ABSTRACT
The stability of black soil carbon in the deep layers of Japanese volcanic ash soil (i.e., buried A horizons) is often explained by its unique chemical (molecular structure) and physical (associated with short-range-order minerals) recalcitrance. However, the stability of black soil C in buried A horizons may be changed by labile C supply for soil microbes. Here, we hypothesized that the mineralization of black soil C in buried A horizons of Japanese volcanic ash soil could be easily accelerated by a supply of labile C (i.e., a priming effect; PE). To test our hypothesis, we investigated the direction and magnitude of the PE with a buried A horizon in Japan using 13C-labeled glucose (2.188 atom %) in a short-term (21 days) incubation study. We also investigated the effect of mineral nitrogen (N), which could contribute to microbial activity in this incubation study. We found that a positive PE occurred by glucose supply with (182%) or without (181%) mineral N input over the 21-day incubation, and its values were very similar to the PE ratios previously reported in other deep soils. The estimated mean residence time (MRT) of black soil C considering PE was clearly accelerated by glucose supply, regardless of mineral N input, compared with the initial soil MRT. These results strongly support our hypothesis that the mineralization rate of black soil C in buried A horizons is easily accelerated by a labile C supply, and it also demonstrates important implications for the effects of global warming on buried A horizons (e.g., increased root exudation, fine root biomass supply, and N deposition) in Japanese volcanic ash soils.
1. Introduction
It is well known that black soil carbon (C), particularly black humic acids in the deep layers (below 20–100 cm) of Japanese volcanic ash soils (i.e., buried A horizons), shows its unique chemical properties which are characterized by their high condensed aromatic C content with a large C-layer plane size and a high degree of darkness (i.e., A600/C), such as black carbon (Shindo and Honma Citation2001; Watanabe and Takeda Citation2006; Watanabe and Fujitake Citation2008; Ikeya et al. Citation2011). These black soil C associated with short-range-order minerals (particularly allophane/imogolite) and/or monomeric Al and Fe ions (i.e., active Al and Fe) form unique aggregate hierarchy, which is physically very stable (Wada and Higashi Citation1976; Inoue Citation1990; Asano and Wagai Citation2014). These chemical and physical recalcitrant properties of black soil C result in the unavailability of C to soil microbes, which is often attributed to its strong stability in situ (Wada and Higashi Citation1976; Inoue Citation1990; Shindo and Honma Citation2001; Watanabe and Takeda Citation2006).
A new theory has recently been proposed that the stability of soil C is attributable to a lack of labile C for soil microbes. This phenomenon is known as the priming effect (PE), referring to a change in the mineralization of native recalcitrant soil C due to the addition of new labile C substrates, and has been observed in many laboratory and field studies (e.g., Kuzyakov, Friedel, and Stahr Citation2000; Fontaine et al. Citation2004, Citation2007, Citation2011; Chen et al. Citation2014). For example, Fontaine et al. (Citation2007) found that cellulose input primed microbial activity, leading to faster decomposition of older recalcitrant soil C in the deep layers of grassland soil (60–80 cm). Wang et al. (Citation2014) also reported similar results in forest soil by litter supply. These results are remarkable as new findings of soil C stabilization theory, and this theory probably applies to the stability of black soil C in buried A horizons of Japanese volcanic ash soil with rare black soil C characteristics.
Additionally, it is generally considered that land use change (e.g., reforestation) and/or global warming increases labile C input (e.g., root exudation and fine root biomass) to the soil, especially deep layers of soil, through increased photosynthesis and C allocation below ground (Jobbágy and Jackson, Citation2000; Lal, Citation2004; Wang et al. Citation2014; Karhu et al. Citation2016). Such a labile C input in the deep layers of soil through deepening of the root profile can break aggregates and decrease bulk density, increasing soil oxygen diffusion rates (Mueller, Jensen, and Megonigal Citation2015; Bernal et al. Citation2016). There is also a concern that an increase in N deposition (e.g., NH4+ and NO3−) to terrestrial ecosystems will accompany application of mineral N fertilizer and/or global warming (Galloway et al. Citation2004). Therefore, many researchers are focusing on the PE phenomenon and the effect of N deposition on the PE, especially in deep layers of soils.
