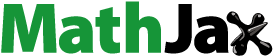
ABSTRACT
Biological nitrogen fixation (BNF) is an important nitrogen source for both N2-fixers and their neighboring plants in natural and managed ecosystems. Biological N fixation can vary considerably depending on soil conditions, yet there is a lack of knowledge on the impact of varying soils on the contribution of N from N2-fixers in mixed swards. In this study, the amount and proportion of BNF from red clover were assessed using three grassland soils. Three soil samples, Hallsworth (HH), Crediton (CN), and Halstow (HW) series, were collected from three grassland sites in Devon, UK. A pot experiment with 15N natural abundance was conducted to estimate BNF from red clover, and the proportion of N transferred from red clover to the non-N2 fixing grass in a grass-clover system. The results showed that BNF in red clover sourced from atmosphere in the HH soil was 2.92 mg N plant−1, which was significantly lower than that of the CN (6.18 mg N plant−1) and HW (8.01 mg N plant−1) soils. Nitrogen in grass sourced from BNF via belowground was 0.46 mg N plant−1 in the HH soil, which was significantly greater than that in CN and HW soils. However, proportionally there were no significant differences in the percentage N content of both red clover and grass sourced from BNF via belowground among soils, at 65%, 67%, 65% and 35%, 27%, 31% in HH, CN, and HW, respectively. Our observations indicate that the amount of BNF by red clover varies among grassland soils, as does the amount of N sourced from BNF that is transferred to neighboring plants, which is linked to biomass production. Proportionally there was no difference among soils in N sourced from BNF in both the red clover plants and transferred to neighboring plants.
1. Introduction
Biological N fixation (BNF) is an important N source for both N2-fixers and their neighboring plants in natural and managed ecosystems and can have a key role in sustainable development of agricultural production (Crews and Peoples Citation2004; Unkovich et al. Citation2008; Frankow-Lindberg and Dahlin Citation2013). Unkovich et al. (Citation2008) reported that BNF contributes 50–70 × 106 t N annually to the global agricultural N input. For the mixed grass/legume system, the contribution of BNF to grass N yield can be up to 300 kg N ha−1 year−1 (Nyfeler et al. Citation2011). However, this high contribution of BNF to the N yield of mixed swards was only achieved when soil conditions are suitable for BNF and transfer in mixed grass/legume system (Nyfeler et al. Citation2011).
The amount of BNF and transfer in mixed grass/legume system are affected by soil pH, salinity, nutrient content, temperature, moisture, mineral toxicity, and so on (Brockwell, Bottomley, and Thies Citation1995; Peoples, Ladha, and Herridge Citation1995). For example, insufficient phosphorus (P) and potassium (K) supply limits BNF through changes in relative growth among nodules, roots, shoots, and nitrogenase activity, as demonstrated in pot experiments with red clover and white clover grown in hydroponics (Hellsten and Huss-Danell Citation2000; Høgh‐Jensen Citation2003). Biological N fixation is an energetically costly process (Vitousek and Howarth Citation1991; Kambatuku, Cramer, and Ward Citation2013), and normally, plants depend on BNF if its benefits outweigh its costs (Crews Citation1999; Kambatuku, Cramer, and Ward Citation2013), for example, under soil N limited conditions (Cramer et al. Citation2007). High concentration of soil inorganic N can inhibit the growth of nodules. Kambatuku, Cramer, and Ward (Citation2013) reported a negative relationship between soil N supply and the contribution of BNF to the N input. However, it has also been reported that soil inorganic N concentration did not affect the proportion of BNF in a clover and grass mixed sward because grasses have a greater competitive ability to uptake N from soils than clover (Nyfeler et al. Citation2011; Oberson et al. Citation2013). Thus, the proportion of BNF can remain high with increasing inputs of mineral or organic N fertilizer (Nyfeler et al. Citation2011; Oberson et al. Citation2013). Lemaire, Hodgson, and Chabbi (Citation2011) also suggested that soil inorganic N content did not directly inhibit BNF under high abundance of competitive non-N2-fixers, such as grasses, in a mixed sward.
Fixed N is made available to neighboring and succeeding plants (Hauggaard-Nielsen and Jensen Citation2005; Carlsson and Huss-Danell Citation2014). Non-N2-fixers intercropped with N2-fixers have been observed to have a lower natural 15N content than when grown in pure stands, indicating a net transfer of N from the N2-fixers (which obtain N from the atmosphere which has an inherently lower proportion of N as 15N than the soil) to neighboring non-N2-fixers (Peoples et al. Citation2015). Decomposition of plant aboveground residues and belowground roots is commonly considered the important pathway of N in N2-fixers derived from BNF transfer to neighboring plants (Munroe and Isaac Citation2014) and belowground N transfer has generally been studied in the direction of N2-fixers to neighboring non-N2-fixers (Oberson et al. Citation2013; Munroe and Isaac Citation2014). Direct transfer between roots of N2-fixers and non-N2-fixers through exudation or mycorrhizal networks has also been suggested as important N pathways too (Fellbaum et al. Citation2014; Dhamala et al. Citation2018). Low-molecular-weight N compounds, such as ammonium, nitrate, and amino-acids, exuded by N2-fixer roots may be taken up by neighboring plants (Paynel and Cliquet Citation2003; Fustec et al. Citation2010; Munroe and Isaac Citation2014), provided that the plant uptake can occur before microbial uptake (Fellbaum et al. Citation2014). However, there is a lack of information about the contribution of belowground pathways of N transfer from N2-fixers to neighboring plants.
