, the Azolla started growing and formed a cover (i.e. Azolla dual crop) on the soil surface in the AGM and NPK+AGM treatments. Incorporated Azolla had dry weight concentrations of 339.9 g kg−1 organic C and 33.8 g kg−1 total N. Although Azolla grows in this region during the summer rice-growing season, no native Azolla was available because of the harsh winter conditions; therefore, an introduced species (IRRI code FI 1001) was adopted and grown in the greenhouse for this experiment (Cheng et al. Citation2010; Kimani et al. Citation2018). Haenuki is a popular edible rice cultivar widely grown in local areas of the Yamagata Prefecture and was used for this experiment.
The pot experimental soil was collected from a rice field at the University Farm and classified as an alluvial soil (). The soil was air-dried, sieved through a 5-mm mesh, and mixed well before use. On 26 April 2017, germinated rice seeds were sown in a seedling tray (three seeds per cell) and at 5 weeks after sowing, on 31 May 2017, the seedlings were transplanted (three seedlings per pot) to 12 plastic pots with a 19.5-cm inside diameter, 27-cm height, and 0.2-cm thickness. Prior to transplanting, 7.0 kg (e.g. 4.9 kg dry soil equivalent, 30% water content per total weight of soil) alluvial soil was mixed with 0.87 g KH2PO4 and 0.87 g CO(NH2)2 and used to fill each pot for the NPK and NPK+AGM treatments. The amounts of nitrogen, phosphorus, and potassium of the basal fertilizers were 0.40, 0.20, and 0.25 g pot−1, respectively. At 49 days after transplanting (DAT), the NPK and AGM treatments only were top-dressed with 0.20 g N, 0.10 g P, and 0.13 g K pot−1 by 0.43 g CO(NH2)2 and 0.43 g KH2PO4. At the end of the experiment, the total amounts of applied N, P, and K at field level were 200.9, 100.4, and 127.2 kg per ha, respectively. The flooding water was maintained at approximately 5 cm depth above the soil surface throughout the experimental period by adding tap water. Azolla cover in the AGM and NPK+AGM treatment groups (herein Azolla amended treatments) was maintained throughout the rice growth period and its final dry weight was determined prior to rice harvesting.
Table 1. Major properties of experimental paddy soil.
2.2. CH4 and N2O flux and plant night respiration (CO2 flux) measurement
Whole rice growth period variations in CH4 and N2O flux, and plant nighttime respiration (CO2 flux) from the rice pots were measured as described previously (Kimani et al. Citation2018). Briefly, a 30-mL gas sample was collected at 0, 15, 30 min from each treatment replicate with a 30-mL plastic syringe through a capillary tube at the top of a 1-m closed-top chamber and injected in a 19-mL pre-evacuated vial fitted with a rubber stopper and screw cap. All samples were analyzed at the Institute for Agro-Environmental Sciences, NARO, and the fluxes were calculated from the increase in gas concentration inside the chamber per square meter per hour (Sudo Citation2006; Cheng et al. Citation2008; Kimani et al. Citation2018). Fluxes in the three gases were measured once per week for the first 84 DAT after which, the measurements were taken every 2 weeks and terminated after 112 DATs, which was 1 day before the final rice harvesting (113 DATs).
2.3. Measurement of dissolved CO2 and CH4, and NO3–N in soil solutions
For all treatment groups, soil solutions from each replicate were collected for dissolved CO2, CH4 and NO3–N analysis as reported previously (Kimani et al. Citation2018). Briefly, 9.5 mL soil solution was aspirated into a 19-mL semi-vacuum bottle filled with pure N2 gas at 0.5 atm that was fitted with a rubber stopper and a screw cap, using a 10 cm long microporous polymer tube (2.5 mm outside diameter x 1.5 mm inside diameter) fitted to a PVC tube (50 cm length x 2.7 mm outside diameter x 1.0 mm inside diameter) and inserted vertically into the soil between the rice plant and pot edge at a depth of 10–15 cm 1 day after rice transplanting. CO2 and CH4 were measured in the laboratory with a gas chromatograph (GC-7A, Shimadzu, Kyoto, Japan) with a thermal conductivity detector (TCD) and a flame ionization detector (FID), respectively, and their concentrations were calculated with Henry’s law according to the concentrations of CO2 and CH4 in the headspace (Cheng et al. Citation2005, Citation2006). The NO3–N concentration in soil solutions was analyzed colorimetrically at 450 nm using a UV-1200V spectrophotometer (Shimadzu, Japan).
2.4. Measurement of plant growth, grain yield, and soil c content
Data for rice height and tiller number for each plant per treatment were collected once a week beginning at 8 DAT. At that time, the top rice leaf greenness (SPAD value), which indicates the amount of chlorophyll present, was measured using a SPAD-502 Plus chlorophyll meter (Konica Minolta Inc., Tokyo, Japan). The data from four hills per treatment were averaged during the rice growth period. Above-ground parts of the rice plants were harvested at 113 DAT for all treatments. Before harvest, floating Azolla cover was also collected, oven-dried at 70°C for 2 days for dry weight calculation. After harvest, the soil in the pots was divided into two equal parts from the center of the pot. One part was used for roots sampling (Kimani et al. Citation2018), and the other part for soil property measurements, such as soil pH, EC, SOC and TN content, which were measured using air-dry soil sample procedures (JSSSPN Citation1986). Total dry weight biomass of rice plants included above-ground parts and roots together. Total biomass, grain yield and harvest index for all treatments were determined at harvest as previously described (Amanullah and Inamullah Citation2016). To measure grain yield, rice ears were air-dried for 1 month and grain was carefully threshed.
2.5. Evaluation of net GHG influenced by azolla green manure incorporation
Effects of fresh Azolla incorporation in a flooded paddy soil on global warming potential (GWP), soil C sequestration, and the net GHG balance compared to NPK alone were evaluated. We derived the GWP in g CO2 equivalent per square meter by combining the cumulative CH4 and N2O flux at harvest. In these calculations, the GWP values for CH4 and N2O were considered to be 34 and 298, respectively, over a 100-year time frame (IPCC Citation2013). Global warming potential (GWP) and soil C sequestration were calculated using the following equations, respectively:
where Ctre is soil C content in the treatment after rice cultivation (g kg−1 dry soil), Cbef is the soil C content before the experiment (14.50 g kg−1 dry soil), Sdw is the amount of soil in the pot at the start of the experiment (4.9 kg dry weight), Parea is the pot area. Multiplied by a ratio of molecular weight of CO2 to C (44/12) to calculate the C sequestration in CO2 equivalent. Changes in the net GHG balance following the application of Azolla as a green manure and its subsequent growth as a dual crop with rice were calculated relative to the NPK treatment.
