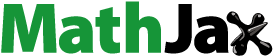
ABSTRACT
The increasing demand for sugarcane residue to produce industrial biofuels competes with organic-matter inputs to tropical soils for increasing fertility. Soil nitrogen (N) availability to plants and carbon (C) storage can be optimized by the selection of substrate types and quantities. We tested whether substrate-induced microbial growth increases soil N mineralization and C stabilization as substrate inputs increase. The effects of substrate additions (cane sugar, leaf litter, and humic acid extracted from filter cake) on microbial growth and N availability were studied using C3/C4 plant C isotopic signatures in a sandy soil of northeast Thailand. The initial growth of microbial biomass in cane sugar-amended soil caused a short-term flush of N derived from the native soil organic matter within 3 days, whereas in the soils amended with leaf litter or humic acid, N was retained in microbial biomass or dissolved organic matter, respectively. Net mineralization of the native soil organic N was maximized by sugar addition at a dosage corresponding to 10 folds of microbial biomass-C. The efficacy of substrates stabilized in the heavy density fraction (>1.6 g cm−3) followed the order: leaf litter > humic acid > sugar. Soil net N mineralization can be promoted by sugar inputs (e.g., bud node) at an early growth stage, while leaf litter and humic acid inputs can increase C storage efficiently in the sandy soil without increasing risks of N starvation and loss when they are applied at several tons of C per hectare at least 3 weeks before planting.
1. Introduction
The increasing demand for feedstock in bioenergy production may compete with crop residue amendment in arable lands. The sugarcane, as well as maize, is a common crop due to the high primary productivity (Carvalho et al. Citation2017). The removal of sugarcane straw reduces organic carbon (C) inputs and soil C storage (Carvalho et al. Citation2017; De Oliveira Bordonal et al. Citation2018). Moreover, the industrial and agricultural demands for using residues or waste, such as bagasse or filter cake (i.e., industrial fibrous residue from juice extraction), are increasing (Leal et al. Citation2013). The addition of sugarcane residues and wastes increases nutrient availability and C stabilization in most soils (Rasul et al. Citation2008). However, the effects of sugarcane-waste amendment on microbial growth and nitrogen (N) dynamics are unclear for sandy soils in northeast Thailand.
The amendment of organic matter in soil can influence the soil microbial biomass, soil C storage, and N availability, depending on substrate quantity and quality (Schimel and Weintraub Citation2003; Puttaso et al. Citation2011). During sugarcane production, leaf litter, and sugars in seed stems (bud node) may be applied to the soil. Sugarcane leaf ash after burning or filter-cake compost collected during sugarcane juice production is often supplied to the soils. The high availability of labile C (e.g., sugars) can stimulate microbial growth (Schneckenberger et al. Citation2008). The microbial necromass can contribute to the stabilization of soil C (Buckeridge et al. Citation2020). The previous study has reported that microbial biomass increases with substrate dose rather than substrate types (filter cake vs. compost) (Rasul et al. Citation2008).
On the other hand, substrate-induced microbial growth has various influences on soil N dynamics. The microbial N demand may increase competition for available N (i.e., fertilizer or soluble soil N) with plants, a phenomenon known as N starvation (Schimel and Bennett Citation2004). Microbial growth and enzyme production might also stimulate mineralization of the native soil organic N (insoluble N), leading to an increase in N availability to plants. This phenomenon is known as microbial N mining or positive priming effects in terms of C (Kuzyakov Citation2002). The dominant process could vary depending on substrate quantity and quality that affect the extent of microbial growth and N mining (Kuzyakov Citation2002).
Tropical sandy soils typically have the low contents of organic C and N, but microbial responses of substrate addition could be relatively sharp compared to the temperate soils or tropical clayey soils (Sugihara, Funakawa, and Kosaki Citation2010; Fujii et al. Citation2017). We aim to (1) characterize changes in microbial biomass and stabilized soil C in response to various substrate amendments, and (2) test whether N-poor sugar inputs increase microbial N mining, leading to the release of more inorganic N from the native soil organic N, compared with more recalcitrant substrates, such as leaf litter or humic acid.
To trace sugarcane-derived C in soil, we used the differences of C-isotope signatures (δ13C) between C4 and C3 plants. Sugarcane (C4 plant) and cassava (C3 plant), which are common crops in northeast Thailand, have different δ13C values due to their different photosynthetic pathways (Yoneyama et al. Citation2006). Sugarcane application to cassava-cultivated soils allows us to trace sugarcane-derived C dynamics. To test whether substrate-induced microbial growth increases soil N mineralization and organic-matter storage as substrate inputs increase, we investigated the effects of quality and quantity of sugarcane material inputs on microbial biomass, N availability, and soil C storage.