The transfer of fixed N from N2-fixers to neighboring plants is affected by many factors. Montesinos-Navarro et al. (Citation2016) reported that common mycorrhizal relationships could enhance N transfer among adult plants. Additionally, the extent of nodule turnover and the size of the root system, through their effect on the amounts of rhizodeposited N and the incidence of mycorrhizal fungi, are likely to affect N transfer (Moyer-Henry et al. Citation2006; Phillips, Fox, and Six Citation2006; Rasmussen et al. Citation2007). Dromph et al. (Citation2006) reported that root parasites induced N transfer between plants and was dependant on the density of nematodes within clover roots. Furthermore, it has been found that the amount of N transferred from N2-fixers to neighboring non-N2-fixers was dependent on the shoot biomass ratio of donor to companion species, root turnover rate and C allocation within the plants (Rasmussen et al. Citation2007).
The objective of this study was to quantify BNF and between-plant N transfer in red clover and grass systems under controlled conditions. Estimates of BNF and N transfers were made using the 15N natural abundance method. The following hypotheses were tested: (1) the relative and absolute biomass of red clover and grass will vary between grassland soils; (2) increased red clover biomass increases both the total amount of BNF and the proportion of clover-N content derived from BNF; (3) the amount and proportion of N transferred from clover to grass will vary between grassland soils due to differences in grass growth.
2. Materials and methods
2.1. Soil preparation
Grassland soils were collected in May 2017 from the North Wyke site of Rothamsted Research in southwest England (50°46′10″N, 3°54′05″W). North Wyke has an annual precipitation of 1008 mm and mean daily minimum and maximum temperatures of 6.9°C and 13.8°C (17 years mean, Carswell et al. Citation2019). Samples were taken from three sites with varying nutrient content (): Hallsworth (HH), Crediton (CN), and Halstow (HW) series (Clayden and Hollis Citation1985), where HH and HW are of similar texture (clay loam) and CN is a sandy clay loam. All sites are permanent (CN) or semi-permanent (HH and HW) grasslands and had received no fertilization for more than 3 years before sampling. Topsoil (to a depth of 15 cm) was collected from fields using a ‘W-shaped’ sampling strategy with all samples being pooled (Loick et al. Citation2016). After sampling, the soil was air-dried to ~30% H2O (w/w, dry basis), plant residue and roots were removed, and the soil sieved to <8 mm. The soil was then split into two sub-samples, the first sub-sample was used to characterize background soil properties (), and the second sub-sample was stored in the dark at 4°C prior to use for pot experiment. All soils were acidic with the highest pH (in H2O) in the HH soil, followed by CN soil and HW soil (). The lowest total carbon (TC) and nitrogen (TN) values were found in CN soil, and the highest were found in HH soil. The highest ammonia (NH4+) was found in HH soil, followed by HW and CN soils, whereas, there was no significant difference in nitrate (NO3−) among different soils (). The highest soil exchangeable P content was found in HW soil, followed by CN soil and HH soil. However, the highest soil exchangeable magnesium content was found in HH soil, followed by CN and HW soils ().
Table 1. Soil properties.
2.2. Experimental design
The experiment was conducted in June 2017 in a glasshouse at North Wyke, southwest England. For the non-N2-fixer, we used a festulolium hybrid-grass (AberNiche) and the N2-fixer was a red clover (AberClaret). For each soil, five replicate pots containing 350 g dry weight equivalent (DWE) of soil were sown with a mixture of grass and red clover. Pots were planted on 1 June with 10 grass seeds and 8 clover seeds. According to the average ratio of grass to clover in the field, five grass and three clover plants were retained after germination. Meanwhile, a second set of pots were prepared for each soil, containing 150 g DWE soil and sown with grass alone as the reference plant. For these pots, 10 grass seeds were sown, and 5 grass plants were retained after germination. The day after sowing, the pots with red clover were inoculated with a crushed nodule suspension (5 mL pot−1, crushed nodule: water ≈ 1:20, w/w), which was collected from local mature red clover.
Grass and clover litter were collected during the experiment and their dry biomass included in the yield measurement so that no aboveground plant components decomposed in the soil. Plants were harvested to 3 cm above the soil surface (shoot) 129 days after sowing. After cleaning with deionized water, harvested biomass was oven-dried at 65°C for 72 hr for dry matter determination. Soil samples were collected after harvesting for the determination of 15N abundance. The soil samples were prepared by air-drying and passing through a series of sieves down to 0.149 mm to remove as much root material as possible. After sieving, any residual root material was removed using an electrically charged organic glass bar (organic glass bar rubbed with silk textiles).