2.6. Statistical analysis
Data were submitted to an analysis of variance and means were compared with the Tukey-test to determine significant differences of the measured plant parameters between the treatments, the emissions of CH4, N2O, night respiration (CO2 flux) and the dissolved CO2, CH4 and NO3–N in soil.
3. Results
3.1. Changes in rice plant growth parameters, grain yield, and azolla cover dry weight
Rice growth period from transplanting to harvesting was about 112 DAT for all treatment groups (NPK, AGM and NPK+AGM). Plant height increased steadily in all setups until the grain-filling stage 77–84 DAT ()). At harvest, the shoot height was 81.8, 88.5, and 84.7 cm for NPK, AGM, and NPK+AGM treatments, respectively. There was no significant difference in rice plant height among NPK and Azolla amended treatments.
Figure 1. Plant height (a), tiller number (b), and leaf color measured in SPAD values (c) of the rice plants in NPK, AGM, and NPK+AGM treatment groups throughout the experiment period. Bars indicate standard deviation (n = 4). The arrow indicates the day fertilizer was added to the pots.
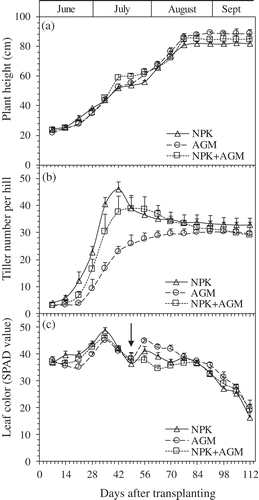
Rice tiller numbers reached a maximum at 42, 91, and 49 DAT for NPK, AGM, and NPK+AGM treatments, respectively ()). The maximum tiller numbers per hill were 46.0, 30.3, and 38.8 for, NPK, AGM, and NPK+AGM treatments, respectively. At harvest, the productive tiller numbers per hill were 32.0, 28.3, and, 32.3 for the NPK, AGM, and NPK+AGM treatments, respectively.
Top rice leaf greenness (i.e. SPAD value) reached its maximum value at 35 DAT, which was 48.6, 45.2, and 45.9 for NPK, AGM, and NPK+AGM, respectively, and then decreased sharply in all three treatments. The leaf greenness in both NPK and AGM treatments increased after top-dressing at 49 DAT ()), while NPK+AGM treatments were not top-dressed and the SPAD value decreased steadily until harvest. The AGM only treatment maintained significantly higher SPAD values (at P < 0.05) compared to NPK and NPK+AGM treatments between 56 and 70 DAT ()).
The total biomasses were 2.55, 2.65, and 2.85 kg m−2 in the NPK, AGM, and NPK+AGM treatment groups, respectively, and were not significantly different from one another. However, Azolla amended treatments maintained significantly higher grain yields of 1.06 kg m−2 for AGM and 1.09 kg m−2 for NPK+AGM, with harvest indexes of 40.27% and 38.30%, respectively, compared to 0.80 kg m−2 and 31.53% for the NPK treatment (). The dry biomasses of the floating Azolla cover in AGM and NPK+AGM treatments at harvest were 0.37 and 0.34 kg m−2, respectively.
Table 2. Total biomass, grain yield, harvest index, cumulative CH4 and N2O emissions during early (before 63 DAT) and late (after 63 DAT) rice growth stages, and total CH4 and N2O emissions per grain yield equivalent among three treatments.
3.2. Changes in CH4 and N2O fluxes, and accumulated emissions
The pattern and intensity of CH4 fluxes from the pot experiment varied with the stage of rice growth ()). The flux rates were relatively low and similar in all treatment groups (0.01–1.46 mg C m−2 h−1) during the initial growth stage (15–35 DAT) and increased with the rice growth. Compared to the NPK treatment, AGM and NPK+AGM treatments had significantly higher fluxes at 42–56 DAT. The NPK and NPK+AGM treatments had single CH4 flux peaks at 77 DAT (42.1 mg C m−2 h−1) and 84 DAT (49.3 mg C m−2 h−1), respectively, while the AGM treatment had two flux peaks, one at 63 DAT (32.0 mg C m−2 h−1) that was slightly higher but not significantly different from the other two treatments (at P= 0.06), and a second peak at 84 DAT (38 .0 mg C m−2 h−1) that was different from the NPK and NPK+AGM treatment groups. Therefore, variations of CH4 emissions from the three treatments in the pot experiment differed significantly during the early stage of rice growth (before 63 DAT) and from heading to maturity stages (after 63 DAT).
Figure 2. Changes in CH4 (a) and N2O (b) fluxes from pots treated with NPK, AGM, and NPK+AGM throughout the experiment period. Bars indicate standard deviation (n = 4). Inset in (b) shows the concentration of NO3–N dissolved in the soil solution during the first 3 weeks on the day of gas sampling.
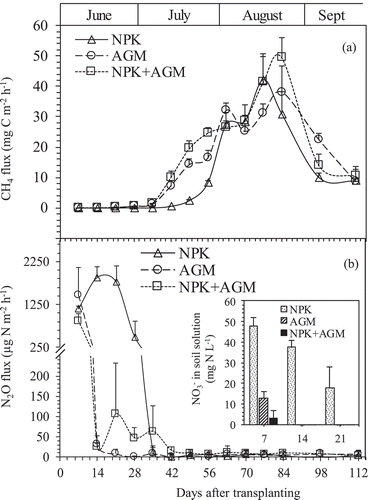
The pattern of seasonal variation in N2O fluxes from rice soil differed from that of CH4 fluxes ()). Fluxes at 7 DAT in the three treatments were not significantly different from each other, with values of 1151.8, 1473.4, and 860.2 µg N m−2 h−1, for NPK, AGM, and NPK+AGM treatments, respectively. After the N2O flux peaks at 7 DAT for AGM and NPK+AGM treatments, N2O fluxes decreased rapidly following the development of anaerobic soil conditions. The NPK treatment that only included inorganic fertilizer had significantly higher N2O flux rates during the initial growth stages (14–28 DAT, 1885.4–496.3 µg N m−2 h−1), compared to AGM (32.1–1.15 µg N m−2 h−1) and NPK+AGM (25.5–47.0 µg N m−2 h−1) treatments. After 42 DAT, the N2O fluxes were very low until rice harvest for all treatments.
The total seasonal average CH4 and N2O emission rates in all treatments were divided into early (before 63 DAT) and late (after 63 DAT) rice growth periods (). Cumulative CH4 emissions varied significantly among the three treatments during the early rice growth stages (4.21, 9.40 and 11.65 g C m−2 for NPK, AGM and NPK+AGM, respectively), while cumulative CH4 emissions were not significantly different between NPK and AGM, or AGM and NPK+AGM during the late rice growth stages (26.25 g C m−2 for NPK, 30.65 g C m−2 for AGM and 32.06 g C m−2 for NPK+AGM). Cumulative N2O emissions during the early growth period were significantly higher for NPK treatment (907.44 mg N m−2) compared to AGM (257.52 mg N m−2), and NPK+AGM (191.09) treatments. No significant N2O emission differences were recorded during the late growth period among the three treatments ().
