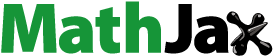
ABSTRACT
Electrolytes have been verified as a vital internal factor controlling soil aggregate stability (SAS). Several reports have focused on whether potassium promotes or demotes SAS and have shown contradictory results in different soils and clay mineralogy. Only a few studies have investigated such an effect on a soil-plant system, even though this information is critical for soil management practices to maintain soil structural stability. This research studied the dynamics of SAS as influenced by potassium in a soil-plant system. Corn as a test plant was grown on Haplic Acrisols (Typic Paleustults) in a greenhouse. The potassium adsorption ratio (PAR), cation ratio of structural stability (CROSS), and SAS were recorded on the day before planting (day 0), the day of planting (day 1), days 10, 20, 30, and the day of harvesting (day 55) at the tasseling stage. The corn growth, biomass, and potassium uptake were also examined. The relationship between soil and plant parameters was statistically tested. The results showed the existence of SAS dynamics caused by soil potassium change, with agreement among the responses for PAR, CROSS, and plant uptake. Within 24 h of soil treatment with potassium, the SAS move rapidly from 74% to 96%. It remained steady at 96–97% when the corn was in the vegetative stage and then fell significantly (α = 0.05) to 88% when the corn was in the tasseling stage, where the potassium uptake was maximized. The results implied that SAS is a sensitive index of soil physical quality associated with potassium, especially for tropical kaolinitic soils.
1. Introduction
Soil aggregate stability (SAS) is defined as the ability of the soil to uphold the structure based on its solid components and its pore space when exposed to a disruptive force field derived from either natural or human-made sources (Dexter Citation1988; Kay Citation1990; Amézketa Citation1999; Huang, Li, and Sumner Citation2011). In general, SAS controls the geometry and continuity of soil pores, which influence the fate and transport of mass and energy in soils interfacing with agriculture and the environment (Herrick et al. Citation2001). Therefore, it is profoundly used as a useful index of soil physical quality in determining the status of soil structure and erosion risk (Barthes and Roose Citation2002; Bronick and Lal Citation2005; Moncada et al. Citation2013; Collins, Stone, and Leonard Citation2015).
It has been well established that SAS is derived from particle-particle interaction (Hu et al. Citation2015; Xu, Yu, and Li Citation2015). Generally, clay to clay bonding is mediated by exchangeable cations, especially high valency cations such as magnesium and calcium (Hu et al. Citation2018). This action is caused by a favorable condition between positive-charged cations and negative-charged clay surfaces, leading to their mutual attraction (Rengasamy and Marchuk Citation2011). However, such a concept could not be used to explain mono-valency cations such as potassium and sodium are adsorbed on negative charges of clay-sized particles, resulting in clay swelling, dispersion, and, eventually, soil aggregate degradation. This phenomenon is probably one of the reasons why there are still debates in the literature on whether potassium demotes or promotes soil structure. Therefore, it is challenging to categorize potassium as either a clay-flocculating or clay-dispersing cation unless the attraction/repulsion energy of clay particles in the presence of potassium is specified.
Potassium application has been proven to benefit crop yields and their quality (Arienzo et al. Citation2009). In addition, potassium has also been investigated as a dispersal inducer similar to sodium (Rengasamy and Sumner Citation1998; Rengasamy Citation2002; Smiles and Smith Citation2004; Smiles Citation2006). Rengasamy and Marchuk (Citation2011) defined a new ratio, named the cation ratio of soil structural stability (CROSS), which improved the sodium adsorption ratio by incorporating the dispersive effects of potassium and sodium with respect to the flocculating impact of calcium and magnesium. However, Hu et al. (Citation2015) revealed that increasing potassium in soil solutions decreased the clay surface potential, reducing the repulsion energy between adjacent clay particles, and further improving soil aggregate strength. Others (Holthusen, Peth, and Horn Citation2010) reported on the improvement of soil microstructural stability as influenced by potassium using the rheological approach where shear stress, storage modulus, and loss modulus of clays were considered with successive potassium concentrations.