2.3. B-value determination
To calculate the proportion of N in red clover derived from BNF, the 15N abundance of clover with only atmospheric N2 as an N source should be determined (B-value). The abundance of natural 15N abundance was usually expressed in terms of δ via EquationEquation (1)(1)
(1) . This study considered only the plant shoots for BNF and the transfer of N sourced from BNF. However, isotopic fractionation of 14N and 15N can occur between the N2-fixer roots and shoots (Unkovich et al. Citation2008). Thus, we determined the shoot-derived B-value according to both Unkovich et al. (Citation2008) and Burchill et al. (Citation2014), which accounts for within-plant fractionation of N sourced from BNF. In brief, five replicate pots were filled with a sand and perlite mixture (1:1, v/v) and six red clover seeds planted per pot, with three clover plants retained after germination. The day after sowing, the seeds were inoculated with a crushed nodule suspension. The clover was watered with an N-free nutrient solution, including K2SO4, Ca(H2PO4)2, CaSO4, FeSO4, and Trace Elements (Hatch and Murray Citation1994). Nodulation status was checked after harvesting, according to Unkovich et al. (Citation2008). And the δ15N of red clover shoot was measured after harvesting.
2.4. Laboratory analyses
Soil TN and TC contents, and the 15N abundance of soil, grass and clover samples were measured using a Carlo Erba NA 2000 linked to a Sercon20/22 isotope ratio mass spectrometer (Sercon, Crewe, UK; Carlo Erba, CE Instruments, Wigan, UK). Soil pH was determined in a 1:2.5 (v/v) soil:water solution. Soils were extracted with 2 M KCl for colorimetric determination of NH4+ and NO3− (NO3− values include NO2−) and with NaHCO3 for colorimetric determination of exchangeable P (using the Skalar SANPLUS system; Skalar Analytical B.V. Breda, Netherlands). Soil exchangeable K, calcium (Ca) and magnesium (Mg) were determined in 1 M NH4NO3 (soil: solution = 1:5, w/w) extracts using ICP-OES (Perkin Elmer LAS (UK) Ltd. Beaconsfield, UK).
2.5. Data and statistical analyses
Calculations for determining BNF from N2-fixers and the transfer of N from BNF to neighboring plants are described in full by Unkovich et al. (Citation2008). In summary, the 15N abundance of plant material is calculated as δ15N using EquationEquation 1(1)
(1) , and the proportion of BNF in clover is calculated using EquationEquation 2
(2)
(2) .
where PNdfa is the proportion of N in red clover derived from BNF; δ15Nref and δ15Nclover are the δ15N of reference plant and red clover (shoots only); and B is the δ15N of red clover shoots, which derived N only from atmospheric N2. The B-value was the average from five replicates in this study.
The amount of N fixed per red clover plant is calculated using EquationEquations 3(3)
(3) :
where ANdfa is the amount of N in red clover fixed from atmospheric N2, clover N yield was calculated as dry yield multiplied by the N concentration.
The proportion of N in grass transferred from red clover (PNdfc, as %) is calculated according to EquationEquation 4(4)
(4) (Frankow-Lindberg and Dahlin Citation2013).
where δ15Nnon_fixing_pure and δ15Nnon_fixing_mixed are the δ15N in grass sown alone and mixed with red clover. The net BNF-N transferred from red clover to the shoot component of grass (ANdfc, as mg N plant−1) was calculated as PNdfc multiplied by the TN content of the grass shoot (5).
The variance of amount and proportion of δ15N in grass, the amount and proportion of BNF in clover and the amount transferred from clover to grass and proportion of N from BNF within grass were examined using one-way ANOVA (PASW Statistics 18.0). Duncan’s test was used to identify significant differences among soils, and the differences of δ15N within the same soils in the mixed clover/grass and pure grass treatments were examined by paired-samples t-test. Differences were reported as significant where p < 0.05.
3. Results
3.1. Aboveground biomass yield and source of N for clover
The aboveground biomass yield for red clover for the HH soil was significantly lower than that for the CN or HW soils (). The red clover shoot-N concentration was significantly greater for the HW soil than that observed in the HH or CN soils (). The total N in red clover fixed from atmospheric N2 was significantly lower in the HH soil (2.92 ± 0.68 mg N plant−1) than in the CN and HW soils, which were up to 6.18 ± 1.62 and 8.01 ± 1.94 mg N plant−1 ()). However, the proportion of N in red clover fixed from atmospheric N2 was not significantly different among the soils at 65%, 67%, and 65% in HH, CN, and HW, respectively ()). There was a positive relationship between the amount of fixed N in clover and the clover shoot biomass (R2 = 0.50; ).
Table 2. Nitrogen concentration and δ15N of the reference grass, and the grass and red clover grown together, after harvesting.
Figure 1. Grass and clover aboveground biomass yields for the three soils. HH, CN, and HW are the Hallsworth, Crediton, and Halstow soil series, respectively. Error bars show the standard error of the treatment mean (n = 5) and different letters represent significant differences between values within soils (p < 0.05).