Over the entire rice growth period, AGM and NPK+AGM recorded significantly higher total cumulative CH4 emissions at 40.05, and 43.71 g C m−2 relative to NPK at 30.46 g C m−2. While NPK recorded significantly higher cumulative N2O emissions at 911.45 mg N m−2 compared to 265.48, and 199.08 mg N m−2, for AGM and NPK+AGM treatments, respectively ().
Total CH4 emission per grain yield was not significantly different among the treatments (P= 0.832); however compared with the NPK treatment, Azolla amended treatments significantly reduced N2O emissions per grain yield from the rice soil (P < 0.001) ().
3.3. Changes in CO2 night respiration
Night respiration was composed mostly of CO2 emitted from rice plant in the NPK treatment and both rice plants and floating Azolla masses in the Azolla amended treatments, which ranged from 18.0 to 577.9 mg C m−2 h−1 during the experimental period and varied with the rice growth stage. The highest peak of nighttime CO2 respiration was observed 6 weeks after rice transplanting in all treatments (Figure S2a). The consecutive small peaks at 84 DAT were attributed to the daytime and high night temperature at sampling (Figure S2b). Throughout the experiment, AGM and NPK+AGM treatments maintained a higher nighttime CO2 respiration compared with the NPK treatment, significantly higher at 42–112 DAT (P < 0.05). The average values of CO2 respiration during the entire experiment period were 220.8, 316.7, and 321.4 mg C m−2 h−1 from NPK, AGM, and NPK+AGM treatments, respectively.
3.4. Changes in CO2, CH4, and NO3–N concentrations in soil solution
Dissolved CO2 in soil solutions of the AGM and NPK+AGM treatments was significantly higher than that in the NPK treatment group at 7–42 DAT (P < 0.01) (). At 28 DAT, significantly higher peaks of dissolved CO2 were observed for the Azolla amended treatments compared with NPK treatment (222.87, 184.99, and 106.49 µg C mL−1 for AGM, NPK+AGM, and NPK, respectively). Dissolved CO2 in soil solutions of all treatments converged at 56 DAT and no further significant observations were made thereafter.
Figure 3. Changes in the concentration of CO2 (a) and CH4 (b) dissolved in the soil solutions in pots treated with NPK, AGM, and NPK+AGM throughout the experiment period. Bars indicate standard deviation (n = 4).
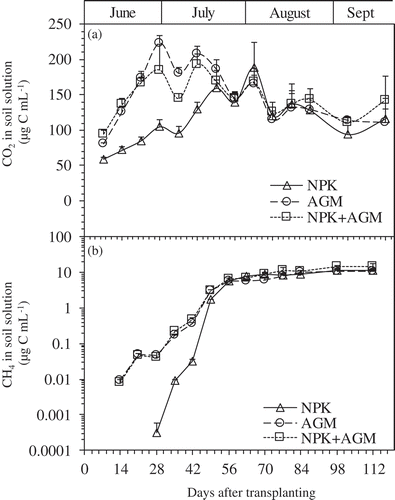
Dissolved CH4 in soil solutions was recorded for AGM and NPK+AGM treatments throughout the rice growth period (7–112 DAT) but was not significantly different. However, significantly lower soil dissolved CH4 concentrations were observed in the NPK treatment compared to the Azolla amended treatments at 7–49 DAT ()). Dissolved CH4 in soil solutions for all treatments increased uniformly with the highest peaks recorded at 112 DAT, which was the last sampling.
The average values of dissolved CO2 concentrations in soil solution during the experimental period were 116.79, 148.92, and 147.39 µg C mL−1, for NPK, AGM, and NPK+AGM treatments, respectively. Additionally, those of soil dissolved CH4 concentrations were 3.81, 3.66, and 4.58 µg C mL−1 for NPK, AGM, and NPK+AGM treatments, respectively.
The NO3–N concentrations in the soil solutions were only detected on 7 DAT in the AGM (12.69 mg N L−1) and NPK+AGM (3.28 mg N L−1) treatments, while the concentrations in the NPK treatment were detected over a period of 3 weeks and were 47.78, 37.49, and 17.98 mg N L−1 for 7, 14, and 21 DAT, respectively (inset )).
3.5. Global warming potential, soil C sequestration, and net greenhouse gas balance
Regardless of the treatment setup and management, CH4 was the most influential GHG that contributed to the bulk of the total combined GWP in early and late rice growth stage emissions (). Azolla amended treatments showed significantly higher combined GWP by the CH4 and N2O emission values relative to NPK treatment (1807.97, 1939.90, and 2074.81 g CO2 equivalent m−2, for NPK, AGM, and NPK+AGM, respectively). However, the soil C sequestered in the Azolla amended treatments was significantly higher (1821.17 and 1955.92 g CO2 equivalent m−2 for AGM and NPK+AGM treatments, respectively) compared to NPK treatment (1.63 g CO2 equivalent m−2). Subsequently, the Azolla amended treatments showed significantly lower net GHG balance when evaluating the difference between combined GWP by the CH4 and N2O emissions and amount of soil C sequestered (118.73 and 118.89 g CO2 equivalent m−2 for AGM and NPK+AGM treatments, respectively) relative to the NPK treatment (1806.34 g CO2 equivalent m−2) (). There were no significant differences between the Azolla amended treatments for the combined GWP values that were measured by the CH4 and N2O emissions, amount of soil C sequestered, and the net GHG balance.
Table 3. The net CO2-equivalent greenhouse gas emissions balance from CH4 and N2O emissions (positive), and soil C sequestration (negative) among three treatments.
4. Discussion
4.1. Effects of azolla incorporation on CH4 emissions
In this experiment, incorporation of Azolla as a green manure plus its subsequent growth as a cover crop in conjunction with chemical fertilizers (NPK) either at basal or top-dressing, significantly stimulated the total cumulative CH4 emissions throughout the rice growth period (112 DAT) by 31.5% and 43.5% with AGM and NPK+AGM treatments, compared to the NPK treatment, respectively (). Contrary to our previous research, where Azolla covers without incorporation significantly suppressed CH4 emission from flooded rice soil (Kimani et al. Citation2018), the emergence of Azolla as a cover following incorporation of Azolla as a green manure in this study did not suppress CH4 emissions from AGM and NPK+AGM treatments, either during the early (before 63 DAT) or late (after 63 DAT) rice growth stages and the subsequent total cumulative CH4 emissions (), ). These stimulating effects were consistent with previous findings that Azolla, either incorporated or as a dual crop with rice, increased CH4 emissions from rice paddy soil pathways (Chen et al. Citation1997; Adhya et al. Citation2000; Ying et al. Citation2000), which was most likely due to the decomposition of the organic amendments by incorporated Azolla.