2. Materials and methods
2.1. Sampling soil material
Soil samples were collected in September 2017 from the surface mineral horizon (Ap horizon: 0–10 cm deep) at an upland field in northeast Thailand (Yasothon series; N15°08ʹ39.7ʹ’, E101°43ʹ54.7ʹ’). Sugarcane (Saccharum officinarum L.) is a common crop in the region studied. To trace sugarcane-derived C, we selected sites where cassava has been continuously cultivated (more than 20 years). Composite soil samples (comprising samples from three subplots) were passed through a 4-mm sieve and used for microbial biomass measurement and incubation studies. All experiments were conducted using a composite soil sample in three technical replicates.
2.2. Experimental design and incubation conditions
To study the effects of sugarcane-derived substrate inputs on soil C and N dynamics, a sugar solution, leaf litter, and humic acid were added to the soil and incubated for 3 weeks, respectively. Humic acid was extracted from the filtering cake of sugarcane juice using KOH and HCl (Asing, Wong, and Lau Citation2009). The filtering cake was supplied from the Eastern Sugar and Cane Public Company Limited, Sakeo Province, Thailand. We prepared the sugar solution and leaf litter from sugarcane cultivated in Okinawa, Japan. The sugar solution was extracted from the milled stem and condensed by heating.
After pre-incubation at 25°C for 7 days in the laboratory incubator (October 2017), the three substrates or water were added to soil samples (10 g) in a vial, respectively. The substrate-amended or deionized water-amended soil samples (control) were dosed at four nutrient levels: 0 mg C kg−1 soil (level 0), 263 mg C kg−1 soil (level 1), 2,630 mg C kg−1 soil (level 2), and 26,300 mg C kg−1 soil (level 3). The experimental dosages (i.e., excluding the control) were equivalent to the microbial biomass C (MBC) of the field condition (MBC = 263 mg C kg−1 soil), 10 times the MBC, and 100 times the MBC of the fresh soil samples, respectively. Soil MBC of the field condition was measured immediately after soil sampling in section 2.4. The soil water content was adjusted to 60% of the water-holding capacity (WHC) by adding distilled water (Öhlinger Citation1995). In total, the soil samples in 222 vials [1 dose level (level 0) × 1 sampling period (0 day) × 2 measurement items (MBC and soil C in heavy fraction) × 3 replicates + 4 dose levels (level 0–3) × 3 substrates × 3 sampling periods (3, 7, 21 days) × 2 measurement items × 3 replicates]. After incubation for 0, 3, 7, and 21 days, soil C in the heavy fraction, MBC, microbial biomass N (MBN), and total dissolved nitrogen [TDN; i.e., dissolved organic nitrogen (DON) and dissolved inorganic nitrogen (DIN)] concentrations in K2SO4 extracts of non-fumigated soil samples were measured (see section 2.5).
2.3. Calculation of contribution of C3 or C4 plant derived to the samples
The contribution of C3 or C4-derived plant to soil C or microbial biomass C was estimated using the measured C-isotope signatures (δ13C) and mass balance approach (Nguyen-Sy et al. Citation2020). The sample (about 50 µg C) was weighed into tin capsules, and δ13C was measured with an on-line C and N analyzer (NC 2500; Thermo Fisher Scientific) coupled with an isotope ratio mass spectrometer (MAT252; Thermo Fisher Scientific). All δ13C values were expressed relative to the international standard Vienna Pee Dee Belemnite (VPDB): δ13C = (Rsample/RVPDB − 1). The standard deviation for four replicate combustions of the same standard within a sequence was less than 0.10‰.