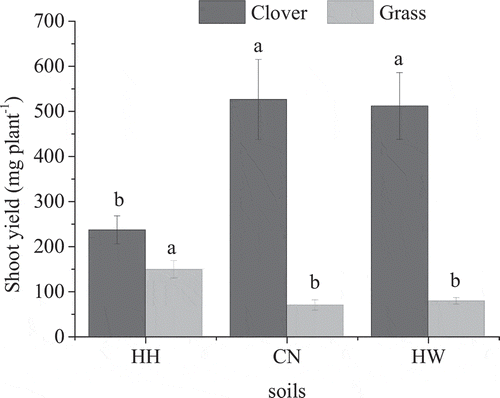
Figure 2. The amount (ANdfa; a) and proportion (PNdfa; b) of N in red clover derived from the atmosphere. HH, CN, and HW are the Hallsworth, Crediton, and Halstow soils, respectively. Error bars show the standard error of the treatment mean (n = 5) and different letters represent significant differences between values (p < 0.05).
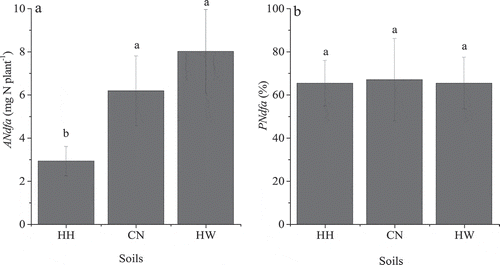
3.2. Aboveground biomass yield and source of N for grass
In contrast to the red clover yield, grass dry matter yield was significantly greater for the HH soil at 96.6 mg plant−1, than for the CN and HW soil (). The grass-N content for the HH soil was also significantly higher than that for the CN and HW soils (). The δ15N of grass planted with red clover was lower than that of the reference grass (). The ANdfc in the grass in the HH soil at 0.46 ± 0.15 mg N plant−1, was significantly greater than observed for the CN and HW soils ()). However, proportionally there was no significant difference in PNdfc across all soils, which were 35%, 27%, and 31% in HH, CN, and HW, respectively ()). The ANdfc generally increased with grass shoot biomass ().
Figure 4. The amount (ANdfc,a) and proportion (PNdfc,b) of N in grass transferred from clover. HH, CN, and HW are Hallsworth, Crediton, and Halstow soil series, respectively. Error bars show the standard error of treatment means (n = 5), different letters represent significant differences between values (p < 0.05).
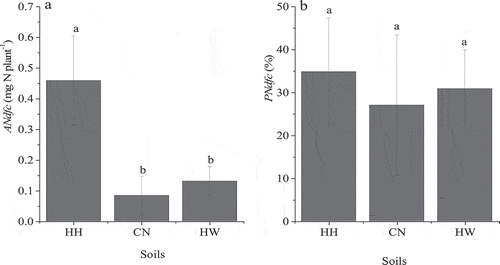
Figure 5. The relationship between the quantity of N in the grass shoot transferred from the red clover and total grass aboveground biomass.
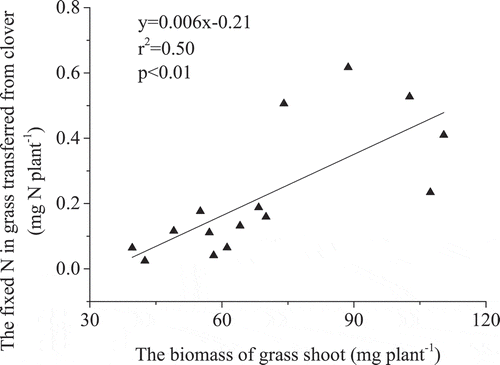
After harvesting, the δ15N of soil planted with the reference plant in HH, CN, and HW soils were 5.56‰, 6.10‰, and 4.69‰ (), respectively. The δ15N of soils generally decreased when they were planted with grass and red clover, with significantly lower values observed for the CN soil at 5.74‰.
Figure 6. The δ15N in soil planted with grass or with grass and red clover together. HH, CN, and HW are Hallsworth, Crediton, and Halstow soil series, respectively. Error bars show the standard error of the treatment mean values (n = 5), and the different letters represent significant differences between values within soil planted grass with or without clover (p < 0.05).
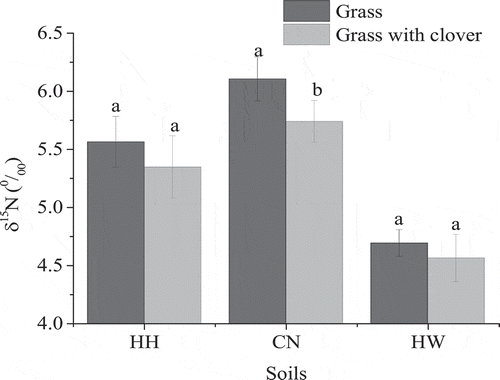
4. Discussion
4.1. The amount, but not the proportion of BNF in clover varies between grassland soils
There was greater biomass of red clover in CN and HW soils than for the HH soil (), indicating that CN and HW soils were more suitable for red clover growth than the HH soil. Additionally, there was more N fixed in red clover in the CN and HW soils than in the HH soil (), which increased with clover biomass (). This observation indicated that N2-fixer dry matter yield may be a key factor in the amount of N fixed by N2-fixers, in agreement with the report of Gierus et al. (Citation2012). The lower clover yield in HH soil may be attributed to the lower exchangeable P content of the HH soil compared with the CN and HW soils (). It has been found that clover yield can be inhibited under low P availability (Oberson et al. Citation2013; Tang et al. Citation2017). This agrees with the report of Kermah et al. (Citation2018), who suggested that growing N2-fixers in fertile fields was more lucrative due to greater stover and grain yields.