During the early rice growth stages before 63 DAT, no significant differences on rice growth parameters among all treatments were observed (,); however, dissolved CO2 until 49 DAT and CH4 concentrations in soil solutions until 63 DAT were significantly higher in the Azolla amended treatments compared to NPK, with no significant differences between the two Azolla amended treatments (,). During the early growth period, cumulative CH4 emissions significantly increased in the Azolla amended treatments 123.3% (AGM) and 176.7% (NPK+AGM) compared to NPK (). These results were consistent with previous reports of increased CH4 emissions of approximately 60% within 40 DAT from milk vetch and/or rye amended plots relative to NPK only (Kim et al. Citation2013). Therefore, CH4 emissions in Azolla amended treatments before 63 DAT are likely attributed to readily available carbon substrates following incorporation of Azolla as green manure.
During the late rice growth stages after 63 DAT, the cumulative CH4 emissions from all treatments were largely higher than those from early rice growth stages before 63 DAT, indicating probable changes of CH4 carbon sources from initial soil organic matter and incorporated Azolla, to the photosynthetic products of the rice plants (Minoda, Kimura, and Wada Citation1996; Inubushi et al. Citation2003). As for the cumulative CH4 emission, although the ratio to the total emission was highest in the NPK treatment (86.2%), the amount in the NPK treatment (26.25 g C m−2) was lower than those in the Azolla amended treatments (). Huang, Sass, and Fisher (Citation1997) reported that more than 75% of the total seasonal CH4 in a permanent flooding rice ecosystem was released during the rice reproductive and ripening stages without any organic matter incorporation. These results also showed that the carbon ratio of CH4 emission to net photosynthetic production was about 8% during ripening periods, which was higher than that measured during the vegetative periods (about 1 ~ 2%). Moreover, the ratio was strongly dependent on plant biomass among the different rice cultivars tested. The highly significant and positive relationship explored in this and other studies between night CH4 emission fluxes and night rice plant respiration (CO2 emission) after 63 DAT in all tested treatments () also suggests that rice photosynthesis supplies carbon substrates for methanogens in the rice soil (Aulakh et al. Citation2001b; Sass and Cicerone Citation2002).
Figure 4. Relationship between daily CH4 flux and night respiration (CO2 emission) among NPK, AGM, and NPK+AGM treatments throughout after 9 weeks rice transplanting (n = 6).
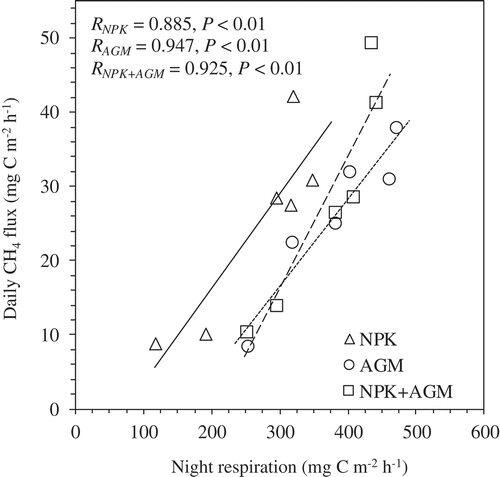
In the current study, cumulative CH4 emission after 63 DAT in the AGM treatment was not significantly different from the NPK treatment (), which suggests that incorporated Azolla as a green manure at the beginning of the experiment, its subsequent growth as a cover, and the consequent observation of masses of dead Azolla cover (not quantified) at harvest neither promoted nor restricted CH4 emission at the late rice growth stages. These observations are consistent with those by Zhu et al. (Citation2012).
The carbon sources for CH4 production in rice paddies can be native soil organic matter, incorporated organic materials such as rice straw and manure, and new carbon substrate from plant growth such as plant debris and root exudates (Nakajima et al. Citation2016; Wang et al. Citation2016). In this experiment, with the exception of the incorporated Azolla in the Azolla amended treatments, the soil had no initial visible plant residues. Although the amount of CH4 dissolved in the soil solution in the Azolla amended treatments significantly increased with increasing rice growth until 35 DAT, showing significantly lower soil solution dissolved CH4 in the NPK treatment ()), aboveground CH4 fluxes before 35 DAT were not detected or were relatively low during the early rice growth period ()). This result implies that the native soil organic matter was not the main source of CH4 production.
Methane fluxes increased significantly between 42 and 56 DAT for the Azolla amended treatments relative to the NPK treatment ()). During the same period, the highest peaks of plant night respiration in the treatments were recorded (Figure S2a). Similarly, the highest CH4 emission peaks for all treatments occurred at 77 DAT for NPK and 84 DAT for both Azolla amended treatments, while additional smaller peaks of plant night respiration were recorded (), and S2a). These results indicate that the sources of CH4 production from the three treatments were less from the old matter from the native soil organic and incorporated Azolla green manure, and mostly from new carbon through rice plant root exudates and rice plant debris in all three treatment groups, which significantly contributed to cumulative CH4 emissions after 63 DAT. These observations were consistent with what was reported previously (Chen et al. Citation1997; Ying et al. Citation2000; Cheng et al. Citation2006, Citation2008; Lou et al. Citation2008).
On average, Azolla amended treatments in conjunction with chemical fertilizers used either as basal or top-dressing (both AGM and NPK+AGM) significantly increased rice grain yield by 34.4% and harvest index by 24.8% relative to NPK treatment (). Generally, reducing CH4 emission per yield equivalent is a major challenge worldwide (Gao et al. Citation2015). In the current study, the values of CH4 emission per grain yield in Azolla amended treatments were 38.08 and 40.07 g C kg−1 for AGM and NPK+AGM, respectively, which were not significantly different from 38.23 g C kg−1 measured in NPK. Since incorporated Azolla can decrease chemical fertilizer application in rice production, no change in CH4 emission per yield equivalent in this study implies that Azolla incorporation as green manure is an alternative technique for sustainable rice production.
4.2. Effects of azolla incorporation on N2O emissions
Nitrous oxide emission is a microbial-mediated soil process in agricultural soils that acts through the nitrification-denitrification pathway (Butterbach-Bahl et al. Citation2013). Application of inorganic N fertilizers increases N2O efflux by increasing substrate availability for nitrification and denitrification (Yao et al. Citation2012). Additionally, adequate amounts of NO3–N and availability of carbon (C) that are susceptible to mineralization either from native organic matter or newly incorporated crop residues are major factors in controlling denitrification, especially under-limited oxygen conditions (Aulakh et al. Citation1991a, Citation2001a).
