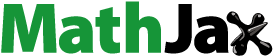
ABSTRACT
Arctic wetlands are significant sources of atmospheric methane and the observed accelerated climate changes in the arctic could cause a change in methane dynamics. Methane oxidation would be the key process to control methane emission from wetlands. In this study, we determined the potential methane oxidation rate of the wetland soils of a taiga–tundra transition zone in northeastern Siberia. Peat soil samples were collected in summer from depressions covered with tussocks of sedges and Sphagnum spp. and from mounds vegetated with moss and larch trees. An aerobic bottle incubation experiment demonstrated that the soil samples collected from depressions in the moss- and sedge-dominated zones exhibited active methane oxidation with no time lag, while the mound soils showed no methane oxidation under the given conditions. The potential methane oxidation rates of the soils at 15°C ranged from 94 to 496 nmol h−1 g−1 dw. The immediate and active methane oxidation was observed over the depths studied (0–40 cm) including the water-saturated anoxic layers; the maximum methane oxidation rate was recorded in the layer above the water-saturated layer. The methane oxidation rate was temperature-dependent, but substantial methane oxidation was observed even at 0°C particularly for the moss soil samples. Soil samples collected from the frozen layer of Sphagnum peat also showed immediate methane consumption when incubated at 15°C. The present results suggest that the methane oxidizing bacteria in the wetland soils could survive under anoxic and frozen conditions keeping their potential activities and immediately utilize methane when the conditions become favorable. On the other hand, the inhibitor of methane oxidation (difluoromethane) did not affect the methane flux from the sedge and moss zones in situ, which suggested the minor role of plant-associated methane oxidation.
1. Introduction
Methane is a greenhouse gas produced in natural and anthropogenic anaerobic environments as the terminal product of organic decomposition. Arctic wetlands, where large amounts of organic carbon are stored (Tarnocai et al. Citation2009; Hugelius et al. Citation2014), are one of the largest sources of atmospheric methane (Kirschke et al. Citation2013) (Intergovernmental_Panel_on_Climate_Change Citation2014). Methane emission from the Arctic wetlands could be increased by climate changes that include increasing temperatures, changing precipitation patterns, and permafrost thaw (Olefeldt et al. Citation2013; Schuur et al. Citation2015; Treat et al. Citation2015).
Methane emission from the wetlands to the atmosphere is the result of the balance between methane production and consumption. It is estimated that 50 Tg of methane is annually produced in boreal wetlands and 15 Tg of produced methane is consumed before emitted to the atmosphere (Reeburgh Citation2007). The oxic–anoxic interfaces such as the surface of the wetland soils and the rhizosphere of aerenchymatous plants are often characterized by the active methane oxidation thus playing a key role in controlling methane flux from the wetlands (Zhuang et al. Citation2004; Preuss et al. Citation2013).
The methane oxidation in arctic wetland soils has been reported repeatedly. In many studies, the potential methane oxidation was determined using an incubation experiment in which the collected samples were aerobically incubated with high concentrations of methane, and its controlling factors have been studied (Wagner, Horn, and Daims Citation2003; Wagner et al. Citation2005; Knoblauch et al. Citation2008; Christiansen et al. Citation2015). The potential methane oxidation rate differs spatially and temporally under the influence of different environmental conditions. For example, Wagner, Horn, and Daims (Citation2003) reported that methane oxidation in polygon depression in the Lena Delta, Siberia, was higher than in polygon rim with the increasing rate and expanding active depth with time in summer. The methane oxidation rate at the in situ temperature (0.4–7.5°C) ranged 1.9 − 7.0 nmol h−1 g−1 except for the boundary to the frozen ground where no methane oxidation was recorded (Wagner, Horn, and Daims Citation2003; Wagner et al. Citation2005). Much higher potential methane oxidation rates were recorded in permafrost-affected soils of Northeast Siberia with 45–87 nmol h−1 g−1 for mineral soils and 835 nmol h−1 g−1 for organic soil at the in situ temperature (5°C) (Knoblauch et al. Citation2008); 8–32% of the maximum oxidation rate was observed at 0°C. Thus, water level, soil depth, and temperature are the major factors that affect the methanotrophic activity in the arctic wetlands.
Aerenchymatous plants provide a niche for methane oxidizing bacteria in the rhizosphere where oxygen and methane are both available in wetlands (Frenzel Citation2000). Moss has a symbiotic association with methanotrophs: methanotrophs use oxygen supplied from moss to oxidize methane and moss utilizes CO2 produced by methanotrophs for photosynthesis (Raghoebarsing et al. Citation2005; Kip et al. Citation2010, Citation2011; Larmola et al. Citation2010; Liebner et al. Citation2011). The specific inhibitors such as methyl fluoride (CH3 F) and difluoromethane (CH2F2) are used to estimate the contribution of plant-associated methane oxidation to methane flux in wetland soils (Frenzel and Bosse Citation1996; Frenzel and Rudolph Citation1998; Kruger, Frenzel, and Conrad Citation2001). In this technique, it is assumed that the inhibitor injected in a flux chamber diffuses to the oxic part of a system, where inhibits methane oxidation, thus allowing estimation of methane oxidation in situ by comparing methane fluxes under the conditions with and without the inhibitor. The estimated contribution of methane oxidation to the total methane flux from the wetland rice determined by specific inhibition with difluoromethane ranged from 0% to 40% (Kruger, Frenzel, and Conrad Citation2001), while no contribution of methane oxidation associated with Eriophorum was observed in the bog of Estonia (Frenzel and Rudolph Citation1998). The contribution of plant-associated methane oxidation to methane flux has been poorly studied in the arctic wetlands (Liebner et al. Citation2011; Nielsen et al. Citation2017).