More recently, potassium has been proven to have a significant promotion of clay flocculation and aggregate stability in tropical kaolinitic soils without plants (Phocharoen et al. Citation2018). They reported that potassium improved SAS of Typic Paleustults (Haplic Acrisols) even after the soil had equilibrated with KCl at 0.025 M for 24 h. Nevertheless, such an effect within a soil-plant system, critical for managing cultivated soils to maintain their physical quality, is limited. Here, we conducted follow-up experiments to determine SAS dynamics driven by potassium in a soil-plant system. We hypothesized that there is a decrease in SAS due to the potassium consumed by the plant, where the response to potassium is the most sensitive when the plant uptake is approaching a maximum, that is, at the tasseling stage in corn.
2. Materials and methods
2.1. Experimental approach
Our approach was to grow corn as a test plant on a kaolinitic tropical soil with adequate potassium and other nutrients. SAS, PAR, and CROSS were analyzed at different times: the day before planting (day 0), the day of planting (day 1), day 10, day 20, day 30, and the day of harvesting (day 55) where the corn was in the tasseling stage. Corn growth parameters, including shoot height and perimeter, were recorded from days 10 to 55. Simultaneously, the total weight of fresh and dry samples and the potassium concentration and uptake were examined from the plant samples harvested on day 55. The relationship between the soil and plant parameters was then statistically tested. Based on the potassium taken up by the corn in the soil-plant system, the SAS dynamics were also verified.
2.2. Soil and its analysis before planting
The soil sample was collected in the Ap horizon (0–30 cm), indicated by the dark color boundary of organic matter accumulation, in a corn-cassava field managed on a rotational basis in Nakhon Ratchasima province, Northeastern Thailand. This soil was classified as Typic Paleustults using the USDA soil taxonomy (Soil Survey Staff Citation2014b) and Haplic Acrisols according to WRB (IUSS Working Group WRB Citation2015), and was locally called the Warin (Wn) soil series. The soil was entirely mixed as a composite sample, air-dried and sieved to a size smaller than 2 mm for fundamental analyses such as texture using a pipette method (Gee and Bauder Citation1986), pH using a pH meter (National Soil Survey Center Citation1996), effective electrical conductivity (ECe) using an electric conductometer (soil:H2O; 1:5) (Talsma Citation1968), cation exchange capacity (CEC) using the ammonium acetate saturation method (Chapman Citation1965; National Soil Survey Center Citation1996), organic matter using Walkley and Black titration (Walkley and Black Citation1934) for organic carbon and multiplied by 1.72, available phosphorus by Bray-II method (Kuo Citation1996), available potassium and exchangeable calcium, magnesium and sodium using the ammonium acetate method (Chapman Citation1965; Brown and Warnke Citation1988), and sulfate using the turbidimetric method (Wall, Gehrke, and Suzuki Citation1980). For trace elements, diethylenetriaminepentaacetic acid (DTPA) extraction (Lindsay and Norvell Citation1978) was used. These soil properties are summarized in .
Table 1. Soil properties of the studied soil before greenhouse experiment
2.3. Greenhouse experiment
We intended to observe the dynamics of SAS caused by potassium taken up by the corn. Therefore, a large amount of soil per pot is needed for the measurement frequency of the soil parameters, requiring approximately 200 g in each period from the same pot throughout the experiment. We conducted the greenhouse experiment from the initial step (day 0) to harvest (day 55). ) shows the schematic diagram of the experimental setup. ) displays a plan view of the pot, showing the soil samples were taken from different circular positions in the same pot. The activity schedule for each period is presented in .