Our results showed that combined fresh Azolla incorporation and dual cropping significantly inhibited N2O emission from flooded paddy soil compared with NPK treatment (), ). Despite the significant differences in the dissolved soil NO3–N concentrations among the treatments at 7 DAT (inset )), N2O fluxes did not differ at 7 DAT among the treatments (at P= 0.137). Following the initial N2O flux peaks for all treatments at 7 DAT, NPK treatment had prolonged and significantly higher N2O fluxes 14, 21, and 28 DAT compared to Azolla amended treatments, whose fluxes dropped rapidly to relatively low emission values (<106.53 µg N m−2 h−1). Moreover, significantly higher dissolved soil NO3–N concentrations were recorded for the NPK treatment at 14, 21 and 28 DAT compared to the Azolla amended treatments (), inset )). The cumulated total N2O emissions during the entire rice growth period (112 DAT) were significantly lower in the Azolla amended treatments compared to NPK treatments (). Cumulatively high N2O emissions were recorded during the early rice growth stages (before 63 DAT) in all treatments and on average, at the early rice growth stages, NPK treatment emitted 99.5% N2O of all growth stages compared to the Azolla amended treatments (97.0% AGM and 96.0% NPK+AGM). There were no significant N2O emission differences during the late rice growth stages among the three treatments. During the entire rice growth period, AGM and NPK+AGM treatments decreased seasonal N2O emissions by 3.4 (70.9%), and 4.6 (78.2%) fold relative to NPK treatment, respectively ().
Although we may have omitted the initial high N2O emission peaks (e.g. at 2–5 days after soil flooding) from Azolla amended treatments and/or NPK treatments, lower N2O emission fluxes during the early rice growth stages from Azolla amended treatments compared to NPK may have been due to a more effective reduction of N2O to N2 during denitrification as a result of the availability of readily decomposable organic matter, in this case the incorporated Azolla. Availability of organic C has previously been reported to be a critical factor for denitrification (Aulakh et al. Citation1991b; Baruah and Baruah Citation2015).
Unlike in the NPK treatment where high N2O fluxes (2–4 weeks after transplanting) were in tandem with the decrease in soil dissolved NO3–N concentrations, Azolla amended treatments maintained significantly low N2O fluxes and dissolved NO3–N concentrations (), insert )). This suggests that N2O emissions at the early rice growth stage for all treatments were probably a result of high initial soil NO3–N levels. The experimental soil was stored outside for 1 year before the start of the experiment and a substantial amount of decomposable organic C and N may have already been converted into CO2 and NO3–N, respectively. The amount of NO3–N in the initial soil was 101.6 mg N kg−1 in 4.9 kg of dry soil (). These observations were consistent with those by Cheng et al. (Citation2006) and Kimani et al. (Citation2018). These results favor the conclusion that the incorporation of Azolla green manure as an N source may decrease N2O emissions in flooded paddy soils. The combination of green manure and urea has been reported to mitigate urea N loss through N2O flux (Zhu et al. Citation2012).
4.3. Effects of azolla incorporation on GWP, soil c sequestration and net GHG balance
According to Johnson et al. (Citation2007), global warming potential (GWP) represents the expected effect on the radiation balance of the earth due to the addition of a unit of gas as a result of the specific gas mean lifetime and total quantity emitted. The major gases contributing to positive radiative forcing in the atmosphere are CO2, CH4 and N2O (IPCC Citation2013). Compared to the NPK treatment, incorporation of Azolla as a green manure plus its subsequent growth as a dual crop with rice significantly increased the combined GWP (CH4 and N2O emissions) in AGM (7.3%) and NPK+AGM (14.8%) treatments, respectively (). This result was mainly attributed to the significantly higher seasonal CH4 emissions from the Azolla amended treatments compared to NPK treatment (). Methane emissions have been reported to be a key factor in determining the combined GWP during rice cultivation (Hwang et al. Citation2017; Setyanto et al. Citation2018; Tirol-Padre et al. Citation2018). As the contribution of N2O to the combined GWP was very small in the flooded paddy soil condition irrespective of the treatment management, effective control of CH4 emissions could be a useful mitigation approach to reducing total GHG emissions and hence, the subsequent combined GWP (Kim et al. Citation2013). Despite the high combined GWP from Azolla amended treatments, incorporated Azolla as green manure significantly increased soil organic carbon (SOC) content during the rice growth period by 23.9% on average for AGM and NPK+AGM treatments compared to NPK treatment (Table S2). The soil C sequestration values in the AGM and NPK+AGM treatments were significantly higher than those measured in the NPK only treatment, and reduced net GHG balance values in the Azolla amended treatments compared to the NPK only treatment (). Management practices that increase organic inputs (e.g. biomass and manure) have been reported to enhance microbial functions and SOC sequestration (Jarecki and Lal Citation2003). While incorporation of Azolla as green manure and its subsequent growth as a dual crop in the flooded paddy soil with rice in this study increased the soil C sequestration relative to NPK treatment, the significantly high combined GWP from the Azolla amended treatments () overshadowed the benefits of its SOC content increase. In spite of this, significant increases in grain yield, and decreases in N2O and no significant CH4 emissions per grain yield at harvest in the Azolla amended treatments compared to NPK treatment () is a positive result. In addition, the cultivation and incorporation of Azolla is a process involving C accumulation from the atmosphere to the soil, while the production of synthetic N fertilizer consumes fossil fuels that release C and contribute to GHG emissions. Thus, in an effort to mitigate climate change, especially in organic farming practices, further long-term studies on field conditions are needed to not only quantify and recommend the best approaches for Azolla incorporation as a green manure and cover in conjunction with or without chemical fertilizers, but also to quantitatively evaluate changes in soil C storage related to organic matter application.
5. Conclusion
To determine whether Azolla incorporation as green manure (AGM) and its subsequent growth as a dual crop in conjunction with chemical fertilizers (NPK) either as basal or top-dressing affects both CH4 and N2O emissions from flooded paddy ecosystems, a pot experiment was carried out in Tsuruoka, Yamagata, Japan in 2017. Significantly higher CH4 emissions from Azolla amended treatments (AGM and NPK+AGM) were observed in the early rice growth stage and attributed to the incorporated Azolla, while those at the late growth stage were a result of plant photosynthesis due to increased rice biomass. On average, AGM amendment increased rice yield by 34.4% and seasonal CH4 emission by 37.5% but decreased N2O emission by 74.5% relative to the NPK only treatment. Azolla amended treatments significantly increased total grain yield, global warming potential, soil organic content in the soil and decreased the net GHG balance. It should be noted that our results are based on a pot experiment, and field studies should be carried out in future to confirm its application to farming conditions.
SR2_20191122.docx
Download MS Word (253.8 KB)Acknowledgments
We thank all members in the Laboratory of Soil Science and Plant nutrition, Yamagata University, and at the Institute for Agro-Environmental Sciences, NARO, Tsukuba, Japan, for their help in the field and laboratory measurements.
Disclosure statement
No potential conflict of interest was reported by the authors.
Supplementary material
Supplemental data for this article can be accessed here.