Most studies to determine the potential methane oxidation rates of the arctic wetlands have been done by in vitro incubations; the target samples were transferred from the study sites to the laboratory and the methane oxidation activity was measured after some time of storage, which may affect the enzymatic activities of soils depending on the type (Burns et al. Citation2013). Also, it is not clear if the measured methane oxidation represents the actual potential of the collected samples or if the methanotrophic activity was induced by incubation because the temporal change in methane concentration in the system is poorly documented in the incubation experiments. In this study, we measured the potential methane oxidation of the wetland soils in the northeastern Siberia immediately (<24 h) after sample collection to avoid possible bias caused by sample storage as much as possible. The incubation experiments were conducted to study the depth profile of the potential methane oxidation of wetland soils under different conditions and the temperature dependence of potential methane oxidation. As microbial growth in Arctic soil could be limited by the availability of nutrients like nitrogen (Sistla, Asao, and Schimel Citation2012), the effect of minerals on the potential methane oxidation was also studied by adding nutrients mimicking the transfer from the sea and forest fire to the arctic region (de Caritat et al. Citation2005). Besides the potential methane oxidation of soils, we estimated plant-associated in situ methane oxidation for the first time in the arctic wetlands by measuring the methane fluxes using the specific inhibitor of methane oxidation (difluoromethane).
2. Materials and methods
2.1. Sample collection
Soil samples were collected from the thawed (active) layer of the wetland in a taiga–tundra transition zone along the tributary of Indigirka River (N 70°33.8ʹ, E 148°15.9ʹ) in northeastern Siberia, Russia (Supplementary Fig. S1a & b), during the summer (July) of 2012–2015. Samples collected and experiments conducted for each year are summarized in . The study site was described before with the name of Kryvaya or site K (Iwahana et al. Citation2014; Liang et al. Citation2014; Morozumi et al. Citation2019; Takano et al. Citation2019). Three representative vegetation types were selected: two depression zones that were dominantly covered with moss-wet (Sphagnum spp., Supplementary Fig. S1 c) or tussocks of sedges (Carex spp., Supplementary Fig. S1d) and a dry mound vegetated with moss and larch trees (Supplementary Fig. S1e). The height difference between the depression zones and mound was approximately 40 cm and the maximum depth of active layer ranged from 20 to 40 cm (Morozumi et al. Citation2019; Takano et al. Citation2019). The ground water level in the depression zones ranged from 0 to 10 cm below the soil surface during the period of the study. The vertical profiles of soil total carbon and bulk density determined in 2011 are shown in Supplementary Figs. S2 and S3.
Table 1. Summary of samples used and experimental setup
Blocks of the surface soil (0–10 cm) were collected in triplicate using a serrated knife in 2012 and 2014; dry mound soil was collected only in 2012. Different layers (0–2, 4–6, and 8–10 cm) of soil were subsampled in 2013. Soil samples in the deeper layer including the surface part of the frozen layer (below 30 and 37 cm in wet-moss and sedge depressions, respectively) were also collected from the depression zones in 2015 using a metallic core sampler (i.d., 4 cm; length, 80 cm). Collected samples were stored at 4°C and subjected to measurement of potential methane oxidation within a day.
2.2. Vertical profiling of dissolved oxygen in soil
The vertical profile of dissolved oxygen in peat soil of the sedge and moss wetlands was measured by inserting a DO meter (HI 2040–01, Hanna Instruments, RI, U.S.A.) into small wells (diameter: ca. 1.5 cm) that were made by drilling the peat with a wooden stick a few days prior to measurement. The soil was water saturated and we measured the DO of the saturated water. The ground water table depth was about 1.5–2 cm below the soil surface when measured.
2.3. Methane oxidation potential of soil samples
Samples collected were gently pressed to remove the surplus water and homogenized by cutting into pieces (<5 mm) with scissors and mixing. The water content of homogenized samples was gravimetrically determined by heating subsamples at 80°C for 48 h. Ten grams of wet subsamples put into 50-ml or 100-ml GC vials (Nichiden-Rika Grass, Kobe, Japan). Frozen samples were thawed at 4°C before homogenized. The vials were capped with butyl rubber stoppers and open top screw caps and injected with 0.5 (in 2012) or 1.0 (in the other years) ml of 99% methane to give an initial concentration of ca. 5,000 or 10,000 ppmv in the headspace containing atmospheric air. The samples were incubated in the dark at 15°C. Methane concentration in the headspace was monitored using a photoacoustic field gas monitor (Innova 1412, LumaSense Technologies, Ballerup, Denmark). The small volume of the headspace (1 ml) was subsampled and injected into an empty and closed GC vial, and the methane concentration of the GC vial injected with the headspace gas was determined by circulating with the gas monitor. The methane oxidation rate was calculated from the linear regression of the methane concentration decreasing with time in 2 days (49–55 h). The methane oxidation rate was expressed per dry weight of samples that was obtained by drying at 80°C for 48 h.