Table 2. Activity schedule for measurements of soil and plant parameters
Figure 1. Schematic diagram of experimental approach of pot experiment from the day of soil preparation with potassium (day 0) to the day of harvesting at tasseling stage (day 55) of corn (a) along with pot details and soil sampling position (b). PAR = potassium adsorption ratio; CROSS = cation ratio of structural stability; SAS = soil aggregate stability
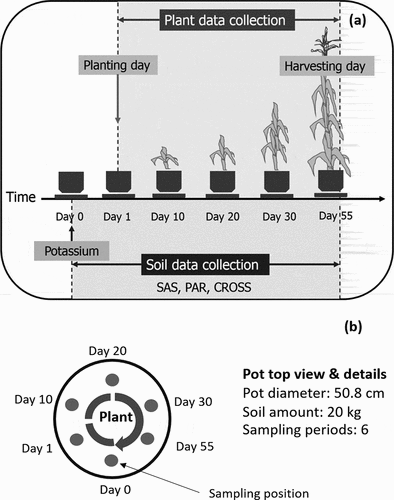
On day 0, the air-dried soil was thoroughly mixed and separately collected for the measurement of the initial soil parameters. Then, the 20 kg of soil were placed into a pot with a diameter of 50.8 cm in three replications. The fertilizer applications based on the previous soil nutrient analysis () were then added at the rates of 146 mg N kg−1, 18 mg P2O5 kg−1, and 49 mg K2O kg−1 to provide the adequate levels recommended for corn growth (Thailand Department of Agriculture Citation2005). The three pots were placed on trays containing the same level of deionized water to allow the soil to moisturize from the bottom based on capillary action, which minimized watering disturbance of the soil surface.
After equilibrating with water and fertilizers, including potassium, for 24 h. On day 1, the soil sampling was done for the measurement. Then, corn seeds (Zea mays L., hybrid var. Suwan 4452) were grown in each pot. On days 10, 20, and 30, soil and corn growth parameters were recorded. On day 55, the corn was in the tasseling stage, with assumed maximum uptake of nutrients (Aramrak et al. Citation2007). Besides collecting the soil and plant data, the aerial parts of corn were harvested, weighed, and oven-dried at 70 °C to a constant weight. The dry plant samples were recorded as the biomass and taken for digestion in an aqua regia mixture, then analyzed for potassium using an atomic absorption spectrophotometer (AA 240, Agilent Technologies, Santa Clara, USA), and used to calculate the total potassium uptake. It should be noted that there was only one level of potassium applied as fertilizer based on the recommended rate following the soil analysis for corn grown in this pot experiment.
2.4. Soil aggregate stability
The greenhouse schedule involved the soil samples being air-dried, sieved to a diameter of 1–2 mm, and gradually moisturized to field capacity. We determined SAS based on Kemper and Rosenau (Citation1986) with further modifications by Phocharoen et al. (Citation2018) using a wet sieving apparatus with a mesh size of 250 μm at a speed of 35 rpm and a vertical distance of 1.3 cm for 3 min (Eijkelkamp, Thai Victory, Bangkok, Thailand). To exclude the mass of the sand particle, plant residues, and organic matter, a high-intensity ultrasonic processor (VCX 750, Becthai Bangkok Equipment & Chemical, Bangkok, Thailand) was used to disperse the stable aggregates which remained after the wet-sieving. Therefore, the fraction of dry aggregates with a diameter of 1–2 mm were separated into stable and unstable parts using wet-sieving for 250 μm. After drying the soil samples in an oven at 105 °C, SAS was calculated using EquationEquation (1)(1)
(1) :
where %SAS is the soil aggregate stability as a percentage, S is the dry weight of the stable parts, and U is the dry weight of the unstable parts that passed through the sieve hole after wet sieving.
2.5. Soil PAR and CROSS
Using the same soil samples as for the SAS analysis, the soils were saturated with a soil paste (Soil Survey Staff Citation2014a). Their filtrates were extracted to measure the amounts of exchangeable K+, Na+, Mg2+, and Ca2+ using the atomic absorption spectrophotometer. These exchangeable bases (mmolc L−1) were used to calculate the potassium adsorption ratio (PAR) according to Laurenson et al. (Citation2011) and cation ratio of structural stability (CROSS) based on Rengasamy and Marchuk (Citation2011), as shown in EquationEquations (2)(2)
(2) and (Equation3
(3)
(3) ), respectively:
2.6. Data analysis
The SAS data as a function of time, PAR, CROSS, and plant growth were tested using analysis of variance (ANOVA) in the R software package. The mean comparisons were determined at a significance level of α = 0.05 using LSD only if the F-value was significant. The SAS dynamics based on potassium changed due to corn uptake in the soil-plant system were functionally derived using the Solver add-on to the Microsoft Excel software package.