Additional information
Funding
References
- Adhya, T. K., K. Bharati, S. R. Mohanty, B. Ramakrishnan, V. R. Rao, N. Sethunathan, and R. Wassmann. 2000. “Methane Emission from Rice Fields at Cuttack, India.” Nutrient Cycling in Agroecosystems 58 (1): 95–105. doi:10.1023/A:1009886317629.
- Akiyama, H., X. Yan, and K. Yagi. 2006. “Estimations of Emission Factors for Fertilizer-Induced Direct N2O Emissions from Agricultural Soils in Japan: Summary of Available Data.” Soil Science and Plant Nutrition 52 (6): 774–787. doi:10.1111/j.1747-0765.2006.00097.x.
- Amanullah, and Inamullah. 2016. “Dry Matter Partitioning and Harvest Index Differ in Rice Genotypes with Variable Rates of Phosphorus and Zinc Nutrition.” Rice Science 23 (2): 78–87. doi:10.1016/j.rsci.2015.09.006.
- Aulakh, M. S., J. W. Doran, D. T. Walters, and J. F. Power. 1991a. “Legume Residue and Soil Water Effects on Denitrification in Soils of Different Textures.” Soil Biology and Biochemistry 23 (12): 1161–1167. doi:10.1016/0038-0717(91)90029-J.
- Aulakh, M. S., T. S. Khera, J. W. Doran, and K. F. Bronson. 2001a. “Denitrification, N2O and CO2 Fluxes in Rice-Wheat Cropping System as Affected by Crop Residues, Fertilizer N and Legume Green Manure.” Biology and Fertility of Soils 34 (6): 375–389. doi:10.1007/s003740100420.
- Aulakh, M. S., D. T. Walters, J. W. Doran, D. D. Francis, and A. R. Mosier. 1991b. “Crop Residue Type and Placement Effects on Denitrification and Mineralization.” Soil Science Society of America Journal 55 (4): 1020. doi:10.2136/sssaj1991.03615995005500040022x.
- Aulakh, M. S., R. Wassmann, C. Bueno, and H. Rennenberg. 2001b. “Impact of Root Exudates of Different Cultivars and Plant Development Stages of Rice (Oryza Sativa L.) On Methane Production in a Paddy Soil.” Plant and Soil 230 (1): 77–86. doi:10.1023/A:1004817212321.
- Baruah, A., and K. K. Baruah. 2015. “Organic Manures and Crop Residues as Fertilizer Substitutes: Impact on Nitrous Oxide Emission, Plant Growth and Grain Yield in Pre-Monsoon Rice Cropping System.” Journal of Environmental Protection 06 (07): 755–770. doi:10.4236/jep.2015.67069.
- Bharati, K., S. R. Mohanty, D. P. Singh, V. R. Rao, and T. K. Adhya. 2000. “Influence of Incorporation or Dual Cropping of Azolla on Methane Emission from a Flooded Alluvial Soil Planted to Rice in Eastern India.” Agriculture, Ecosystems and Environment 79 (1): 73–83. doi:10.1016/S0167-8809(99)00148-6.
- Bouwman, A. F. 1991. “Agronomic Aspects of Wetland Rice Cultivation and Associated Methane Emissions.” Biogeochemistry 15 (2): 65–88. doi:10.1007/BF00003218.
- Bouwman, A. F. 1998. “Environmental Science: Nitrogen Oxides and Tropical Agriculture.” Nature 392 (6679): 866–867. doi:10.1038/31809.
- Butterbach-Bahl, K., E. M. Baggs, M. Dannenmann, R. Kiese, and S. Zechmeister-Boltenstern. 2013. “Nitrous Oxide Emissions from Soils: How Well Do We Understand the Processes and Their Controls?” Philosophical Transactions of the Royal Society B: Biological Sciences 368 (1621): 20130122. doi:10.1098/rstb.2013.0122.
- Cagauan, A. G., R. D. Branckaert, and C. van Hove. 2000. “Integrating Fish and Azolla into Rice-Duck Farming in Asia.” Naga, the ICLARM Quarterly 23: 4–10.
- Chakraborty, S., A. Mishra, E. Verma, B. Tiwari, A. K. Mishra, and S. S. Singh. 2019. “Physiological Mechanisms of Aluminum (Al) Toxicity Tolerance in Nitrogen-Fixing Aquatic Macrophyte Azolla Microphylla Kaulf: Phytoremediation, Metabolic Rearrangements, and Antioxidative Enzyme Responses.” Environmental Science and Pollution Research February. doi:10.1007/s11356-019-04408-7.
- Chen, G. X., G. Huang, B. Huang, J. Wu, K. W. Yu, H. Xu, X. Xue, and Z. Wang. 1995. “CH4 and N2O Emission from a Rice Field and Effect of Azolla and Fertilization on Them.” Chinese Journal of Applied Ecology 6 (4): 378–382.
- Chen, G. X., G. H. Huang, B. Huang, K. W. Yu, J. Wu, and H. Xu. 1997. “Nitrous Oxide and Methane Emissions from Soil–Plant Systems.” Nutrient Cycling in Agroecosystems 49 (1): 41–45. doi:10.1023/A:1009758900629.
- Cheng, W., Y. Okamoto, M. Takei, K. Tawaraya, and H. Yasuda. 2015. “Combined Use of Azolla and Loach Suppressed Weed Monochoria Vaginalis and Increased Rice Yield without Agrochemicals.” Organic Agriculture 5 (1): 1–10. doi:10.1007/s13165-015-0097-3.
- Cheng, W., H. Sakai, A. Hartley, K. Yagi, and T. Hasegawa. 2008. “Increased Night Temperature Reduces the Stimulatory Effect of Elevated Carbon Dioxide Concentration on Methane Emission from Rice Paddy Soil.” Global Change Biology 14 (3): 644–656. doi:10.1111/j.1365-2486.2007.01532.x.
- Cheng, W., H. Sakai, M. Matsushima, K. Yagi, and T. Hasegawa. 2010. “Response of the Floating Aquatic Fern Azolla Filiculoides to Elevated CO2, Temperature, and Phosphorus Levels.” Hydrobiologia 656 (1): 5–14. doi:10.1007/s10750-010-0441-2.
- Cheng, W., H. Tsuruta, G. Chen, H. Akiyama, and K. Yagi. 2004a. “N2O and N2 Production Potential in Various Chinese Agricultural Soils by Denitrification.” Soil Science and Plant Nutrition 50 (6): 909–915. doi:10.1080/00380768.2004.10408553.
- Cheng, W., H. Tsuruta, G. Chen, and K. Yagi. 2004b. “N2O and NO Production in Various Chinese Agricultural Soils by Nitrification.” Soil Biology and Biochemistry 36 (6): 953–963. doi:10.1016/j.soilbio.2004.02.012.