To examine the effect of nutrients and black carbon – potential atmospheric depositions in the Arctic – on methane oxidation, the samples (10 g) collected in 2012 were applied with 1 ml of inorganic solution (10 μM NH4NO3, 250 μM NaCl, 40 μM CaCl2, 20 μM MgSO4, 10 μM KCl) and/or 1 ml of 100 μg l−1 charcoal powder of oak (Quercus L., <47 μm). The soil incubation was done as described above.
Temperature dependence of methane oxidation was studied as described above under different incubation temperatures (0°C, 5°C, 10°C and 15°C) using the surface layer soils of the moss- and sedge-dominated wetlands collected in 2013. The temperature coefficient (Q10) of methane oxidation was calculated for three temperature ranges (0°C to 5°C, 5°C to 10°C, and 10°C to 15°C):
where R1 and R2 are the methane oxidation rates at the temperatures of T2 and T1 (T1 < T2), respectively.
2.4. Estimation of the effect of plant-associated methane oxidation to in situ methane flux
The contribution of methane oxidation associated with the wetland plants to methane flux was estimated using difluoromethane (CH2F2) in 2014 and 2015 according to Kruger, Frenzel, and Conrad (Citation2001). Methane flux from the wetlands with different vegetation types was measured by a closed chamber method in which the surface of the wetlands was covered with a plexiglass chamber (height: 25 cm, inner diameter: 24.5 cm). Gas samples in the headspace were taken every 15 min into 20-ml pre-vacuumed vials for 3 times (0, 15, and 30 min). After the first flux measurement, the gas phase in the chamber was refreshed with atmospheric air by using a pump and then injected with CH2F2 at the concentration of 1% (v/v). Then, the second measurement of methane flux was done 10–15 min after injection of the inhibitor in 2014; as no influence of the inhibitor on the methane flux was observed, we extended the exposure time of the inhibitor to 18–19 h in 2015 in order to verify the result in 2014. Methane concentration in the collected gas samples were determined by an FID-GC (GC-14B, Shimadzu, Kyoto, Japan) and the methane flux before and after the injection of the inhibitor was calculated from the linear regression of methane concentration with time. At least two chambers were set per site and methane flux measurement without the inhibitor was conducted in parallel to monitor the temporal shift of methane flux which could affect our interpretation of the effect of the inhibitor on methane flux.
2.5. Statistical analysis
Differences in methane oxidation rate between treatments were tested with a one-way ANOVA followed by Tukey’s multiple comparison and the effect of CH2F2 on methane emission was assessed by Wilcoxon’s test using SPSS for Windows Ver. 22.0.
3. Results
3.1. The relationship between the vegetation types and CH4 oxidation
The methane concentration in the headspace of the bottle with peat samples from wetlands with moss and sedge vegetations rapidly decreased with time from the initial concentration (ca. 5,000 ppmv) to an atmospheric level in 7 days ( & b). On the other hand, the sample from the moss-dominated mound did not show any activity of methane oxidation under the given conditions ().
Figure 1. Temporal change of methane concentration in the headspace of the microcosms with soil samples collected from the different vegetation types in 2012 (a, moss; b; sedge; c, moss in the mound) treated with inorganic nutrient and black carbon. Bars indicate the standard error (n = 3)
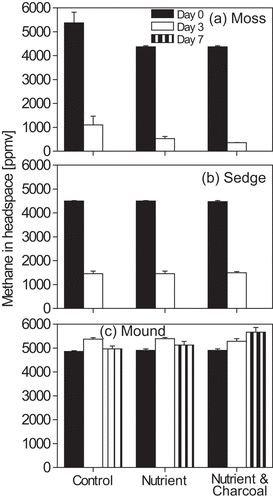
Addition of nutrients and charcoal powder did not affect methane oxidation of any samples. We also tested 10 times higher concentrations of nutrients and charcoal powder but found no influence on methane oxidation of all the samples (data not shown).
3.2. Vertical profile of CH4 oxidation and DO in pore water
The time course incubation experiment using the different layers of surface (0–10 cm) wetland peat samples in depressions in 2013 showed that methane was oxidized with no lag period ( & b). Immediate methane oxidation of the moss peat sample was also observed in the deeper layers in the 2015 measurement even in the frozen layer (30–40 cm) (). Peat samples from the sedge-dominated wetland up to 20 cm in depth also showed active methane oxidation, but the mineral frozen soil collected from below the organic layer of the sedge-dominated wetland in 2015 exhibited no detectable methane oxidation during the incubation period (). The calculated methane oxidation rate of the organic layer soils ranged from 54 to 496 nmol h−1 g−1 (). The moss peat samples had higher rates per dry weight basis than the sedge peat samples; this was contrasting with the higher decreasing rate of methane concentration in the head space in the sedge peat sample, which was attributed to the higher water content of moss peat samples (93–94%[w/w]) than the sedge peat samples (75–85%[w/w]). The highest activity of methane oxidation was recorded at the middle (4–6 cm, 496 nmol h−1 g−1) layer and the top (0–2 cm, 181 nmol h−1 g−1) layer of moss and sedge peat samples, respectively.