3. Results
3.1. Soil aggregate stability
The SAS data changed throughout the corn growth period (). On day 0 at the start of the experiment, the SAS was 74%. There was a significant (p < 0.05) and rapid increase in SAS from 74% on day 0 to 96% on day 1, but only after introducing potassium for 24 h. SAS remained consistently within a narrow range of 96–97% during days 1–30. During this period, the corn was in the vegetative growth stage with significant changes in height (from 5.1 cm to 29.0 cm) and width (from 1.7 cm to 5.3 cm), as shown in . On day 55, when the corn was in the tasseling stage and it was assumed that it had reached maximum potassium uptake (1.7 g K kg−1), SAS declined to 88% ().
Table 3. Soil aggregation parameters influenced by potassium (mean ± SD) and plant response
3.2. Soil PAR and CROSS
) show soil PAR and CROSS, respectively, throughout the period of plant growth. Overall, the results demonstrated that PAR and CROSS sharply increased after the potassium application but declined when the corn started growing, which was in agreement with the SAS results. PAR was 0.14 (mmolc L−1)0.5 at first, then rose to 0.19 (mmolc L−1)0.5 on day 1 and reached a maximum of 0.30 (mmolc L−1)0.5 on day 10. However, on day 20, PAR decreased substantially to 0.20 (mmolc L−1)0.5, then further diminished to 0.15 (mmolc L−1)0.5. Additionally, CROSS had a similar trend to that of PAR. Nevertheless, the added potassium caused an increase in CROSS that was at a higher order of magnitude than for PAR, ranging from 1.26 to 3.84 (mmolc L−1)0.5.
3.3. SAS as a function of PAR and CROSS
In this study, we aimed to identify whether the change in potassium uptake by a test plant corresponded with the potassium in the soil aggregate and its subsequent effects on SAS. We then used the PAR data to evaluate the presence of K+ in the soil aggregates considering Ca2+ and Mg2+. In contrast, the CROSS data were included to investigate whether decreasing the K+ in soil aggregates would reduce SAS in the soil-plant system.
Regardless of the collecting day, the PAR and CROSS data were plotted versus SAS and fitted. The results showed that the PAR data varied according to plant uptake and SAS was fitted to a non-linear function with an inverse exponential rise to a maximum with a very high coefficient of determination (R2 = 0.993), as shown in ). PAR measured 0.19 (mmolc L−1)0.5 on day 1 when SAS in the soil-plant system reached a plateau of 96%. The CROSS data also fitted very well with an inverse exponential curve rising to a maximum but with a lower R2 value of 0.865 ()). The CROSS value was 2.65 (mmolc L−1)0.5 when the SAS value also started to be consistent at 96% on day 1.
4. Discussion
We recognized that the effects of potassium change on SAS might be confounded by nitrogen and phosphorus during fertilizer application. However, the major basic cations generally involved in SAS in a similar mechanism are calcium, magnesium, potassium, and sodium (Amézketa Citation1999). Based on the strong and positive influence of potassium on SAS in the same soil as reported by Phocharoen et al. (Citation2018), the SAS data which directly interfered with nitrogen and phosphorus fertilizers would be minimal or negligible in this experiment. With our experimental approach (), the SAS value that was determined together with potassium indices (PAR and CROSS) across the period of plant growth elucidated the SAS dynamics derived by potassium alternation under a soil-plant system. Based on the potassium uptake by the corn, the change in SAS revealed that SAS is a sensitive index of soil physical quality associated with the chemical fertility of potassium.