- Cheng, W., K. Yagi, X. Hua, H. Sakai, and K. Kobayashi. 2005. “Influence of Elevated Concentrations of Atmospheric CO2 on CH4 and CO2 Entrapped in Rice-Paddy Soil.” Chemical Geology 218 (1–2): 15–24. doi:10.1016/j.chemgeo.2005.01.016.
- Cheng, W., K. Yagi, H. Sakai, and K. Kobayashi. 2006. “Effects of Elevated Atmospheric CO2 Concentrations on CH4 and N2O Emission from Rice Soil: An Experiment in Controlled-Environment Chambers.” Biogeochemistry 77 (3): 351–373. doi:10.1007/s10533-005-1534-2.
- Gao, B., J. Xiaotang, Q. Meng, Z. Cui, P. Christie, X. Chen, and F. Zhang. 2015. “The Impact of Alternative Cropping Systems on Global Warming Potential, Grain Yield and Groundwater Use.” Agriculture, Ecosystems and Environment 203 (May): 46–54. doi:10.1016/j.agee.2015.01.020.
- Gao, X., L. Aimin, S. Wang, S. Liantai, P. Zhou, and A. Yuan. 2016. “Greenhouse Gas Intensity and Net Ecosystem Carbon Budget following the Application of Green Manures in Rice Paddies.” Nutrient Cycling in Agroecosystems 106 (2): 169–183. doi:10.1007/s10705-016-9797-7.
- Huang, Y., R. Sass, and F. Fisher. 1997. “Methane Emission from Texas Rice Paddy Soils. 2. Seasonal Contribution of Rice Biomass Production to CH4 Emission.” Global Change Biology 3 (6): 491–500. doi:10.1046/j.1365-2486.1997.00106.x.
- Hwang, H. Y., G. W. Kim, S. Y. Kim, M. M. Haque, M. I. Khan, and P. J. Kim. 2017. “Effect of Cover Cropping on the Net Global Warming Potential of Rice Paddy Soil.” Geoderma 292 (April): 49–58. doi:10.1016/j.geoderma.2017.01.001.
- Inubushi, K., W. Cheng, M. M. Shinichi Aonuma, K. K. Hoque, S. Miura, H. Y. Kim, and M. Okada. 2003. “Effects of Free-Air CO2 Enrichment (FACE) on CH4 Emission from a Rice Paddy Field.” Global Change Biology 9 (10): 1458–1464. doi:10.1046/j.1365-2486.2003.00665.x.
- IPCC. 2013. “Working Group I Contribution to the Fifth Assessment Report of the Intergovernmental Panel on Climate Change.” In Climate Change 2013 – The Physical Science Basis by Intergovernmental Panel on Climate Change, edited by T. F. Stocker, D. Qin, G. K. Plattner, M. Tignor, S. K. Allen, J. Boschung, A. Nauels, Y. Xia, V. Bex, and P. M. Midgley, 1535. Cambridge, United Kingdom and New York, NY, USA: Cambridge University Press. doi:10.1017/CBO9781107415324.
- Jarecki, M. K., and R. Lal. 2003. “Crop Management for Soil Carbon Sequestration.” Critical Reviews in Plant Sciences 22 (6): 471–502. doi:10.1080/713608318.
- Johnson, J. M.-F., A. J. Franzluebbers, S. L. Weyers, and D. C. Reicosky. 2007. “Agricultural Opportunities to Mitigate Greenhouse Gas Emissions.” Environmental Pollution 150 (1): 107–124. doi:10.1016/j.envpol.2007.06.030.
- JSSSPN. 1986. Soil Normal Analysis Methods, Hakuyusha Press, Tokyo (In Japanese). Tokyo: Japanese Society of Soil Science and Plant Nutrition.
- Kim, S. Y., C. H. Lee, J. Gutierrez, and P. J. Kim. 2013. “Contribution of Winter Cover Crop Amendments on Global Warming Potential in Rice Paddy Soil during Cultivation.” Plant and Soil 366 (1): 273–286. doi:10.1007/s11104-012-1403-4.
- Kimani, S. M., W. Cheng, T. Kanno, T. Nguyen-Sy, R. Abe, A. Z. Oo, K. Tawaraya, and S. Sudo. 2018. “Azolla Cover Significantly Decreased CH4 but Not N2O Emissions from Flooding Rice Paddy to Atmosphere.” Soil Science and Plant Nutrition 64 (1): 68–76. doi:10.1080/00380768.2017.1399775.
- Kollah, B., A. K. Patra, and S. R. Mohanty. 2016. “Aquatic Microphylla Azolla: A Perspective Paradigm for Sustainable Agriculture, Environment and Global Climate Change.” Environmental Science and Pollution Research 23 (5): 4358–4369. doi:10.1007/s11356-015-5857-9.
- Kubo, M., and M. Purevdorj. 2004. “The Future of Rice Production and Consumption.” Journal of Food Distribution Research 35 (1): 128–142.
- Li, C. 2007. “Quantifying Greenhouse Gas Emissions from Soils: Scientific Basis and Modeling Approach.” Soil Science and Plant Nutrition 53 (4): 344–352. doi:10.1111/j.1747-0765.2007.00133.x.
- Lou, Y., K. Inubushi, T. Mizuno, T. Hasegawa, Y. Lin, H. Sakai, W. Cheng, and K. Kobayashi. 2008. “CH4 Emission with Differences in Atmospheric CO2 Enrichment and Rice Cultivars in a Japanese Paddy Soil.” Global Change Biology 14 (11): 2678–2687. doi:10.1111/j.1365-2486.2008.01665.x.
- Lu, J., and L. Xia. 2006. “Review of Rice–Fish-Farming Systems in China — One of the Globally Important Ingenious Agricultural Heritage Systems (GIAHS).” Aquaculture 260 (1): 106–113. doi:10.1016/j.aquaculture.2006.05.059.
- Minoda, T., M. Kimura, and E. Wada. 1996. “Photosynthates as Dominant Source of CH4 and CO2 in Soil Water and CH4 Emitted to the Atmosphere from Paddy Fields.” Journal of Geophysical Research: Atmospheres 101 (D15): 21091–21097. doi:10.1029/96JD01710.
- Mosier, A., C. Kroeze, C. Nevison, O. Oenema, S. Seitzinger, and V. Cleemput. 1998. “Closing the Global N2O Budget: Nitrous Oxide Emissions through the Agricultural Nitrogen Cycle.” Nutrient Cycling in Agroecosystems 55 (2–3): 225–248. doi:10.1023/A:1009740530221.
- Nakajima, M., W. Cheng, S. Tang, Y. Hori, E. Yaginuma, S. Hattori, S. Hanayama, K. Tawaraya, and X. Xingkai. 2016. “Modeling Aerobic Decomposition of Rice Straw during the Off-Rice Season in an Andisol Paddy Soil in a Cold Temperate Region of Japan: Effects of Soil Temperature and Moisture.” Soil Science and Plant Nutrition 62 (1): 90–98. doi:10.1080/00380768.2015.1121116.