Table 2. Estimated potential methane oxidation rate of soil samples from the wetlands of northeastern Siberia (average ± std error, n = 3)
Figure 2. Methane oxidation by the different depth layers of moss- and sedge- dominated soils in 2013 (a and b) and 2015 (c and d). Bars indicate the standard error (n = 3)
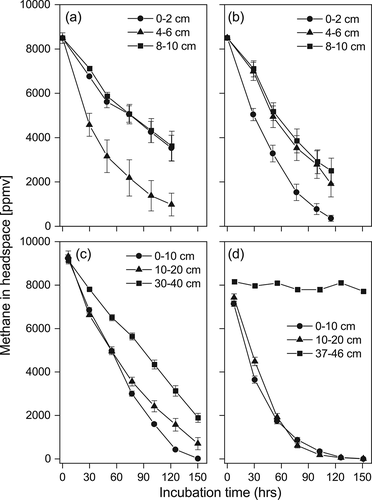
The in situ concentration of dissolved oxygen in the pore water of the wetlands was very low and undetectable below 10 cm with one exception in the sedge wetland ().
3.3. Temperature dependence of methane oxidation
Methane oxidation of surface (0–10 cm) peat samples from moss and sedge wetlands showed a clear temperature dependence. A linear decrease in methane concentration during incubation was observed even at 0°C ( & b). The methane oxidation rate of the sample from the moss-dominated wetland at 0°C did not differ from that at 5°C (). Q10 ranged from 1.13 to 2.10 with an exclusively low value for the moss peat samples incubated at 0°C and 5°C ().
Figure 4. Effect of incubation temperature on methane oxidation by (a) moss and (b) sedge-dominated peat samples and (c) the temperature dependence of the methane oxidation rate (0–10 cm) (2014). Bars indicate the standard error (n = 3). Data marked with different letters are significantly different (P < 0.05, as determined by Tukey’s honestly significant difference test)
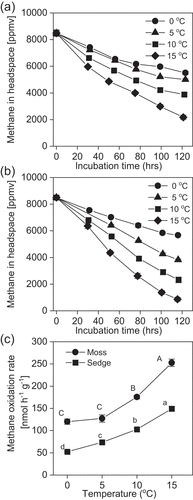
3.4. Effect of the inhibitor on methane emission from the wetland with different vegetations
The methane flux in the first measurement ranged from 2.4 to 1,800 μmol h−1 m−2 (). The methane flux in the second measurement ranged from 0.19 to 1,840 μmol h−1 m−2 and the inhibitor did not affect the methane flux in the second measurement for all the vegetations; this was demonstrated by the 1:1 relationship between the first and second measurements either with or without injection of the inhibitor. The prolonged exposure time of the inhibitor from 10–15 min (in 2014) to 18–19 h (in 2015) did not cause difference.
4. Discussion
The wetland soils in the depression area of the taiga–tundra transition zone in the northeastern Siberia exhibited the active methane oxidation in the incubation experiment. The potential rates estimated in this study (54–496 nmol h−1 g−1) were at the higher end of the rates measured in other Arctic regions including Siberia using a similar headspace concentrations of methane (0–835 nmol h−1 g−1, Knoblauch et al. Citation2008; 50–66 nmol h−1 g−1; Christiansen et al. Citation2015). The highest rate was recorded at the subsurface (4–6 cm) and surface (0–2 cm) of the moss and sedge-dominated wetlands, respectively; these depths corresponded to the ground water level of the study site, where the maximum methane oxidation rate has been often reported in other wetlands (e.g., Vecherskaya et al. Citation1993; Sundh et al. Citation1995; Whalen and Reeburgh Citation2000). The color of the soils collected from layers under the ground water level darkened soon after sample collection in 2013 and 2015. This is most likely due to a browning reaction exposed to oxygen, which in turn indicates the soil below the ground water was under anoxic conditions. The vertical profile of DO verified the low oxygen concentration in the soil under the ground water, though the measurement was done in the different year (2014). On the other hand, the mound soil in the same area showed no methane oxidation under the experimental conditions of this study that targeted low-affinity methane oxidation. The mound has a low water level giving the drained conditions. Our previous study demonstrated that the soil in the mound is characterized by the positive redox potential (Shingubara et al. Citation2019); thus, the methane production is very low and the low-affinity methane oxidation, which is adapted to high concentration of methane, may be also low in the mound soil. The results show the spatial heterogeneity of the potential methane oxidation of the soils in the taiga-tundra ecotone in northeastern Siberia at inter- and intra-regional scales. We tested our hypothesis that the methane oxidation of the soils may be constrained by limited amounts of mineral nutrients including nitrogen, but application of the mineral salts and charcoal that would be transferred from the sea or forest fire (de Caritat et al. Citation2005) did not affect methane oxidation.
Plotting the data in the time-course measurement showed that the wetland soils exhibited methane oxidation without time lag when incubated. The immediate methane oxidation was observed throughout the different soil depths in this study except for the mineral soil in the frozen layer of the sedge-dominated wetlands that exhibited no detectable methane oxidation. The immediate methane oxidation upon thaw is also reported for the frozen permafrost soil from a black-spruce forest in Alaska (Mackelprang et al. Citation2011). The high methane oxidation potential throughout the active layer is in contrast with the stable isotope signals of dissolved methane that indicated that methane oxidation is limited to the surface layer (up to 10 cm) of the soils in the same study site (Shingubara et al. Citation2019). This suggests that methanotrophs in the deeper layers actually do not oxidize methane in situ but survive for a prolonged time under the anoxic/frozen conditions; they keep the potential activity and would be able to immediately oxidize methane when the soil become oxic by decrease in the ground water level (Parmentier et al. Citation2011; Shingubara et al. Citation2019). Roslev and King (Citation1996) reported that peat samples from the freshwater marsh maintain 30% of the initial methane oxidation capacity after 32 days of anoxic incubation and methanotrophs from anoxic peat initiated aerobic methane oxidation within 1–7 hours after oxygen addition.