The majority of N required by clover can be provided by BNF. In a field study, Huss-Danell, Chaia, and Carlsson (Citation2007) observed that the proportion of N in clover herbage derived from BNF was often >80% across the season. In our study, the proportion of N in red clover derived from the BNF was 65–67% ()), less than in other studies (Huss-Danell, Chaia, and Carlsson Citation2007; Rasmussen et al. Citation2007; Oberson et al. Citation2013), which may be attributed to our short growing period. In contrast to the ANdfa in red clover, there was no significant influence of soil on PNdfa ()). It has been reported that, although soil N available improved via mineral N input rate over the range 6.5–150 kg N ha−1 year−1, the proportion of BNF in mixed grass-clover swards was not affected (Oberson et al. Citation2013), which is in agreement with our findings (). This phenomenon was explained due to the fact that mineral N availability remains low for clover because of the highly competitive ability of grasses to uptake inorganic N in the soil; thus, the amount of N in clover derived from the BNF maintains great if fertilizer input just moderately increasing (Nyfeler et al. Citation2011; Oberson et al. Citation2013). Additionally, Pandey et al. (Citation2017) reported that N2-fixers can maintain BNF without being significantly affected by fertilizer application or fertility building measures (e.g. crop covering, animal manure applying, straw returning) in grass-clover or grain legume systems. Our observations are also in agreement with Oberson et al. (Citation2013) who reported that PNdfa was not affected by different levels of fertilization in organically or conventionally managed grass-clover leys. Rasmussen et al. (Citation2012) also reported that the proportion of N derived from BNF in red clover was unaffected by amendment with cattle slurry N. Although, other studies have reported reductions in PNdfa where there was more available N in soil from fertilizer application (Kambatuku, Cramer, and Ward Citation2013), and in N2-fixer dominated swards (e.g. >60% legumes), the activity of N2 fixation was down-regulated by increased N availability in soil (Lemaire, Hodgson, and Chabbi Citation2011). Lemaire, Hodgson, and Chabbi (Citation2011) suggested that, because of the low abundance of the non-N2-fixers, the N demand of the whole swards was reduced and the N2-fixers were readily affected by soil available N.
4.2. The amount, but not the proportion of fixed N2 in grass transferred from clover varied across grassland soils
In contrast to the clover biomass, the grass biomass was lower in the CN and HW soils than in the HH soil (), indicating that the HH soil was more suitable for grass growth than the CN and HW soils. The low exchangeable Mg content in the CN and HW soils () may be a key limiting factor for grass biomass yields, as Mg is an important element for grass growth (Guo et al. Citation2016). Additionally, the competition between red clover and grass for the uptake of divalent cations (Ca2+, Mg2+) may be another reason leading to low grass biomass in the CN and HW soils, because of the greater biomass and capacity of clover for the uptake of divalent cations compared to grass (Frame, Charlton, and Laidlaw Citation1998). Nitrogen concentrations in grass were greatest in the HH soil (), which also contained greater soil TN and NH4+ content (), suggesting that the grass was effective in taking up the soil-N (Oberson et al. Citation2013). The δ15N in grass mixed with clover was generally lower than that of the reference grass (), indicating the transfer of fixed atmospheric N2 from the clover to the grass. However, this method only can be used to qualitatively analyze N in grass transferred from clover when the δ15N of grass decreasing is solely due to BNF of clover and not other N sources (e.g. fertilizer applied). Our observations also showed a slight decrease in δ15N in the soils that were planted with grass and clover relative to the soil containing the grass reference plant alone (), which suggests that the soil N pool might have been affected by clover-derived N. However, differences were not significant and further evidence of such an effect is required.
Intercropped plants can exchange water and nutrients along source-sink gradients (Querejeta et al. Citation2012). It has been reported that decomposition of N2-fixers aboveground litter and exudation of soluble N compounds from N2-fixers may contribute to decreased soil δ15N where clover is planted, which is considered the important pathway of fixed N transfer (Paynel et al. Citation2008; He et al. Citation2009; Munroe and Isaac Citation2014). Additionally, common mycorrhizal networks have been suggested as a potential mechanism for N transfer from N2-fixers to non-N2-fixing plants (Montesinos-Navarro et al. Citation2016). Montesinos-Navarro et al. (Citation2016) also suggest that this fixed-N transfer pathway, mediated by mycorrhizal fungi, is more effective than via root exudates from the N2-fixers in the short term. It was suggested that 46–60% of N uptake by the grass in a two-year grass-clover ley was clover fixed N (Oberson et al. Citation2013). In our study, the proportion of N in grass estimated to have derived from BNF by clover was somewhat less, at 27–35% ()). This could be attributed to the removal of plant-litter in this study, so N did not enter the soil via aboveground litter decomposition. Thus, the pathway of N transferred from red clover to grass in our study was via exudation of soluble N compounds from the plant-associated mycorrhizae, and decomposition of N2-fixer roots belowground. There was no significant difference in the proportion of fixed N in grass across the different soils ()). Our findings are in agreement with Oberson et al. (Citation2013), who reported that the proportion of N in grass derived from clover was similar in soil with different levels of available N due to fertilization. The positive correlation between the grass biomass and the fixed N in grass transferred from red clover suggests that the transfer of N in grass sourced from BNF was directly influenced by grass dry matter yield (). Similarly, Oberson et al. (Citation2013) reported that the amount of clover-derived N in grass was higher with greater grass N yields and considered that N transferred from clover to grass was related to the N accumulation in the grass.