The sample δ13C values depend on a mixture of C3 plant-derived C and C4 plant-derived C, as expressed in the following equation (Nguyen-Sy et al. Citation2020):
where δ13C (sample) is the measured δ13C values of the sample, δ13C (C3) is C3-plant-derived C (av. −27‰), and δ13C value of C4-plant-derived C (av. −12‰). x denotes the proportion of C3 plant-derived C. The proportion of C4 plant-derived C (1 − x) was estimated in the following equation:
2.4. Incorporation of carbon into the heavy soil fraction
To analyze the effects of substrate addition on soil C storage, incorporation of substrate-derived C into the heavy fraction (HF) was determined on days 0, 3, and 21 as follows. Briefly, 10-g aliquots of dried soil were dispersed in sodium iodide solution (NaI, 1.60 g cm−3) and centrifuged at 2,600 × g (modified from Spycher, Sollins, and Rose Citation1983). HFs were recovered after filtering for light fractions.
where HF soil C and C4 plant-derived HF soil C denote soil C in the heavy fraction (mg kg−1 soil) and C4 plant-derived soil C in the heavy fraction (mg kg−1 soil), respectively. The differences in C4 plant-derived HF soil C between the sugarcane-amended and control soils were assumed to be sugarcane-derived in our study. The percentages of sugarcane-derived HF soil C in dose were estimated.
2.5. Soil microbial biomass carbon and nitrogen
The MBC and MBN in the soil samples were determined using the chloroform fumigation-extraction method (Vance, Brookes, and Jenkinson Citation1987). The soluble C and N of the fumigated and non-fumigated soil samples were extracted with 0.5 M K2SO4 (soil to solution ratio of 1:5) and measured using a total organic C analyzer (TOC-V CSH; Shimadzu, Japan). MBC and MBN were estimated using the differences in soluble C and N between the fumigated and non-fumigated soil samples and extraction efficiency coefficients [kEC factor of 0.45 (Wu et al. Citation1990) and a kEN factor of 0.54 (Jenkinson Citation1988), respectively]. The concentrations of DIN, NH4+-N, and NO3−-N in the K2SO4 extracts were determined using a modified indophenol blue method (Rhine et al. Citation1998) and the Griess–Ilosvay method (Mulvaney Citation1996) after cadmium reduction to nitrite.
The δ13C values of freeze-dried K2SO4 extract from the fumigated and non-fumigated soil samples were measured. The δ13C values of microbial biomass C were estimated using the following equation (Ryan and Aravena Citation1994):
where δ13C (fumigate) and δ13C (non fumigate) denote δ13C values (‰) of freeze-dried K2SO4 extract from the fumigated and non-fumigated soil samples, respectively. The Cfumigate and Cnon fumigate denote soluble C concentrations (mg kg−1 soil) of the fumigated and non-fumigated soil samples, respectively. The contribution of C4 plant-derived MBC was calculated in the following equation:
where MBC and C4 plant-derived MBC denote microbial biomass C (mg kg−1 soil) and microbial biomass C derived from C4 plants (mg kg−1 soil). Assuming that differences in C4 plant-derived MBC between the sugarcane-amended and control soils are sugarcane-derived in our study, the percentages of sugarcane-derived MBC in dose were estimated by dividing the differences in C4 plant-derived MBC between the sugarcane-amended and control soils by the respective dose levels [(263 mg C kg−1 soil (level 1), 2,630 mg C kg−1 soil (level 2), and 26,300 mg C kg−1 soil (level 3)]. The fractions of C4-derived C in solution (mg kg−1 soil; non-fumigated 0.5 M K2SO4 extracts) were estimated in the same way:
2.6. Statistics
All results are expressed on an oven-dried (105°C, 24 h) weight basis as the mean ± standard error (SE) of three replicates. Significant differences (p < 0.05) in MBC, MBN, soil solution (soluble C, DON, and DIN), and soil C in the heavy fractions were tested using analysis of variance (ANOVA) for between sampling periods. The analyses were performed using SYSTAT software (ver. 11.0; Systat Software, San Jose, CA, USA).
3. Results
3.1. Characteristics of sugarcane and soil materials used in the incubation
The study soil was sandy loam and poor in organic C and N (). The δ13C values differed significantly between sugarcane materials (sugar, leaf litter, and humic acid) and cassava-cultivated soil (). This supports the precondition of our experiment that sugarcane materials can be traced in incubation studies. The order of C/N ratios followed the order: cane sugar > leaf litter > humic acid (). The soil MBC at the start of incubation dropped to 63 mg C kg−1 soil, which was lower compared to the MBC measured immediately after soil sampling (263 mg C kg−1 soil), due to the substrate limitation in a tropical sandy soil ().