We hypothesized that mineralization rate of black soil C in the deep layers of Japanese volcanic ash soil could be easily accelerated by a labile C supply with more aerobic condition despite its strong chemical recalcitrance and physical resistance. To test our hypothesis, we conducted a short-term (21 days) laboratory incubation study to investigate the direction and magnitude of PE in deep black soil C (below 40–50 cm) in Japanese volcanic ash soil with and without a supply of glucose (the most often released sugar in rhizodeposits; Derrien, Marol, and Balesdent Citation2004). We also investigated the relationships between PE and mineral nitrogen (N) application.
2. Materials and Methods
2.1. Soil sample
A soil sample was taken from the campus of the Takayama Experimental Forest (Takayama site), River Basin Research Center, Gifu University, Japan (36° 08′ N, 137° 25′ E, 1425 m above sea level). A detailed description of this site is provided elsewhere (Ohtsuka et al. Citation2005; Chen et al. Citation2017; Iimura et al. Citation2019). The soil at this study site was characterized as Andosols (Iimura et al. Citation2019). We collected our sample from the buried A horizon (40–50 cm). After collection, the fresh soil samples were passed through a 2-mm mesh sieve, which was followed by removal of almost all visible plant debris and roots using tweezers. Then, the fresh soil samples were immediately stored in a refrigerator at 5°C for approximately 10 days until use in the incubation experiment (see Section 2.3). Subsamples for soil characterization were air-dried and subjected to the same process of sieving and removal of roots as described above.
2.2. Soil analysis
Soil pH was measured using a solution of the air-dried soil (H2O or 1 M KCl) at a ratio of 1:5 after shaking for 1 h. To measure the pH of NaF, 1 g of air-dried soil was mixed with 50 ml of 1 M NaF (pH 7.0), the suspension was stirred continuously for 2 min, and the pH of the suspension was measured. The C and N contents of the soil were measured with an NC analyzer (Sumigraph NC-22 F, Sumika Chemical Analysis Service, Japan). Microbial biomass C was determined by the ATP method (Aoyama Citation2005). The melanic index of soil humus (MI) was measured using the methods of Yamamoto, Honna, and Utsumi (Citation2000). The δ13C value of the soil sample was measured by an elemental analysis/stable isotopic ratio mass spectrometer (EA/IRMS) continuous flow system (Thermo Scientific, USA). The δ13C measurements were repeated until the standard deviation reached <0.2‰. The 14C content of soil was determined by accelerator mass spectrometry (AMS) at the National Institute for Environmental Studies. Soil samples were first converted to CO2 and purified cryogenically. The purified CO2 was then reduced to graphite with H2 over Fe and its 14C/12C ratio was measured. The 14C activities are determined with respect to the international standard of oxalic acid. Final 14C activities are reported in Δ14C. The Δ14C is defined as the deviation in parts per thousand from the modern standard (Stuiver and Polach Citation1977). Ages were calculated from the Δ14C values using the conventional 14C half-life.
2.3. Incubation experiment
The experimental units consisted of fresh sieved soil (50 g of oven-dried samples) placed in 500-ml jars and incubated at 25°C for 21 days until the CO2 emission from the soil slowed down. After 10 days of preincubation, glucose was added to the soil (ca. 1 g C-glucose per kg dry soil was prepared according to Fontaine et al. Citation2007) and mixed. Mineral N ((NH4)2SO4) was also applied to the soil at N rate of 50 mg NH4+-N kg−1, which was based on the predicted amount of mineral N (NH4+, NO3−) deposition to terrestrial ecosystems in the future (Galloway et al. Citation2004). Soil without glucose and mineral N was prepared as a control and was mixed to provide the same physical disturbance. The incubation experiment included four treatments (three replicates): soil only (S), soil with mineral N (SN), soil with glucose (SC), and soil with glucose plus mineral N (SCN). The moisture content of the soil was adjusted to 60% of the water-holding capacity with distilled water during incubation. The released CO2 was trapped in a NaOH solution at 2, 4, 7, 14, and 21 days of incubation until CO2 emission from the soil slowed down. A glass vial containing 20 ml of 0.2 M NaOH solution was placed in each jar to trap the CO2 emitted from the soil, and the jars were sealed. The jars were flushed with CO2-free air each time the NaOH solution was replaced. The C content of the NaOH solution was measured by titration (Wang et al. Citation2014). δ13C of CO2 was analyzed by an EA/IRMS continuous flow system after precipitation of the carbonates with excess BaCl2 and filtration (Fontaine et al. Citation2011) every 7 days of incubation. The δ13 C measurements were repeated until the standard deviation reached <0.2‰.