A clear temperature dependence on potential methane oxidation was observed in the top 10 cm layer of the wetland soils. The result is mostly consistent with the previous reports (e.g., Knoblauch et al. Citation2008). One different observation was that the soil from the moss wetland incubated at 0°C showed a comparative activity of methane oxidation to the soil incubated at 5°C with the low value of Q10 (1.13 between 0°C and 5°C). This suggests that the methanotrophs in the soil sample from the moss wetland would be less sensitive to change in the low temperatures compared to the sedge-dominated wetland soil sample and implies that methanotrophs in the soil samples of moss and sedge-dominated wetlands could differently respond to the increasing temperature in future. The depth profile of methane oxidation potential was estimated at 15°C, but the deeper sample could have the higher activity at the lower optimum temperature (Liebner and Wagner Citation2007).
The methane emission rate in the moss- and sedge-dominated wetlands observed in this study ranging from 7.36 to 1,840 μmol m−2 h−1 was mostly comparable to or more than that reported in the previous studies (Cao, Gregson, and Marshall Citation1998; Kutzbach, Wagner, and Pfeiffer Citation2004; Petrescu et al. Citation2008). Addition of the inhibitor of methane oxidation did not affect the methane flux from all the vegetations studied. Stable-isotopic studies of dissolved methane in the Alaska Tundra indicate the minor role of methane oxidation during the transport from the deeper layer to the surface (Throckmorton et al. Citation2015). The minor role of plant-associated methane oxidation in methane emission from aerenchymatous plants in arctic peatlands was also demonstrated by the microcosm study in Greenland using 13CH4 labeling (Nielsen et al. Citation2017). The potential activity of methanotrophs may be sustained by the release of oxygen from the aerenchymatous plant roots at the very low level (Nielsen et al. Citation2017), which may not affect the methane flux from the vegetation. The low in situ temperature could be another reason for the undetectable level of the rhizospheric methane oxidation (Saarnio et al. Citation1997).
A symbiotic relationship between methanotrophs and wetland mosses is well known for Sphagnum species (Basiliko, Knowles, and Moore Citation2004; Raghoebarsing et al. Citation2005; Kip et al. Citation2010) and also for brown mosses (Liebner et al. Citation2011). The lack of an effect of the added inhibitor in the headspace on the methane flux from the moss-dominated wetlands suggests that moss-associated methanotrophs may not use oxygen diffused from the atmosphere unlike aerenchymatous plants but use oxygen released from moss by photosynthesis (Raghoebarsing et al. Citation2005). The moss peat samples sustained the methane oxidation potential over the depth studied. Moss-associated methanotrophs may keep their potential activity even after the moss is dead and accumulated in the deeper layer where the conditions are not favorable for methane oxidation due to the anoxia (King Citation1996).
In conclusion, the wetland soils of the taiga-tundra ecotone in northeastern Siberia keep the high methane oxidation potential even under anaerobic/frozen conditions that is expressed upon aerobic incubation with methane. The difference in temperature dependence on methane oxidation at the lower temperatures between the moss- and sedge-dominated wetland soils would give an insight for understanding and predicting methane dynamics in the arctic wetland under global warming. The vertical shift of the oxic–anoxic interface caused by the fluctuation of the water level may not affect the methane oxidation at the site as the methane oxidation potential is maintained over the depth. As microbial community in the arctic wetlands is geographically heterogeneous (Jansson et al., Citation2014), ecology of methane oxidizing bacteria actively involved in the methane cycle in the wetland of northeastern Siberia should be studied considering the vegetation types to better understand methane dynamics in this region.
Fig._S3_soil__bulk_density.pdf
Download PDF (122 KB)SSPN_Fig._S2.pdf
Download PDF (93.3 KB)SSPN_Fig._S1.png
Download PNG Image (1,019 KB)Acknowledgments
This research was partly supported by Grant-in-Aids, the Global COE Program “Establishment of Center for Integrated Field Environmental Science” (IFES-GCOE) from the Ministry of Education, Culture, Sports, Science and Technology-Japan (MEXT), the Green Network of Excellence (GRENE) Program funded by MEXT, and the COPERA (C budget of ecosystems and cities and villages on permafrost in eastern Russian Arctic) project funded by the Belmont Forum, and JST. This work was also supported by JSPS KAKENHI (Grants-in-Aid for Scientific Research) [16H05618] to J.M. The authors thank Y. Hoshino, A. Kononov, R. Petrov, E. Starostin, T. Stryukova, S. Ianygin for supporting our fieldwork.
Disclosure statement
The authors declare that they have no conflict of interest.
Supplementary material
Supplemental data for this article can be accessed here.
Correction Statement
This article has been republished with minor changes. These changes do not impact the academic content of the article.
Additional information
Funding
References
- Basiliko, N., R. Knowles, and T. R. Moore. 2004. “Roles of Moss Species and Habitat in Methane Consumption Potential in a Northern Peatland.” Wetlands 24 (1): 178–185. doi:https://doi.org/10.1672/0277-5212(2004)024[0178:ROMSAH]2.0.CO;2.
- Burns, R. G., J. L. DeForest, J. Marxsen, R. L. Sinsabaugh, M. E. Stromberger, M. D. Wallenstein, M. N. Weintraub, and A. Zoppini. 2013. “Soil Enzymes in a Changing Environment: Current Knowledge and Future Directions.” Soil Biology and Biochemistry 58 (Supplement C): 216–234. doi:https://doi.org/10.1016/j.soilbio.2012.11.009.