Table 1. Carbon, nitrogen, isotope signature, microbial biomass of substrate, and the sandy soil
Table 2. Fractions of sugarcane-derived carbon added to the soil
3.2. Fate of sugarcane-derived 13C in the soil
As the incubations progressed, sugarcane-derived C was transformed into microbial biomass, soluble C in soil solution, and heavy fractions (). The remaining proportion was composed of CO2 and light fraction, although proportions of these fractions were not measured in our study. As dose level increased, both the δ13C values and the proportion of C4 plant-derived C in MBC, soluble C (solution), and heavy fractions increased (). The differences in C4 plant-derived MBC between the sugarcane-amended and control soils were assumed to be sugarcane-derived in our study ().
Figure 1. The fractions of sugarcane-derived C incorporated into microbial biomass C (MBC), soil solution, and the heavy fractions in the incubated soils. Levels 3, 2, and 1 denote substrate doses 100 times the MBC, 10 times the MBC, and equivalent to the MBC (the field condition), respectively. Bars indicate standard errors (n = 3). Percentage in dose () was plotted
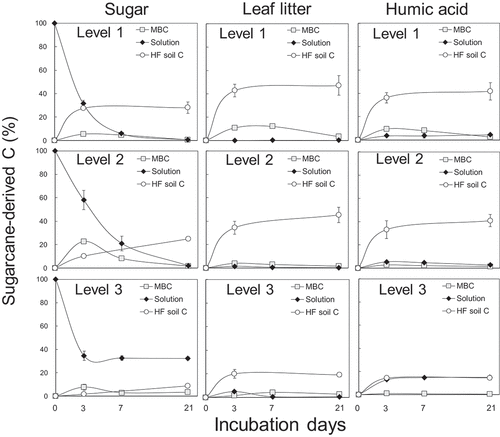
Sugarcane-derived MBC exhibited the peak on 3 days of the incubation in sugar- or humic acid-amended soil samples and after 7 days for leaf litter-amended soil samples (). The microbial biomass declined rapidly after 3 or 7 days in sugar- or humic acid-amended soil, respectively (). On the other hand, there was no significant decrease in microbial biomass between days 7 and 21 in leaf litter-amended soil samples ().
3.3. Nitrogen dynamics in substrate-amended soils
Changes in MBN, DON, and DIN in the incubated soils showed that the forms and amounts of N varied depending on the substrate type and dose level. MBN peaked after 3 days in the sugar-amended and humic acid-amended soils, but MBN dropped sharply after 3 days. An increase in MBN from 0 to 7 days in the sugar-amended soils exceeded the substrate-derived N inputs at dose levels 1 and 2 (), indicating the release of N from the native soil organic N or N mining. By contrast, N in the leaf litter-amended soil was dominated by MBN and DON, and no significant decreases in MBN were observed at 7 or 21 days at dose levels 2 and 3 (). DON was the dominant N fraction in the humic acid-amended soils at dose levels 2 and 3 (). In both the leaf-litter or humic acid-amended soils, an increase in MBN was smaller than the substrate-derived N inputs at all the dose levels ().
Figure 2. The fluctuations in microbial biomass nitrogen (MBN), dissolved organic nitrogen (DON), and dissolved inorganic nitrogen (DIN) in the incubated soils. Levels 3, 2, 1, and 0 denote substrate doses 100 times the MBC, 10 times the MBC, equivalent to the MBC (the field condition), and with no substrate addition (control), respectively. Bars indicate standard errors (n = 3). * indicates significant differences between sampling periods (p < 0.05), n.s. indicates non-significance (ANOVA)
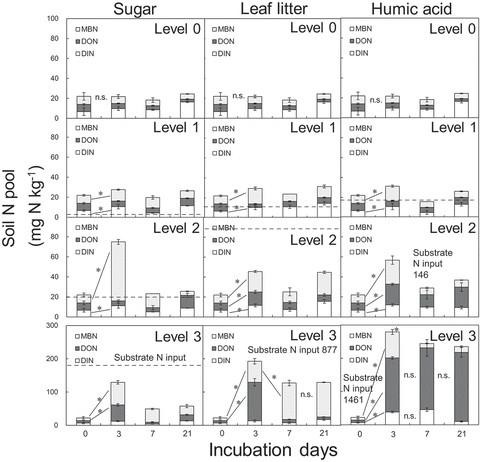
3.4. Carbon storage in substrate-amended soils
A net gain in organic-matter storage was indicated by a significant increase in sugarcane-derived C for substrate-amended soil samples compared with the control (). Nine percent (level 3) to twenty-eight percent (level 1) of sugar remained in the heavy fractions after a 3-week incubation, while 19−47% of leaf litter or 15−42% of humic acid remained in the heavy fractions, respectively (). The storage of sugarcane-derived C in the heavy fractions followed the order: leaf litter > humic acid > sugar (). The efficacy of stabilization of sugarcane-derived C in the heavy fractions decreased with increasing dose levels (; ).