- Nishimura, S., H. Akiyama, S. Sudo, T. Fumoto, W. Cheng, and K. Yagi. 2011. “Combined Emission of CH4 and N2O from a Paddy Field Was Reduced by Preceding Upland Crop Cultivation.” Soil Science and Plant Nutrition 57 (1): 167–178. doi:10.1080/00380768.2010.551346.
- Olivier, J. G. J., A. F. Bouwman, K. W. Van der Hoek, and J. J. M. Berdowski. 1998. “Global Air Emission Inventories for Anthropogenic Sources of NOx, NH3 and N2O in 1990.” Environmental Pollution Nitrogen, the Confer-N-s First International Nitrogen Conference 1998 102 (1, Supplement 1): 135–148. doi:10.1016/S0269-7491(98)80026-2.
- Reay, D., P. Smith, and A. van Amstel, eds. 2010. Methane and Climate Change. London; Washington, DC: Earthscan.
- Sass, R. L., and R. J. Cicerone. 2002. “Photosynthate Allocations in Rice Plants: Food Production or Atmospheric Methane?” Proceedings of the National Academy of Sciences of the United States of America 99 (19): 11993–11995. doi:10.1073/pnas.202483599.
- Setyanto, P., A. Pramono, T. A. Adriany, H. L. Susilawati, T. Tokida, A. T. Padre, and K. Minamikawa. 2018. “Alternate Wetting and Drying Reduces Methane Emission from a Rice Paddy in Central Java, Indonesia without Yield Loss.” Soil Science and Plant Nutrition 64 (1): 23–30. doi:10.1080/00380768.2017.1409600.
- Shukla, M., A. Bhattacharyya, P. K. Shukla, D. Roy, B. Yadav, and R. Sirohi. 2018. “Effect of Azolla Feeding on the Growth, Feed Conversion Ratio, Blood Biochemical Attributes and Immune Competence Traits of Growing Turkeys.” Veterinary World 11 (4): 459–463. doi:10.14202/vetworld.2018.459-463.
- Snyder, C. S., T. W. Bruulsema, T. L. Jensen, and P. E. Fixen. 2009. “Review of Greenhouse Gas Emissions from Crop Production Systems and Fertilizer Management Effects.” Agriculture, Ecosystems and Environment, Reactive Nitrogen in Agroecosystems: Integration with Greenhouse Gas Interactions 133 (3): 247–266. doi:10.1016/j.agee.2009.04.021.
- Sudo, S. 2006. “Method and Instrument for Measuring Atmospheric Gas.” Industrial Property Digital Library, Patent of Japan (No. 2006–275844).
- Tang, H., X. Xiao, W. Tang, K. Wang, J. Sun, L. Weiyan, and G. Yang. 2015. “Effects of Winter Covering Crop Residue Incorporation on CH4 and N2O Emission from Double-Cropped Paddy Fields in Southern China.” Environmental Science and Pollution Research 22 (16): 12689–12698. doi:10.1007/s11356-015-4557-9.
- Tirol-Padre, A., K. Minamikawa, T. Tokida, R. Wassmann, and K. Yagi. 2018. “Site-Specific Feasibility of Alternate Wetting and Drying as A Greenhouse Gas Mitigation Option in Irrigated Rice Fields in Southeast Asia: A Synthesis.” Soil Science and Plant Nutrition 64 (1): 2–13. doi:10.1080/00380768.2017.1409602.
- Wang, W., D. Y. F. Lai, C. Wang, C. Tong, and C. Zeng. 2016. “Effects of Inorganic Amendments, Rice Cultivars and Cultivation Methods on Greenhouse Gas Emissions and Rice Productivity in a Subtropical Paddy Field.” Ecological Engineering 95 (October): 770–778. doi:10.1016/j.ecoleng.2016.07.014.
- Xu, H., B. Zhu, J. Liu, L. Dengyun, Y. Yang, K. Zhang, Y. Jiang, H. Yuegao, and Z. Zeng. 2017. “Azolla Planting Reduces Methane Emission and Nitrogen Fertilizer Application in Double Rice Cropping System in Southern China.” Agronomy for Sustainable Development 37 (4): 29. doi:10.1007/s13593-017-0440-z.
- Yadav, R. K., G. Abraham, Y. V. Singh, and P. K. Singh. 2014. “Advancements in the Utilization of Azolla-Anabaena System in Relation to Sustainable Agricultural Practices.” Proceedings of the Indian National Science Academy 80 (2): 301. doi:10.16943/ptinsa/2014/v80i2/55108.
- Yan, X., H. Akiyama, K. Yagi, and H. Akimoto. 2009. “Global Estimations of the Inventory and Mitigation Potential of Methane Emissions from Rice Cultivation Conducted Using the 2006 Intergovernmental Panel on Climate Change Guidelines.” Global Biogeochemical Cycles 23 (2): GB2002. doi:10.1029/2008GB003299.
- Yao, Z., X. Zheng, H. Dong, R. Wang, B. Mei, and J. Zhu. 2012. “A 3-Year Record of N2O and CH4 Emissions from A Sandy Loam Paddy during Rice Seasons as Affected by Different Nitrogen Application Rates.” Agriculture, Ecosystems and Environment 152 (May): 1–9. doi:10.1016/j.agee.2012.02.004.
- Ying, Z., P. Boeckx, G. X. Chen, and O. Van Cleemput. 2000. “Influence of Azolla on CH4 Emission from Rice Fields.” Nutrient Cycling in Agroecosystems 58 (1): 321–326. doi:10.1023/A:1009871308968.
- Zhu, B., Y. Li-xia, H. Yue-gao, Z.-H. Zeng, H.-M. Tang, G.-L. Yang, and X.-P. Xiao. 2012. “Effects of Chinese Milk Vetch (Astragalus Sinicus L.) Residue Incorporation on CH4 and N2O Emission from a Double-Rice Paddy Soil.” Journal of Integrative Agriculture 11 (9): 1537–1544. doi:10.1016/S2095-3119(12)60154-3.
- Zou, J., Y. Huang, J. Jiang, X. Zheng, and R. L. Sass. 2005. “A 3-Year Field Measurement of Methane and Nitrous Oxide Emissions from Rice Paddies in China: Effects of Water Regime, Crop Residue, and Fertilizer Application.” Global Biogeochemical Cycles 19 (2): GB2021. doi:10.1029/2004GB002401.
- Zou, J., Y. Huang, Y. Qin, S. Liu, Q. Shen, G. Pan, L. Yanyu, and Q. Liu. 2009. “Changes in Fertilizer-Induced Direct N2O Emissions from Paddy Fields during Rice-Growing Season in China between 1950s and 1990s.” Global Change Biology 15 (1): 229–242. doi:10.1111/j.1365-2486.2008.01775.x.