2.4. Calculation of priming effect (PE)
The substrates (glucose) added to the soil allowed the separation of the respiration rates (mg CO2-C kg−1 soil) of native soil C (Rsoil) and of substrates (Rsub) using mass balance equations (Fontaine et al. Citation2011) as follows:
Rsoil + Rsub=Rtotal
Rsoil × δ13C soil + Rsub × δ13 C sub=Rtotal × δ13C total
where δ13C soil is the δ13C of soil C, δ13C sub is the δ13C of glucose C, Rtotal is the total CO2 emitted by soil with glucose, and δ13C total is its δ13C.
The PE (mg C-CO2 kg−1 soil) induced by the addition of substrates was calculated as:
PE=(Rsoil soil with substrates) – (Rsoil control soil)
where (Rsoil control soil) is the CO2 emitted by the control soil.
2.5. Statistical analysis
For total CO2 emission, soil-derived CO2 emission, and PE among treatments in each incubation period, a one-way analysis of variance (ANOVA) was performed with StatPlus:mac Pro (version 5.9.92).
3. Results and discussion
In this study, we focused on the stability of black soil C in buried A horizon in a Japanese volcanic ash soil that is usually considered very stable in situ (Watanabe and Takeda Citation2006; Fujitake Citation2011), and this is the first study to quantify the effect of labile C addition on black soil C mineralization in the deep layers of Japanese volcanic ash soil. The soil is characterized as Andosols (FAO et al., Citation2014). It is weakly acidic, with a pH (NaF) of 9.5 or more, an MI of <1.7, and a high C content (). These results are consistent with the findings of a previous study (Chen et al. Citation2017; Iimura et al. Citation2019), which classified the soil at the Takayama site as an andic Japanese volcanic ash soil (Kuroboku). Additionally, the 14C age of this soil was calculated as 4764 to 5082 years before present (YrBP) (). From these results, we confirmed that the soil used in this study satisfied our study aims.
Table 1. Characteristics of the soil used in this study.
The cumulative total CO2 emission from each treatment in the 21-day incubation experiment is shown in . Analysis by one-way ANOVA revealed that the total CO2 emission in each incubation period was significantly different among treatments. Glucose application (SC and SCN), regardless of mineral N input, stimulated total CO2 emission within a week and then gradually increased with incubation. In the soil with mineral N (SN), there was a gradual decrease in CO2 emission compared with the control soil (S) during the 21-day incubation period; this result is in accordance with a meta-analysis where N deposition impedes organic C decomposition in temperate forest soils (Janssens et al. Citation2010). Chen et al. (Citation2014), in studies of surface sandy loam soil, reported that total CO2 emission significantly increased with sucrose (sugar) application, regardless of mineral N (NH4+) input, but that the CO2 emission from soil with mineral N showed a slightly lower trend than that of the control soil during 9 days of incubation, which was similar to our results. The reduction of CO2 emission from soil with N deposition is often explained by N availability for microbes (Moorhead and Sinsabaugh Citation2006; Janssens et al. Citation2010) i.e., an increase in N availability with N deposition reduces microbial activity in mining for soil organic matter (SOM) and our result in SN strongly supported this theory.
Figure 1. Cumulative total CO2 emissions from each treatment. S, soil only (control); SN, soil with mineral N; SC, soil with glucose; SCN, soil with glucose plus mineral N. Bars indicate the standard error of the mean (n = 3). Asterisks (***) indicate significant differences at P < 0.001 by a one-way analysis of variance (ANOVA).