Figure 3. Relationship between sugarcane-derived C in the heavy fraction (HF) incorporated after 3-week incubation. Data were log-transformed. Levels 3, 2, 1, and 0 denote substrate doses 100 times the MBC,10 times the MBC, equivalent to the MBC (the field condition), and with no substrate addition (control), respectively. Bars indicate standard errors (n = 3). * indicates significant differences between substrates (p < 0.05)
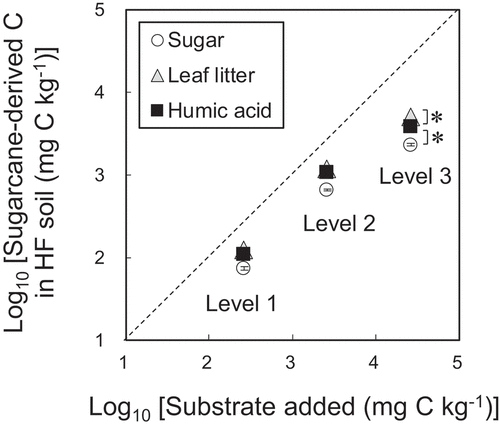
4. Discussion
4.1. Effects of substrate inputs on soil microbial biomass growth and nitrogen mineralization
The microbial biomass (MBC and MBN) increased with increasing substrate inputs (; ). This is consistent with the previous study (Rasul et al. Citation2008). However, the lower proportions of MBC relative to substrate inputs were observed at level 3, compared to level 2 (). The microbial growth is known to reach saturation due to the limitation of the other nutrients (Coody, Sommers, and Nelson Citation1986). In our study, the intrinsically small microbial biomass and low N availability in tropical sandy soils might limit microbial growth under high availability of substrates ().
Substrate types affected both the time lag and magnitude of microbial growth (). The maximum microbial growth comes faster for sugar and humic acid (3 days) than for leaf litter (7 days) (). In the leaf litter-amended soils, enzyme production and solubilization of insoluble leaf litters (mainly cellulose or holocellulose) to sugars are required before microbial substrate uptake (Allison, Wallenstein, and Bradford Citation2010; Hayakawa et al. Citation2014). This leads to the slower microbial growth in the leaf litter-amended soils, compared to the sugar-amended soils in our and previous studies (Sugihara, Funakawa, and Kosaki Citation2010).
Significant N mining in the sugar-amended soils was driven by a sharp increase in microbial biomass, especially at dose level 2 (). Sugar provides abundant labile C relative to N for microbes (). Substrate N limitations on microbial growth induce the release of exoenzymes and promote N mining from the native soil organic matter or priming effects (Chen et al. Citation2014). On the other hand, lower magnitudes of microbial growth and higher N availability in leaf litter and humic acid could limit N mining or priming at dose levels 2 and 3 in our study (; ).
4.2. Effects of substrate types and dose levels on soil organic-matter storage
We hypothesize that soil C storage (heavy fraction) increases with increasing substrate inputs. This was supported by our result (), but the efficacy of C stabilized into the heavy fractions decreased (; ). The concept of C saturation has been proposed for the C-rich soils with the limited capacity to store additional C (Stewart et al. Citation2007). In our study, the maximum storage of substrate-derived C at the dose level 3 (5.4 g kg −1 soil; ) corresponds to about 2 folds of the original soil C concentration (2.5 g kg−1 soil). The C-poor sandy soils would have the limited sorption capacity and aggregate structure to stabilize organic matter (Fujii et al. Citation2017).
Greater storage of C derived from leaf litter and humic acid in the heavy fractions () could be explained by the substrate recalcitrance. This is consistent with the previous study in the sandy soil of northeast Thailand (Puttaso et al. Citation2011), where N-rich and less labile substrates exhibited the higher efficacy of soil C stabilization. Despite a sharp decline in MBC derived from sugar, the gain in heavy fractions was small in our study (). This result differs from the report that microbial necromass and metabolites are important sources of soil C stabilized (Kallenbach, Frey, and Grandy Citation2016). The low contents of clays are considered to limit the incorporation of microbial necromass and metabolites in the heavy fractions in the sandy soils (; ; Hayakawa et al. Citation2018).