- Cao, M., K. Gregson, and S. Marshall. 1998. “Global Methane Emission from Wetlands and Its Sensitivity to Climate Change.” Atmospheric Environment 32 (19): 3293–3299. doi:https://doi.org/10.1016/S1352-2310(98)00105-8.
- Christiansen, J. R., A. Jose Barrera Romero, N. O. G. Jørgensen, M. Andreas Glaring, C. Juncher Jørgensen, L. Kristine Berg, and B. Elberling. 2015. “Methane Fluxes and the Functional Groups of Methanotrophs and Methanogens in a Young Arctic Landscape on Disko Island, West Greenland.” Biogeochemistry 122 (1): 15–33. doi:https://doi.org/10.1007/s10533-014-0026-7.
- de Caritat, P., G. Hall, S. Gislason, W. Belsey, M. Braun, N. I. Goloubeva, H. K. Olsen, J. O. Scheie, and J. E. Vaive. 2005. “Chemical Composition of Arctic Snow: Concentration Levels and Regional Distribution of Major Elements.” Science of the Total Environment 336 (1–3): 183–199. doi:https://doi.org/10.1016/j.scitotenv.2004.05.031.
- Frenzel, P. 2000. “Plant-associated Methane Oxidation in Rice Fields and Wetlands.” In Advances in Microbial Ecology, edited by B. Schink, 85–114. Boston: Springer.
- Frenzel, P., and J. Rudolph. 1998. “Methane Emission from a Wetland Plant: The Role of CH4 Oxidation in Eriophorum.” Plant and Soil 202 (1): 27–32. doi:https://doi.org/10.1023/a:1004348929219.
- Frenzel, P., and U. Bosse. 1996. “Methyl Fluoride, an Inhibitor of Methane Oxidation and Methane Production.” Fems Microbiology Ecology 21 (1): 25–36. doi:https://doi.org/10.1111/j.1574-6941.1996.tb00330.x.
- Hugelius, G., J. Strauss, S. Zubrzycki, J. W. Harden, E. Schuur, C.-L. Ping, L. Schirrmeister, G. Grosse, G. J. Michaelson, and C. D. Koven. 2014. “Estimated Stocks of Circumpolar Permafrost Carbon with Quantified Uncertainty Ranges and Identified Data Gaps.” Biogeosciences 11 (23): 6573–6593. doi:https://doi.org/10.5194/bg-11-6573-2014.
- Intergovernmental_Panel_on_Climate_Change. 2014. Climate Change 2013: The Physical Science Basis: Working Group I Contribution to the Fifth Assessment Report of the Intergovernmental Panel on Climate Change. Cambridge University Press.
- Iwahana, G., S. Takano, R. E. Petrov, S. Tei, R. Shingubara, T. C. Maximov, A. N. Fedorov, et al. 2014. “Geocryological Characteristics of the Upper Permafrost in a Tundra-forest Transition of the Indigirka River Valley, Russia.” Polar Science 8 (2): 96–113. doi:https://doi.org/10.1016/j.polar.2014.01.005.
- Jansson, J., and N. Taş. 2014. “The microbial ecology of permafrost”. Nature Reviews Microbiology 12: 414–425. doi: https://doi.org/10.1038/nrmicro32626
- King, G. M. 1996. “Physiological Liminations of Methanotrophic Activity in Situ.” In Microbiology of Atmospheric Trace Gases: Sources, Sinks an Global Change Processes, edited by J. C. Murrell and D. P. Kelly, 17–32. Berlin: Springer.
- Kip, N., J. F. van Winden, Y. Pan, L. Bodrossy, G.-J. Reichart, A. J. P. Smolders, M. S. M. Jetten, J. S. S. Damste, and H. J. M. Op den Camp. 2010. “Global Prevalence of Methane Oxidation by Symbiotic Bacteria in Peat-moss Ecosystems.” Nature Geoscience 3 (9): 617–621. doi:https://doi.org/10.1038/Ngeo939.
- Kip, N., W. Ouyang, J. van Winden, A. Raghoebarsing, L. van Niftrik, A. Pol, Y. Pan, et al. 2011. “Detection, Isolation, and Characterization of Acidophilic Methanotrophs from Sphagnum Mosses.” Applied and Environmental Microbiology 77 (16): 5643–5654. doi:https://doi.org/10.1128/aem.05017-11.
- Kirschke, S., P. Bousquet, P. Ciais, M. Saunois, J. G. Canadell, E. J. Dlugokencky, P. Bergamaschi, D. Bergmann, D. R. Blake, and L. Bruhwiler. 2013. “Three Decades of Global Methane Sources and Sinks.” Nature Geoscience 6 (10): 813. doi:https://doi.org/10.1038/Ngeo1955.
- Knoblauch, C., U. Zimmermann, M. Blumenberg, W. Michaelis, and E.-M. Pfeiffer. 2008. “Methane Turnover and Temperature Response of Methane-oxidizing Bacteria in Permafrost-affected Soils of Northeast Siberia.” Soil Biology & Biochemistry 40 (12): 3004–3013. doi:https://doi.org/10.1016/j.soilbio.2008.08.020.
- Kruger, M., P. Frenzel, and R. Conrad. 2001. “Microbial Processes Influencing Methane Emission from Rice Fields.” Global Change Biology 7 (1): 49–63. doi:https://doi.org/10.1046/j.1365-2486.2001.00395.x.