Additionally, our observation showed that the δ15N of R-grass was much higher from the HH soil than that of CN and HW soils, although δ15N of total N from the HH soil did not higher than HW soil (). This result may be attributed to the δ15N of inorganic N in soils. Unfortunately, the δ15N of soil inorganic N was not measured in this study, which was affected not only by the δ15N of organic N, but also by N-immobilization and mineralization rates in soil. Because more energy is needed to break or form chemical bonds involving 15N than 14N, 14N-containing molecules react faster than those containing 15N (Robinson Citation2001). Thus, different N-immobilization and mineralization rates would affect the δ15N of soil inorganic N (Bustamante et al. Citation2004). To accurately predict N uptake from soil and from BNF, the relationship between the δ15N of inorganic N in soil and that of plant should be studied in the future.
5. Conclusion
The proportion of N in red clover fixed from atmospheric N2 was not differed among three grassland soils examined while, the total amount of fixed N in red clover increased with clover biomass increasing, which did vary among the soils. The amount of N in grass transferred from that fixed by the red clover varied between soils and there was a positive relationship between the aboveground biomass of the grass and the amount of fixed N the grass contained. However, there was no difference among soils in the proportion of N in grass transferred from clover. Further studies across a broader range of soil textures and inherent fertilities are needed to fully understand the correlation between BNF and soil conditions.
Disclosure Statement
No potential conflict of interest was reported by the authors.
Additional information
Funding
References
- Brockwell, J., P. J. Bottomley, and J. E. Thies. 1995. “Manipulation of Rhizobia Microflora for Improving Legume Productivity and Soil Fertility: A Critical Assessment. Management of Biological Nitrogen Fixation for the Development of More Productive and Sustainable Agricultural Systems.” Plant and Soil 174 (1–2): 143–180. doi:10.1007/BF00032245.
- Burchill, W., E. James, D. Li, G. Lanigan, M. Williams, P. Iannetta, and J. Humphreys. 2014. “Comparisons of Biological Nitrogen Fixation in Association with White Clover (Trifolium Repens L.) Under Four Fertiliser Nitrogen Inputs as Measured Using Two 15N Techniques.” Plant and Soil 385: 287–302. doi:10.1007/s11104-014-2199-1.
- Bustamante, M. M. C., L. A. Martinelli, D. A. Silva, P. B. Camargo, C. A. Klink, T. F. Domingues, and R. V. Santos. 2004. “15N Natural Abundance in Woody Plants and Soils of Central Brazilian Savannas (Cerrado).” Ecological Applications 14 (4): 200–213. doi:10.1890/01-6013.
- Carlsson, G., and K. Huss-Danell. 2014. “Does Nitrogen Transfer between Plants Confound 15N-based Quantifications of N2 Fixation?” Plant and Soil 374: 345–358. doi:10.1007/s11104-013-1802-1.
- Carswell, A. M., K. Gongadze, T. H. Misselbrook, and L. Wu. 2019. “Impact of Transition from Permanent Pasture to New Swards on the Nitrogen Use Efficiency, Nitrogen and Carbon Budgets of Beef and Sheep Production.” Agriculture Ecosystems & Environment. doi:10.1016/j.agee.2019.106572.
- Clayden, B., and J. M. Hollis. 1985. Criteria for Differentiating Soil Series. Harpenden, UK: Rothamsted Experimental Station Press.
- Cramer, M. D., S. B. Chimphango, A. Van Cauter, M. Waldram, and W. Bond. 2007. “Grass Competition Induces N2 Fixation in Some Species of African.” Journal of Ecology 95: 1123–1133. doi:10.1111/j.1365-2745.2007.01285.x.
- Crews, T., and M. Peoples. 2004. “Legume versus Fertilizer Sources of Nitrogen: Ecological Tradeoffs and Human Needs.” Agriculture Ecosystems & Environment 102: 279–297.
- Crews, T. E. 1999. “The Presence of Nitrogen Fixing Legumes in Terrestrial Communities: Evolutionary Vs Ecological Considerations. New Perspectives on Nitrogen Cycling in the Temperate and Tropical Americas.” Biogeochemistry 46: 233–246. doi:10.1007/978-94-011-4645-6_11.
- Dhamala, N. R., J. Rasmussen, G. Carlsson, K. Søegaard, and J. Eriksen. 2018. “Effects of Including Forbs on N2-fixation and N Yield in Red Clover-ryegrass Mixtures.” Plant and Soil 424: 525–537. doi:10.1007/s11104-017-3554-9.