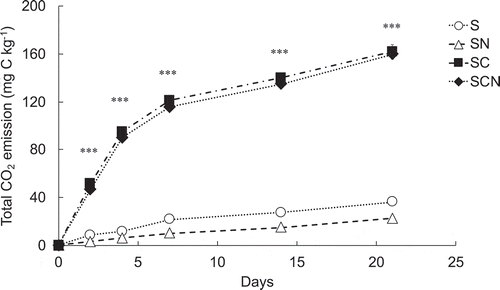
We calculated the PE among each treatment of either glucose and/or mineral N during the incubation period (–c). One-way ANOVA revealed that the PE in each incubation period was significantly different among treatments. The PE for SC and SCN increased at a constant rate every week with the addition of glucose. The addition of glucose to soil, regardless of mineral N application, showed a significant positive effect on PE during the 21-day incubation period, resulting in an PE of +18.1 (183%) to +29.4 (181%) mg C kg−1 for SC and +16.6 (176%) to 29.8 (182%) mg C kg−1 for SCN (–c). On the other hand, the PE for SN was −11.5 (47%) to −13.5 (63%) mg C kg−1 (–c). There was no significant difference in the amount of glucose degradation for SC (81.4–96.3 mg C kg−1) and SCN (77.5–93.7 mg C kg−1). Fontaine et al. (Citation2007) reported that cellulose addition to the deep soil layer in French (Cambisols) soil caused a positive PE (ca. 172%) for 161 days of an incubation study. This corresponds well to our results, despite the different soil types, incubation periods, and substrates added. It also corresponds well to the results of Wang et al. (Citation2014), who reported changes in CO2 emission from Chinese forest deep soil (60–70 cm) with leaf litter (M. macclurei) application by 167–192% higher than that of the control for 129 days of an incubation study. Karhu et al. (Citation2016) also reported that glucose addition to deep soil (32–47 cm) in a boreal forest caused a positive PE (ca. 130%) for 11 days of an incubation period. These results suggest that the reaction of black soil to the addition of glucose is not a rare case phenomenon, and it further suggests that glucose addition causes a positive PE on deep soils in a shorter period of time than higher molecule compounds, such as polysaccharide and plant litter.
Figure 2. Cumulative soil-derived CO2 (gray), glucose-derived CO2 (blank), and PEs (data above the bars) in each treatment. (a) days 7, (b) days 14, and (c) days 21. S, soil only (control); SN, soil with mineral N; SC, soil with glucose; SCN, soil with glucose plus mineral N. The number in parentheses (%) indicates the relative magnitude of PE. Bars indicate the standard error of the mean (n = 3). Values with different letters are significantly different (P < 0.05) by Tukey’s HSD post hoc test. Capital letters indicate glucose-derived CO2, and small letters indicate soil-derived CO2 and the PEs.
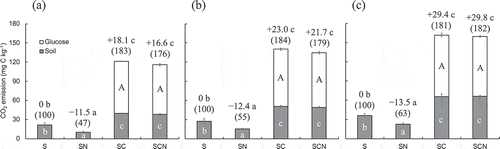
The PE can be separated into real effects (SOM decomposition) and apparent effects (changes in microbial biomass turnover excluding SOM decomposition) (Blagodatskaya and Kuzyakov Citation2008). Unfortunately, the experimental design does not distinguish between real and apparent PE according to differences in 13C signatures. The microbial turnover time usually ranged between 21 and 75 days (Blagodatskaya et al. Citation2009) and was approximately 30 days as determined by the natural abundance approach (Blagodatskaya et al. Citation2011). Considering the shorter monitoring period of the PE in this study (21 days) compared with microbial turnover, it is likely that only a small fraction of primed C comes from the microbial biomass turnover (i.e., apparent PE). In other words, most of the primed CO2 is considered to be real PE.
PE mechanism is often discussed based on ‘microbial N, P mining’ theory that some microbes (especially slow-growing K-strategists) use labile C as energy source to mineralize recalcitrant SOM to acquire nutrient, such as N and phosphorus (P) (Fontaine et al. Citation2004; Chen et al. Citation2014; Wang et al. Citation2014). Based on this theory, microbial activity, particularly slow-growing K-strategist that benefit by utilizing recalcitrant SOM, is relatively lower in SN but is higher in SC and SCN than in control soil, resulting in the possible control in PE. shows the microbial biomass at the end of the incubation period for each treatment. Although one-way ANOVA did not reveal any significant differences among treatment and we did not focused only the domination of K-strategists in the microbial biomass community, the microbial biomass tended to increase (particularly SC and SCN) when glucose was added to black soil. Furthermore, microbial biomass C showed significant positive correlations (r = 0.742, P < 0.01, n = 12) with cumulative soil-derived CO2 emission during the 21-day incubation period for each treatment (). These results support the ‘microbial N, P mining’ theory and it suggests that K-strategists are more abundant in SC and SCN than in SN to acquire N and P as nutrient source.