4.3. Implication for residue management practices
Although we cannot extrapolate the results obtained under controlled incubation studies to the field C and N dynamics, some findings are applicable to sugarcane-residue management practices. Application of cane sugar is not a common practice, but sugars containing in bud node or stem can affect soil N availability at the early growth stage. The initial flush of microbial growth could increase net soil N mineralization near seed cane (), although the SOC gain is minimal in the sugar-amended soils (). The leaf litter or humic acid amendment could not increase soil N mineralization, judging from the result that there was no N mining at realistic dose levels 2 and 3 ().
Soil C storage in heavy fractions increases with increasing substrate inputs, but the stabilization efficiency of large substrate inputs decreases for leaf litter or humic acid-amended soils (). Soil C loss in sugarcane field is one of the soil degradation issues in northeast Thailand (Yoneyama et al. Citation2006). Dose levels 1 and 2 of leaf litter and humic acid are most efficient for stabilization of sugarcane-derived C into the heavy fractions (). Leaf-litter inputs at these dose levels 1 and 2 correspond to approximately 0.37 Mg C ha−1 and 3.68 Mg C ha−1 in the top 0–10 cm of soil with a bulk density of 1.4 Mg m−3, respectively. The dose level 2 is a reasonable residue input for soil C storage under the field condition, because sugarcane produces a biomass of 30 to 45 Mg C ha−1 yr−1 and residue of 5–8 Mg C ha−1 yr−1, which should be adequate to prevent competition for residues between industry and agriculture. In northeast Thailand, burning is a common practice in the small farm and the case of labor and cane harvester shortage, while green cane trash mulching has an advantage in terms of soil C storage in commercial scale.
The sharp decline in microbial biomass within the first 7 days () suggests that competition for soil N between seedlings and microbes is unlikely for sugar and humic acid. Residue management without burning and tillage can also reduce erosion and weed problems (Carvalho et al. Citation2017), but mulching by plant residues enhances the risk of N competition between plants and microbes. The prolonged microbial N accumulation in leaf litter-amended soils () suggests that soils should be amended with leaf litter for more than 3 weeks, in case the incorporation of residue should cause N starvation (). The increase in microbial N assimilation has lasted for 56 days in the sandy soils with 10 Mg ha−1 residue inputs in northeast Thailand (Vityakon et al. Citation2000).
Assuming that filter-cake humic acid is applied at 2 Mg ha−1, and that C concentration in the humic acid is 23% (), amount of the humic acid-derived C is estimated to be 0.46 Mg C ha−1, which is close to the dose level 1 (0.37 Mg C ha−1). Considering that the density of applied humic acid could be heterogeneous at the field scale, C inputs could approach to the dose level 2 (3.68 Mg C ha−1) in the hot spot. Humic acid inputs corresponding to MBC (level 1) and 10 times the MBC (level 2; 2,630 mg C kg−1 soil or 3.68 Mg C ha−1) efficiently enhance SOC storage and N availability without increasing the risk of competition between plants and microbes (). On the other hand, humic acid inputs corresponding to 100 times the MBC (level 3; 26,300 mg C kg−1 soil or 36.8 Mg C ha−1) are not efficient due to the lower efficacy of C stabilization (; ) and the high risk of DON leaching loss from the sandy soil (). Three substrates (cane sugar, leaf litter, and humic acid) have different advantages and disadvantages in soil C storage and N mineralization of the tropical sandy soil and the designed application could improve soil fertility without increasing conflicts between sectors.
Acknowledgments
We are grateful to thank the Center for Advanced Studies for Agriculture and Food (CASAF), Institute for Advanced Studies, Kasetsart University under Higher Education Research Promotion and National Research University Project of Thailand, Office of the Higher Education Commission, Ministry of Education, and Thailand for the financial support in the student exchange program. This work was also financially supported by a Japan Society for the Promotion of Science grant (No. 20H03120) and JST SICORP Grant Number JPMJSC19C3, Japan.
Disclosure statement
No potential conflict of interest was reported by the authors.
Additional information
Funding
References
- Allison, S. D., M. D. Wallenstein, and M. A. Bradford. 2010. “Soil-carbon response to warming dependent on microbial physiology.” Nature Geosci 3 (5): 336. doi:10.1038/ngeo846.
- Asing, J., N. C. Wong, and S. Lau. 2009. “Optimization of extraction method and characterization of humic acid derived from coals and composts.” J. Trop. Agric. Fd. Sc 37: 211–223.