- Dromph, K. M., R. Cook, N. J. Ostle, and R. D. Bardgett. 2006. “Root Parasite Induced Nitrogen Transfer between Plants Is Density Dependent.” Soil Biology & Biochemistry 38: 2495–2498. doi:10.1016/j.soilbio.2006.02.005.
- Fellbaum, C. R., J. A. Mensah, A. J. Cloos, G. E. Strahan, P. E. Pfeffer, E. T. Kiers, and H. Bücking. 2014. “Fungal Nutrient Allocation in Common Mycorrhizal Networks Is Regulated by the Carbon Source Strength of Individual Host Plants.” New Phytologist 203: 646–656. doi:10.1111/nph.12827.
- Frame, J., J. Charlton, and A. S. Laidlaw. 1998. Temperate Forage Legumes. Wallingford, UK: Cab International Press.
- Frankow-Lindberg, B., and A. Dahlin. 2013. “N2 Fixation, N Transfer, and Yield in Grassland Communities Including a Deep-rooted Legume or Non-legume Species.” Plant and Soil 370: 567–581. doi:10.1007/s11104-013-1650-z.
- Fustec, J., F. Lesuffleur, S. Mahieu, and J. B. Cliquet. 2010. “Nitrogen Rhizodeposition of Legumes. A Review.” Agronomy for Sustainable Development 30: 57–66. doi:10.1051/agro/2009003.
- Gierus, M., J. Kleen, R. Loges, and F. Taube. 2012. “Forage Legume Species Determine the Nutritional Quality of Binary Mixtures with Perennial Ryegrass in the First Production Year.” Animal Feed Science and Technology 172: 150–161. doi:10.1016/j.anifeedsci.2011.12.026.
- Guo, W., H. Nazim, Z. Liang, and D. Yang. 2016. “Magnesium Deficiency in Plants: An Urgent Problem.” The Crop Journal 4: 83–91. doi:10.1016/j.cj.2015.11.003.
- Hatch, D. J., and P. J. Murray. 1994. “Transfer of Nitrogen from Damaged Roots of White Clover (Trifolium Repens L.) To Closely Associated Roots of Intact Perennial Ryegrass (Lolium Perenne L).” Plant and Soil 166 (2): 181–185. doi:10.1007/BF00008331.
- Hauggaard-Nielsen, H., and E. S. Jensen. 2005. “Facilitative Root Interactions in Intercrops. Root Physiology: From Gene to Function.” Plant and Soil 274: 237–250. doi:10.1007/s11104-004-1305-1.
- He, X., M. Xu, G. Y. Qiu, and J. Zhou. 2009. “Use of 15N Stable Isotope to Quantify Nitrogen Transfer between Mycorrhizal Plants.” Journal of Plant Ecology 2: 107–118. doi:10.1093/jpe/rtp015.
- Hellsten, A., and K. Huss-Danell. 2000. “Interaction Effects of Nitrogen and Phosphorus on Nodulation in Red Clover (Trifolium Pratense L.).” Acta Agriculturae Scandinavica, Section B-Plant Soil Science 50: 135–142. doi:10.1080/090647100750374287.
- Høgh‐Jensen, H. 2003. “The Effect of Potassium Deficiency on Growth and N2‐fixation in Trifolium Repens.” Physiologia Plantarum 119: 440–449. doi:10.1034/j.1399-3054.2003.00189.x.
- Huss-Danell, K., E. Chaia, and G. Carlsson. 2007. “N2 Fixation and Nitrogen Allocation to above and below Ground Plant Parts in Red Clover-grasslands.” Plant and Soil 299: 215–226. doi:10.1007/s11104-007-9376-4.
- Kambatuku, J. R., M. D. Cramer, and D. Ward. 2013. “Nitrogen Fertilisation Reduces Grass-induced N2 Fixation of Tree Seedlings from Semi-arid Savannas.” Plant and Soil 365: 307–320. doi:10.1007/s11104-012-1389-y.
- Kermah, M., A. Franke, S. Adjei-Nsiah, B. Ahiabor, R. C. Abaidoo, and K. Giller. 2018. “N2-fixation and N Contribution by Grain Legumes under Different Soil Fertility Status and Cropping Systems in the Guinea Savanna of Northern Ghana.” Agriculture Ecosystems & Environment 261: 201–210. doi:10.1016/j.agee.2017.08.028.
- Lemaire, G., J. Hodgson, and A. Chabbi. 2011. Grassland Productivity and Ecosystem Services. Cambridge, MA: Cab Internatiional Press.
- Loick, N., E. R. Dixon, D. Abalos, A. Vallejo, G. Peter Matthews, K. L. McGeough, R. Well, et al. 2016. “Denitrification as a Source of Nitric Oxide Emissions from Incubated Soil Cores from a UK Grassland Soil.” Soil Biology & Biochemistry 95:1–7. doi:10.1016/j.soilbio.2015.12.009.
- Montesinos-Navarro, A., M. Verdú, J. I. Querejeta, L. Sortibrán, and A. Valiente-Banuet. 2016. “Soil Fungi Promote Nitrogen Transfer among Plants Involved in Long-lasting Facilitative Interactions.” Perspectives in Plant Ecology, Evolution and Systematics 18: 45–51. doi:10.1016/j.ppees.2016.01.004.