Figure 3. Microbial biomass in each treatment. S, soil only (control); SN, soil with mineral N; SC, soil with glucose; SCN, soil with glucose plus mineral N. Bars indicate the standard error of the mean (n = 3).
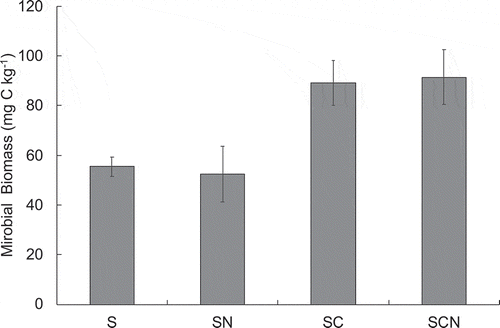
Figure 4. Linear correlation coefficients (Pearson’s correlation coefficient) between microbial biomass C and cumulative soil-derived CO2 emission during the 21-day incubation period for each treatment (n = 12). Asterisks(**) indicates significance level at P < 0.01.
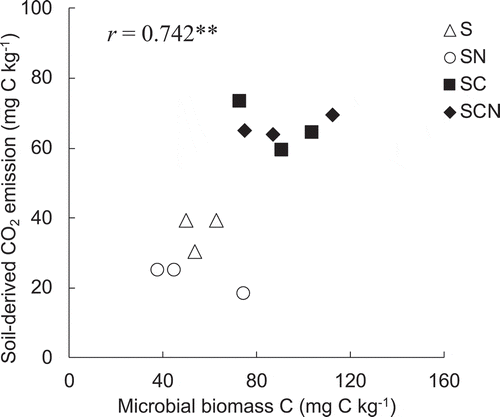
Fontaine et al. (Citation2007) calculated the mean residence time (MRT) of soil C in deep soil considering PE as 324 yr, which was much accelerated compared with the initial soil MRT (2560 yr). If the addition of a glucose supply and the resulting PE were repeated each year in the deep black soil C used in this study, we calculated that the MRT of SC and SCN according to Fontaine et al. (Citation2007) would be 89400/29.4 or 29.8 = 3038 or 2999 yr, respectively, and it was clearly accelerated compared with the initial soil MRT (5960 yr) calculated from soil C stock (89400 mg C kg−1) and annual dissolved organic C (DOC) input into buried A horizon at Takayama site (15.0 mg C kg−1 yr−1). The amount of glucose added to the soil in this study was approximately 1/10 of the annual DOC input into the surface mineral soil through the litter layer at the study site (Chen et al. Citation2017). If this level of glucose continues to be supplied to buried A horizon in the future at Takayama site, its MRT may further accelerate. Therefore, more detailed studies of the PE with labile C input (e.g., root exudation and fine root biomass), including the long-term PE reaction, on deep black soil C in Japanese volcanic ash soils are necessary for the future.
Acknowledgments
We thank the members of Laboratory of Soil Science, The University of Shiga Prefecture. The study was supported by JSPS KAKENHI Grant Number 15K16118 and 17K00524.
Disclosure statement
No potential conflict of interest was reported by the authors.
Additional information
Funding
References
- Aoyama, M. 2005. “Estimation of Microbial Biomass in Ando Soils by measurement using a Handy Iuminometer.” Japanese Society of Microbiology 59: 41–44.
- Asano, M., and R. Wagai. 2014. “Evidence of Aggregate Hierarchy at Micro- to Submicron Scales in an Allophanic Andisol.” Geoderma 216: 62–74. doi:10.1016/j.geoderma.2013.10.005.
- Bernal, B., D. C. Mckinley, B. A. Hungate, P. M. White, T. J. Mozdzer, and J. P. Megonigal. 2016. “Limits to Soil Carbon Stability; Deep, Ancient Soil Carbon Decomposition stimulated by New Labile Organic Inputs.” Soil Biology and Biochemistry 98: 85–94. doi:10.1016/j.soilbio.2016.04.007.
- Blagodatskaya, E., T. Yuyukina, S. Blagodatsky, and Y. Kuzyakov. 2011. “Turnover of Soil Organic Matter and of Microbial Biomass under C3-C4 vegetation change: consideration of 13C Fractionation and Preferential Substrate Utilization.” Soil Biology and Biochemistry 43 (1): 159–166. doi:10.1016/j.soilbio.2010.09.028.