- Buckeridge, K. M., A. F. La Rosa, K. E. Mason, J. Whitaker, N. P. McNamara, H. K. Grant, and N. J. Ostle. 2020. “Sticky dead microbes: rapid abiotic retention of microbial necromass in soil.” Soil Biol. Biochem 149: 107929. doi:10.1016/j.soilbio.2020.107929.
- Carvalho, J. L. N., R. C. Nogueirol, L. M. S. Menandro, R. D. O. Bordonal, C. D. Borges, H. Cantarella, and H. C. J. Franco. 2017. “Agronomic and environmental implications of sugarcane straw removal: a major review.” Gcb Bioener 9 (7): 1181–1195. doi:10.1111/gcbb.12410.
- Chen, R., M. Senbayram, S. Blagodatsky, O. Myachina, K. Dittert, X. Lin, E. Blagodatskaya, and Y. Kuzyakov. 2014. “Soil C and N availability determine the priming effect: microbial n mining and stoichiometric decomposition theories.” Glob. Chan. Biol 20 (7): 2356–2367. doi:10.1111/gcb.12475.
- Coody, P. N., L. E. Sommers, and D. W. Nelson. 1986. “Kinetics of glucose uptake by soil microorganisms.” Soil Biol. Biochem 18 (3): 283–289. doi:10.1016/0038-0717(86)90062-3.
- De Oliveira Bordonal, R., L. M. S. Menandro, L. C. Barbosa, R. Lal, D. M. B. P. Milori, O. T. Kolln, and J. L. N. Carvalho. 2018. “Sugarcane yield and soil carbon response to straw removal in South-central Brazil.” Geoderma 328: 79–90. doi:10.1016/j.geoderma.2018.05.003.
- Fujii, K., C. Hayakawa, T. Panitkasate, I. Maskhao, S. Funakawa, T. Kosaki, and E. Nawata. 2017. “Acidification and buffering mechanisms of tropical sandy soil in Northeast Thailand.” Soil Tillage Res 165: 80–87. doi:10.1016/j.still.2016.07.008.
- Hayakawa, C., K. Fujii, S. Funakawa, and T. Kosaki. 2018. “Effects of sorption on biodegradation of low-molecular-weight organic acids in highly-weathered tropical soils.” Geoderma 324: 109–118. doi:10.1016/j.geoderma.2018.03.014.
- Hayakawa, C., S. Funakawa, K. Fujii, A. Kadono, and T. Kosaki. 2014. “Effects of climatic and soil properties on cellulose decomposition rates in temperate and tropical forests.” Biol. Fertil. Soils 50 (4): 633–643. doi:10.1007/s00374-013-0885-4.
- Jenkinson, D. S. 1988. “Determination of microbial biomass carbon and nitrogen in soil.” In Advances in Nitrogen Cycling in Agricultural Ecosystems, edited by J. R. Wilson, 368–386. Wallingford, UK: CAB International.
- Kallenbach, C. M., S. D. Frey, and A. S. Grandy. 2016. “Direct evidence for microbial-derived soil organic matter formation and its ecophysiological controls.” Nature Commun 7 (1): 13630. doi:10.1038/ncomms13630.
- Kuzyakov, Y. 2002. “Review: Factors Affecting Rhizosphere Priming Effects.” J. Plant Nutr. Soil Sci 165: 382–396.
- Leal, M. R. L., M. V. Galdos, F. V. Scarpare, J. E. Seabra, A. Walter, and C. O. Oliveira. 2013. “Sugarcane straw availability, quality, recovery and energy use: a literature review.” Biomass Bioener 53: 11–19. doi:10.1016/j.biombioe.2013.03.007.
- Mulvaney, R. L. 1996. “Nitrogen–inorganic forms. in methods of soil analysis.” In Soil Science Society of America, edited by S. DL, 1123–1184. Madison, WI: USA.
- Nguyen-Sy, T., W. Cheng, S. M. Kimani, H. Shiono, R. Sugawara, K. Tawaraya, T. Watanabe, and K. Kumagai. 2020. “Stable carbon isotope ratios of water-extractable organic carbon affected by application of rice straw and rice straw compost during a long-term rice experiment in Yamagata, Japan.” Soil Science and Plant Nutrition 149 (1): 125–132. doi:10.1080/00380768.2019.1708209.