- Moyer-Henry, K., J. Burton, D. Israel, and T. Rufty. 2006. “Nitrogen Transfer between Plants: A 15N Natural Abundance Study with Crop and Weed Species.” Plant and Soil 282: 7–20. doi:10.1007/s11104-005-3081-y.
- Munroe, J. W., and M. E. Isaac. 2014. “N2-fixing Trees and the Transfer of fixed-N for Sustainable Agroforestry: A Review.” Agronomy for Sustainable Development 34: 417–427. doi:10.1007/s13593-013-0190-5.
- Nyfeler, D., O. Huguenin-Elie, M. Suter, E. Frossard, and A. Lüscher. 2011. “Grass–legume Mixtures Can Yield More Nitrogen than Legume Pure Stands Due to Mutual Stimulation of Nitrogen Uptake from Symbiotic and Non-symbiotic Sources.” Agriculture Ecosystems & Environment 140: 155–163. doi:10.1016/j.agee.2010.11.022.
- Oberson, A., E. Frossard, C. Buehlmann, J. Mayer, P. Maeder, and A. Luescher. 2013. “Nitrogen Fixation and Transfer in Grass-clover Leys under Organic and Conventional Cropping Systems.” Plant and Soil 371: 237–255. doi:10.1007/s11104-013-1666-4.
- Pandey, A., F. Li, M. Askegaard, and J. E. Olesen. 2017. “Biological Nitrogen Fixation in Three Long-term Organic and Conventional Arable Crop Rotation Experiments in Denmark.” European Journal of Agronomy 90: 87–95. doi:10.1016/j.eja.2017.07.009.
- Paynel, F., and J. Cliquet. 2003. “N Transfer from White Clover to Perennial Ryegrass, via Exudation of Nitrogenous Compounds.” Agronomie 23: 503–510. doi:10.1051/agro:2003022.
- Paynel, F., F. Lesuffleur, J. Bigot, S. Diquélou, and J. B. Cliquet. 2008. “A Study of 15N Transfer between Legumes and Grasses.” Agronomy for Sustainable Development 28: 281–290. doi:10.1051/agro:2007061.
- Peoples, M., J. Ladha, and D. Herridge. 1995. “Enhancing Legume N2 Fixation through Plant and Soil Management. Management of Biological Nitrogen Fixation for the Development of More Productive and Sustainable Agricultural Systems.” Plant and Soil 174: 83–101. doi:10.1007/978-94-011-0055-7_4.
- Peoples, M. B., P. M. Chalk, M. J. Unkovich, and R. M. Boddey. 2015. “Can Differences in 15N Natural Abundance Be Used to Quantify the Transfer of Nitrogen from Legumes to Neighbouring Non-legume Plant Species?” Soil Biology & Biochemistry 87: 97–109. doi:10.1016/j.soilbio.2015.04.010.
- Phillips, D. A., T. C. Fox, and J. Six. 2006. “Root Exudation (Net Efflux of Amino Acids) May Increase Rhizodeposition under Elevated CO2.” Global Change Biology 12: 561–567. doi:10.1111/j.1365-2486.2006.01100.x.
- Querejeta, J. I., L. M. Egerton-Warburton, I. Prieto, R. Vargas, and M. F. Allen. 2012. “Changes in Soil Hyphal Abundance and Viability Can Alter the Patterns of Hydraulic Redistribution by Plant Roots.” Plant and Soil 355: 63–73. doi:10.1007/s11104-011-1080-8.
- Rasmussen, J., J. Eriksen, E. S. Jensen, K. H. Esbensen, and H. Høgh-Jensen. 2007. “In Situ Carbon and Nitrogen Dynamics in Ryegrass–clover Mixtures: Transfers, Deposition and Leaching.” Soil Biology & Biochemistry 39: 804–815. doi:10.1016/j.soilbio.2006.10.004.
- Rasmussen, J., K. Søegaard, K. Pirhofer-Walzl, and J. Eriksen. 2012. “N2-fixation and Residual N Effect of Four Legume Species and Four Companion Grass Species.” European Journal of Agronomy 36: 66–74. doi:10.1016/j.eja.2011.09.003.
- Robinson, D. 2001. “δ15N as an Integrator of the Nitrogen Cycle.” Trends in Ecology & Evolution 16 (3): 153–162. doi:10.1016/S0169-5347(00)02098-X.
- Tang, Y., M. Zhang, A. Chen, W. Zhang, W. Wei, and R. Sheng. 2017. “Impact of Fertilization Regimes on Diazotroph Community Compositions and N2-fixation Activity in Paddy Soil.” Agriculture Ecosystems & Environment 247: 1–8. doi:10.1016/j.agee.2017.06.009.
- Unkovich, M., D. Herridge, M. Peoples, G. Cadisch, B. Boddey, K. Giller, B. Alves, and P. Chalk. 2008. Measuring Plant-associated Nitrogen Fixation in Agricultural Systems. Canberra: ACIAR Press.
- Vitousek, P. M., and R. W. Howarth. 1991. “Nitrogen Limitation on Land and in the Sea: How Can It Occur?” Biogeochemistry 13: 87–115. doi:10.1007/BF00002772.