- Kutzbach, L., D. Wagner, and E.-M. Pfeiffer. 2004. “Effect of Microrelief and Vegetation on Methane Emission from Wet Polygonal Tundra, Lena Delta, Northern Siberia.” Biogeochemistry 69 (3): 341–362. doi:https://doi.org/10.1023/B:BIOG.0000031053.81520.db.
- Larmola, T., E.-S. Tuittila, M. Tiirola, P. J. Hannu Nykanen, K. Y. Martikainen, T. Tuomivirta, and H. Fritze. 2010. “The Role of Sphagnum Mosses in the Methane Cycling of a Boreal Mire.” Ecology 91 (8): 2356–2365. doi:https://doi.org/10.1890/09-1343.1.
- Liang, M., A. Sugimoto, S. Tei, I. V. Bragin, S. Takano, T. Morozumi, R. Shingubara, et al. 2014. “Importance of Soil Moisture and N Availability to Larch Growth and Distribution in the Arctic Taiga-tundra Boundary Ecosystem, Northeastern Siberia.” Polar Science 8 (4): 327–341. doi:https://doi.org/10.1016/j.polar.2014.07.008.
- Liebner, S., and D. Wagner. 2007. “Abundance, Distribution and Potential Activity of Methane Oxidizing Bacteria in Permafrost Soils from the Lena Delta, Siberia.” Environmental Microbiology 9 (1): 107–117. doi:https://doi.org/10.1111/j.1462-2920.2006.01120.x.
- Liebner, S., J. Zeyer, D. Wagner, C. Schubert, E.-M. Pfeiffer, and C. Knoblauch. 2011. “Methane Oxidation Associated with Submerged Brown Mosses Reduces Methane Emissions from Siberian Polygonal Tundra.” Journal of Ecology 99 (4): 914–922. doi:https://doi.org/10.1111/j.1365-2745.2011.01823.x.
- Mackelprang, R., M. P. Waldrop, K. M. DeAngelis, M. M. David, K. L. Chavarria, S. J. Blazewicz, E. M. Rubin, and J. K. Jansson. 2011. “Metagenomic Analysis of a Permafrost Microbial Community Reveals a Rapid Response to Thaw.” Nature 480 (7377): 368–371. doi:https://doi.org/10.1038/nature10576.
- Morozumi, T., R. Shingubara, R. Suzuki, H. Kobayashi, S. Tei, S. Takano, R. Fan, M. Liang, T. C. Maximov, and A. Sugimoto. 2019. “Estimating Methane Emissions Using Vegetation Mapping in the Taiga-tundra Boundary of a North-eastern Siberian Lowland.” Tellus Series B-Chemical and Physical Meteorology 71 (1): 1–17. doi:https://doi.org/10.1080/16000889.2019.1581004.
- Nielsen, C. S., A. Michelsen, T. K. K. Per Ambus, C. Deepagoda, and B. Elberling. 2017. “Linking Rhizospheric CH4 Oxidation and Net CH4 Emissions in an Arctic Wetland Based on 13CH4 Labeling of Mesocosms.” Plant and Soil 412 (1): 201–213. doi:https://doi.org/10.1007/s11104-016-3061-4.
- Olefeldt, D., M. R. Turetsky, P. M. Crill, and A. David McGuire. 2013. “Environmental and Physical Controls on Northern Terrestrial Methane Emissions across Permafrost Zones.” Global Change Biology 19 (2): 589–603. doi:https://doi.org/10.1111/gcb.12071.
- Parmentier, F. J. W., J. van Huissteden, M. K. van der Molen, G. Schaepman-Strub, S. A. Karsanaev, T. C. Maximov, and A. J. Dolman. 2011. “Spatial and Temporal Dynamics in Eddy Covariance Observations of Methane Fluxes at a Tundra Site in Northeastern Siberia.” Journal of Geophysical Research-Biogeosciences 116: G3. doi:https://doi.org/10.1029/2010jg001637.
- Petrescu, A. M. R., J. van Huissteden, M. Jackowicz-Korczynski, A. Yurova, T. R. Christensen, P. M. Crill, K. Backstrand, and T. C. Maximov. 2008. “Modelling CH4 Emissions from Arctic Wetlands: Effects of Hydrological Parameterization.” Biogeosciences 5 (1): 111–121. doi:https://doi.org/10.5194/bg-5-111-2008.
- Preuss, I., C. Knoblauch, J. Gebert, and E. M. Pfeiffer. 2013. “Improved Quantification of Microbial CH4 Oxidation Efficiency in Arctic Wetland Soils Using Carbon Isotope Fractionation.” Biogeosciences 10 (4): 2539–2552. doi:https://doi.org/10.5194/bg-10-2539-2013.
- Raghoebarsing, A. A., A. J. P. Smolders, M. C. Schmid, W. I. C. Rijpstra, M. Wolters-Arts, J. Derksen, M. S. M. Jetten, et al. 2005. “Methanotrophic Symbionts Provide Carbon for Photosynthesis in Peat Bogs.” Nature 436 (7054): 1153–1156. doi:https://doi.org/10.1038/nature03802.ISI:000231416600045.
- Reeburgh, W. S. 2007. “Oceanic Methane Biogeochemistry.” Chemical Reviews 107 (2): 486–513. doi:https://doi.org/10.1021/cr050362v.