- Blagodatskaya, E., and Y. Kuzyakov. 2008. “Mechanisms of real and apparent Priming effects and their dependence on Soil Microbial Biomass and Community Structure: Critical Review.” Biology and Fertility of Soils 45 (2): 115–131. doi:10.1007/s00374-008-0334-y.
- Blagodatskaya, E. V., S. Blagodatsky, T. H. Anderson, and Y. Kuzyakov. 2009. “Contrasting effects of Glucose, Living Roots and Maize Straw on Microbial Growth Kinetics and Substrate availability in Soil.” European Journal of Soil Science 60 (2): 186–197. doi:10.1111/j.1365-2389.2008.01103.x.
- Chen, R., M. Senbayram, S. Blagodatsky, O. Myachina, K. Dittert, X. Lin, E. Blagodatskaya, and Y. Kuzyakov. 2014. “Soil C and N availability determine the Priming effect: Microbial N Mining and Stoichiometric Decomposition Theories.” Global Change Biology 20 (7): 2356–2367. doi:10.1111/gcb.12475.
- Chen, S., S. Yoshitake, Y. Iimura, C. Asai, and T. Ohtsuka. 2017. “Dissolved Organic Carbon (DOC) Input to the Soil: DOC Fluxed and their Partitions during the growing season in a Cool-temperate Broad-leaved Deciduous Forest, Central Japan.” Ecological Research 32 (5): 724–731. doi:10.1007/s11284-017-1488-6.
- Derrien, D., C. Marol, and J. Balesdent. 2004. “The Dynamics of Neutral Sugars in the Rhizosphere of Wheat. An Approach by 13C Pulse-labelling and GC/C/IRMS.” Plant and Soil 267 (1–2): 243–253. doi:10.1007/s11104-005-5348-8.
- FAO, ISRIC, ISSS. 2014. “World Reference Base for Soli Resources.” 106 World soil resources reports. FAO, Rome.
- Fontaine, S., C. Henault, A. Aamor, N. Bdioui, J. M. G. Bloor, V. Maire, B. Mary, S. Revaillot, and P. A. Maron. 2011. “Fungi mediate long term sequestration of Carbon and Nitrogen in Soil through their Priming Effect.” Soil Biology and Biochemistry 43 (1): 86–96. doi:10.1016/j.soilbio.2010.09.017.
- Fontaine, S., G. Bardoux, L. Abbadie, and A. Mariotti. 2004. “Carbon Input to Soil may decrease Soil Carbon content.” Ecology Letters 7 (4): 314–320. doi:10.1111/j.1461-0248.2004.00579.x.
- Fontaine, S., S. Barot, P. Barre, M. Bidioui, B. Mary, and C. Rumpel. 2007. “Stability of Organic Carbon in Deep Soil layers controlled by Fresh Carbon supply.” Nature 450 (7167): 277–280. doi:10.1038/nature06275.
- Fujitake, N. 2011. “Humus Accumulation and Underlying Mechanisms in Andosols.” Japanese Society of Pedology 55: 93–98.
- Galloway, J. N., F. J. Dentener, D. G. Capone, E. W. Boyer, R. W. Howarth, S. P. Seitzinger, G. P. Asner, et al. 2004. “Nitrogen Cycles: Past, Present, and Future.” Biogeochemistry 70 (2): 153–226. doi:10.1007/s10533-004-0370-0.
- Iimura, Y., I. Tabara, K. Izumitsu, and N. Fujitake. 2019. “Priming Effect of the Addition of Maize to a Japanese Volcanic Ash Soil and Its Temperature Sensitivity: A Short-term Incubation Study.” Soil Science and Plant Nutrition 65 (5): 444–450. doi:10.1080/00380768.2019.1665969.
- Ikeya, K., T. Hikage, S. Arai, and A. Watanabe. 2011. “Size Distribution of Condensed Aromatic Rings in Various Soil Humic Acids.” Organic Geochemistry 42 (1): 55–61. doi:10.1016/j.orggeochem.2010.10.006.
- Inoue, K. 1990. Active Aluminium and Iron Components in Andisols and Related Soils.” Transactions of 14th International Congress of Soil Science, Vol. VII, 153–158. Kyoto: International Society of Soil Science.