- Öhlinger, R. 1995. “Maximum Water-holding Capacity.” In Methods in Soil Biology, edited by F. Schinner, R. Öhlinger, E. Kandeler, and R. Margesin, 385–386. Berlin: Springer.
- Puttaso, A., P. Vityakon, P. Saenjan, V. Trelo-Ges, and G. Cadisch. 2011. “Relationship between residue quality, decomposition patterns, and soil organic matter accumulation in a tropical sandy soil after 13 Years.” Nutr. Cycl.Agroecosys 89 (2): 159–174. doi:10.1007/s10705-010-9385-1.
- Rasul, G., K. S. Khan, T. Müller, and R. G. Joergensen. 2008. “Soil microbial response to sugarcane filter cake and biogenic waste compost.” J. Plant Nutr. Soil Sci 171 (3): 355–360. doi:10.1002/jpln.200700094.
- Rhine, E. D., G. K. Sims, R. L. Mulvaney, and E. J. Pratt. 1998. “Improving the berthelot reaction for determining ammonium in soil extracts and water.” Soil Sci Soc Am 62 (2): 473–480. doi:10.2136/sssaj1998.03615995006200020026x.
- Ryan, M. C., and R. Aravena. 1994. “Combining 13C natural abundance and fumigation-extraction methods to investigate soil microbial biomass turnover.” Soil Biology & Biochemistry 26 (11): 1583–1585. doi:10.1016/0038-0717(94)90101-5.
- Schimel, J. P., and J. Bennett. 2004. “Nitrogen mineralization: challenges of a changing paradigm.” Ecology 85 (3): 591–602. doi:10.1890/03-8002.
- Schimel, J. P., and M. N. Weintraub. 2003. “The implications of exoenzyme activity on microbial carbon and nitrogen limitation in soil: a theoretical model.” Soil Biol. Biochem 35 (4): 549–563. doi:10.1016/S0038-0717(03)00015-4.
- Schneckenberger, K., D. Dermin, K. Stahr, and Y. Kuzyakov. 2008. “Microbial Utilization and Mineralization of [14 c]glucose added in six orders of concentration to soil.” Soil Biol. Biochem 40 (8): 1981–1988. doi:10.1016/j.soilbio.2008.02.020.
- Spycher, G., P. Sollins, and S. Rose. 1983. “Carbon and nitrogen in the light fraction of a forest soil: vertical distribution and seasonal patterns.” Soil Sci. 135 (2): 79–87. doi:10.1097/00010694-198302000-00002.
- Stewart, C. E., K. Paustian, R. T. Conant, A. F. Plante, and J. Six. 2007. “Soil Carbon Saturation: Concept, Evidence and Evaluation.” Biogeochemistry 86 (1): 19–31. doi:10.1007/s10533-007-9140-0.
- Sugihara, S., S. Funakawa, and T. Kosaki. 2010. “In situ short-term carbon and nitrogen dynamics in relation to microbial dynamics after a simulated rainfall in croplands of different soil texture in Thailand.” Soil Sci. Plant Nutr 56 (6): 813–823. doi:10.1111/j.1747-0765.2010.00516.x.
- Vance, E. D., P. C. Brookes, and D. S. Jenkinson. 1987. “An extraction method for measuring soil microbial biomass C.” Soil Biol. Biochem 19 (6): 703–707. doi:10.1016/0038-0717(87)90052-6.
- Vityakon, P., S. Meepech, G. Cadisch, and B. Toomsan. 2000. “Soil organic matter and nitrogen transformation mediated by plant residues of different qualities in sandy acid upland and paddy soils.” NJAS-Wageningen J. Life Sci 48 (1): 75–90. doi:10.1016/S1573-5214(00)80006-8.
- Wu, J., R. G. Joergensen, B. Pommerening, R. Chaussod, and P. C. Brookes. 1990. “Measurement of soil microbial biomass c by fumigation extraction—an automated procedure.” Soil Biol. Biochem 22 (8): 1167–1169. doi:10.1016/0038-0717(90)90046-3.
- Yoneyama, T., H. Okada, P. Chongpraditnum, S. Ando, P. Prasertsak, and K. Hirai. 2006. “Effects of Vegetation and Cultivation on δ 13C Values of Soil Organic Carbon and Estimation of Its Turnover in Asian Tropics: A Case Study in Thailand.” Soil Sci. Plant Nutr 52 (1): 95–102. doi:10.1111/j.1747-0765.2006.00001.x.