- Roslev, P., and G. M. King. 1996. “Regulation of Methane Oxidation in a Freshwater Wetland by Water Table Changes and Anoxia.” FEMS Microbiology Ecology 19 (2): 105–115. doi:https://doi.org/10.1016/0168-6496(95)00084-4.
- Saarnio, S., J. Alm, J. Silvola, A. Lohila, H. Nykänen, and P. J. Martikainen. 1997. “Seasonal Variation in CH4 Emissions and Production and Oxidation Potentials at Microsites on an Oligotrophic Pine Fen.” Oecologia 110 (3): 414–422. doi:https://doi.org/10.1007/s004420050176.
- Schuur, E. A. G., A. D. McGuire, C. Schadel, G. Grosse, J. W. Harden, D. J. Hayes, G. Hugelius, et al. 2015. “Climate Change and the Permafrost Carbon Feedback.” Nature 520 (7546): 171–179. doi:https://doi.org/10.1038/nature14338.
- Shingubara, R., A. Sugimoto, J. Murase, G. Iwahana, S. Tei, M. Liang, S. Takano, T. Morozumi, and T. C. Maximov. 2019. “Multi-year Effect of Wetting on CH4 Flux at Taiga–tundra Boundary in Northeastern Siberia Deduced from Stable Isotope Ratios of CH4.” Biogeosciences 16 (3): 755–768. doi:https://doi.org/10.5194/bg-16-755-2019.
- Sistla, S. A., S. Asao, and J. P. Schimel. 2012. “Detecting Microbial N-limitation in Tussock Tundra Soil: Implications for Arctic Soil Organic Carbon Cycling.” Soil Biology and Biochemistry 55: 78–84. doi:https://doi.org/10.1016/j.soilbio.2012.06.010.
- Sundh, I., C. Mikkelä, M. Nilsson, and B. H. Svensson. 1995. “Potential Aerobic Methane Oxidation in a Sphagnum-dominated Peatland—controlling Factors and Relation to Methane Emission.” Soil Biology & Biochemistry 27 (6): 829–837. doi:https://doi.org/10.1016/0038-0717(94)00222-M.
- Takano, S., A. Sugimoto, S. Tei, M. Liang, R. Shingubara, T. Morozumi, and T. C. Maximov. 2019. “Isotopic Compositions of Ground Ice in Near-surface Permafrost in Relation to Vegetation and Microtopography at the Taiga–Tundra Boundary in the Indigirka River Lowlands, Northeastern Siberia.” Plos One 14 (10): e0223720. doi:https://doi.org/10.1371/journal.pone.0223720.
- Tarnocai, C., J. G. Canadell, E. A. G. Schuur, P. Kuhry, G. Mazhitova, and S. Zimov. 2009. “Soil Organic Carbon Pools in the Northern Circumpolar Permafrost Region.” Global Biogeochemical Cycles 23 (2): GB2023. doi:https://doi.org/10.1029/2008GB003327.
- Throckmorton, H. M., J. M. Heikoop, B. D. Newman, G. L. Altmann, M. S. Conrad, J. D. Muss, G. B. Perkins, et al. 2015. “Pathways and Transformations of Dissolved Methane and Dissolved Inorganic Carbon in Arctic Tundra Watersheds: Evidence from Analysis of Stable Isotopes.” Global Biogeochemical Cycles 29 (11): 1893–1910. doi:https://doi.org/10.1002/2014gb005044.
- Treat, C. C., S. M. Natali, J. Ernakovich, C. M. Iversen, M. Lupascu, A. D. McGuire, R. J. Norby, et al. 2015. “A pan-Arctic Synthesis of CH4 and CO2 Production from Anoxic Soil Incubations.” Global Change Biology 21 (7): 2787–2803. doi:https://doi.org/10.1111/gcb.12875.
- Vecherskaya, M. S., V. F. Galchenko, E. N. Sokolova, and V. A. Samarkin. 1993. “Activity and Species Composition of Aerobic Methanotrophic Communities in Tundra Soils.” Current Microbiology 27 (3): 181–184. doi:https://doi.org/10.1007/bf01576018.
- Wagner, M., A. Loy, M. Klein, N. Lee, N. B. Ramsing, D. A. Stahl, and M. W. Friedrich. 2005. “Functional Marker Genes for Identification of Sulfate-reducing Prokaryotes.” Methods Enzymol 397: 469–489. doi:https://doi.org/10.1016/S0076-6879(05)97029-8.
- Wagner, M., M. Horn, and H. Daims. 2003. “Fluorescence in Situ Hybridisation for the Identification and Characterisation of Prokaryotes.” Curr Opin Microbiol 6 (3): 302–309. https://www.ncbi.nlm.nih.gov/pubmed/12831908.
- Whalen, S. C., and W. S. Reeburgh. 2000. “Methane Oxidation, Production, and Emission at Contrasting Sites in a Boreal Bog.” Geomicrobiology Journal 17 (3): 237–251. doi:https://doi.org/10.1080/01490450050121198.
- Zhuang, Q., J. M. Melillo, D. W. Kicklighter, R. G. Prinn, A. D. McGuire, P. A. Steudler, B. S. Felzer, and S. Hu. 2004. “Methane Fluxes between Terrestrial Ecosystems and the Atmosphere at Northern High Latitudes during the past Century: A Retrospective Analysis with A Process-based Biogeochemistry Model.” Global Biogeochemical Cycles 18: 3. doi:https://doi.org/10.1029/2004gb002239.