- Janssens, I. A., W. Dieleman, S. Luyssaert, J.-A. Subke, M. Reichstein, R. Ceuemans, P. Ciais, et al. 2010. “Reduction of Forest Soil Respiration in Response to Nitrogen Deposition.” Nature Geoscience 3 (5): 315–322. doi:10.1038/NGEO844.
- Jobbágy, E. G., and R. B. Jackson. 2000. “The Vertical Distribution of Soil Organic Carbon and its Relation to Climate and Vegetation.” Ecological Applications 10 (2): 423−436. doi:10.1890/1051-0761(2000)010[0423:TVDOSO]2.0.CO;2.
- Karhu, K., E. Hilasvuori, H. Fritze, C. Biasi, H. Nykänen, J. Liski, P. Vanhala, J. Heinonsalo, and J. Pumpanen. 2016. “Priming Effect Increases with Depth in a Boreal Forest Soil.” Soil Biology and Biochemistry 99: 104–107. doi:10.1016/j.soilbio.2016.05.001.
- Kuzyakov, Y., J. K. Friedel, and K. Stahr. 2000. “Review of Mechanisms and Quantification of Priming Effects.” Soil Biology and Biochemistry 32 (11–12): 1485–1498. doi:10.1016/S0038-0717(00)00084-5.
- Lal, R. 2004. “Soil Carbon Sequestration Impacts on Global Climate Change and Food Security.” Science 304 (5677): 1623−1627. doi:10.1126/science.1097396.
- Moorhead, D. L., and R. L. Sinsabaugh. 2006. “A Theoretical Model of Litter Decay and Microbial Interaction.” Ecological Monographs 76 (2): 151–174. doi:10.1890/0012-9615(2006)076[0151:ATMOLD]2.0.CO;2.
- Mueller, P., K. Jensen, and J. P. Megonigal. 2015. “Plants Mediate Soil Organic Matter Decomposition in Response to Sea Level Rise.” Global Change Biology 22 (1): 404–414. doi:10.1111/gcb.13082.
- Ohtsuka, T., T. Akiyama, Y. Hashimoto, M. Inatomi, T. Sakai, S. Jia, W. Mo, S. Tsuda, and H. Koizumi. 2005. “Biometric Based Estimates of Net Primary Production (NPP) in a Cool-temperate Deciduous Forest Stand beneath a Flux Tower.” Agric For Meteorol 134 (1–4): 27–38. doi:10.1016/j.agrformet.2005.11.005.
- Shindo, H., and H. Honma. 2001. “Significance of Burning Vegetation in the Formation of Black Humic Acids in Japanese Volcanic Ash Soils.” In Humic Substances, Structures, Models and Function, edited by E. A. Ghabbour and G. Davies, 293–306. Cambridge UK: Royal Society of Chemistry.
- Stuiver, M., and H. A. Polach. 1977. “Discussion: Reporting of 14C Data.” Radiocarbon 19 (3): 355–363. doi:10.1017/S0033822200003672.
- Wada, K., and T. Higashi. 1976. “The Categories of Aluminium- and Iron-humus Complexes in Ando Soils Determined by Selective Dissolution.” Journal of Soil Science 27 (3): 357–368. doi:10.1111/j.1365-2389.1976.tb02007.x.
- Wang, Q., Y. Wang, S. Wang, T. He, and L. Liu. 2014. “Fresh Carbon and Nitrogen Inputs Alter Organic Carbon Mineralization and Microbial Community in Forest Deep Soil Layers.” Soil Biology and Biochemistry 72: 145–151. doi:10.1016/j.soilbio.2014.01.020.
- Watanabe, A., and H. Takeda. 2006. “Structural Stability and Natural 13C Abundance of Humic Acids in Buried Volcanic Ash Soils.” Soil Science and Plant Nutrition 52 (2): 145–152. doi:10.1111/j.1747-0765.2006.00029.x.
- Watanabe, A., and N. Fujitake. 2008. “Comparability of Composition of Carbon functional Groups in Humic Acids between Inverse-gated Decoupling and Cross Polarization/magic Angle Spinning 13C Nuclear Magnetic Resonance Techniques.” Analytica Chimica Acta 618 (1): 110–115. doi:10.1016/j.aca.2008.04.045.
- Yamamoto, S., T. Honna, and K. Utsumi. 2000. “Easy Estimation of Humic Acid Types by Using Meranic Index.” Japanese Journal of Soil Science and Plant Nutrition 59: 82–85.