The initial SAS at day 0 was 74% (), classified as having high stability, corresponding with the medium level of potassium, according to Moncada et al. (Citation2013) (). This soil was collected using the same procedure as described in Phocharoen et al. (Citation2018), yet its potassium level was relatively higher in our case due to the fertilizer application practice at the time of soil sampling. As a consequence of the previous management practice, the initial variation in potassium can explain the SAS at day 0. In our study, there was a higher SAS value (74%) with the initial potassium level being 71 mg kg−1, compared with those reported by Phocharoen et al. (Citation2018), where the SAS was 45% with a lower potassium level (35 mg kg−1). In our case, the SAS level increased sharply to 96% after introducing potassium within 24 h before planting the corn seeds. Consequently, the increase in SAS was a rapid response, possibly due to clay flocculation enhanced by potassium (Phocharoen et al. Citation2018), a vital prerequisite for soil aggregation and stability (Dexter Citation1988; Amézketa Citation1999).
During the corn growth on days 1–30 (), SAS was steady but suddenly decreased to 88% on day 55, where the corn was at the tasseling stage. The decrease in SAS could most likely be explained by the firming of the repulsion between adjacent particles developed when the test plant reached maximum potassium uptake. We accepted that a range of the measurement frequency did not cover the SAS data between days 30 to 55 and whether the decrease was gradual or abrupt. We chose this range because the amount of soil remaining in the pot decreased with each successive period involving SAS measurement, thereby requiring that the number of soil samples taken should be minimized in order to not impact plant growth. With our six periods from days 0–55, the total soil sample taken from each pot was about 1.2 kg out of 20 kg from the initial soil provided, thus maintaining enough soil for corn growth sustained throughout the experiment.
SAS as a function of PAR in the soil-plant system in ) could best be described as having an inverse exponential rise to a maximum (R2 = 0.993), agreeing well with the batch experiment in the laboratory with similar soil (R2 = 0.994) as reported by Phocharoen et al. (Citation2018). Without a test plant and the initial SAS of 45% in their investigation, the critical PAR that consistently maintained SAS of at least 88% was 1.9 (mmolc L−1)0.5 based on potassium saturation at the rate of 0.025 M. In our plant test experiment and with an initial SAS of 74%, the critical PAR was much lower (as low as 0.19 (mmolc L−1)0.5) derived from the potassium fertilizer application at the rate of 49 mg K2O kg−1 soil ( and ). These two experiments used different approaches and amounts of potassium, explaining the difference of PAR data by one order. In the same soil, at a higher range of PAR (0.1–5.0 (mmolc L−1)0.5) as reported by Phocharoen et al. (Citation2018) versus the lower range of PAR (0.15–0.30 (mmolc L−1)0.5)) in our experiment, we noticed that the change in PAR could explain SAS variation in both experiments. These results confirmed that SAS is highly sensitive to soil potassium change either through an increase resulting from potassium application or a decrease due to plant intake.
SAS changes induced by CROSS fluctuation also existed () and )) in the soil-plant system. The CROSS increase induced by potassium did not decrease SAS in the current work. Our results suggested that CROSS from the viewpoint of potassium having a similar negative impact as sodium on soil structural stability (Rengasamy and Sumner Citation1998; Rengasamy Citation2002; Smiles and Smith Citation2004; Smiles Citation2006; Rengasamy and Marchuk Citation2011) may not be conclusive for tropical-kaolinitic soils with plant growth.
According to the CROSS concept, an increase in CROSS would result in soil structure failure. Emami et al. (Citation2014) studied the change in CROSS caused by calcium, sodium, and potassium derived from different soil conditions under a soil-plant system. They found that potassium addition induced an increase in CROSS significantly and negatively correlated with structural stability indicators such as the mean weight diameter, S-index, and water-dispersive clay. It should be noted that no determination was made on the repulsion energy of the clay particles as affected by the different soil conditions in their study.
Hu et al. (Citation2015) tested the relationship between the particle interaction energy of potassium and the adjacent clay particles extracted from the studied soils. They found that increasing the potassium concentration caused a decrease in surface potentials, thereby reducing the repulsion energy, further improving the breaking strength of clay domains, and enhancing soil aggregates. With the same range (0–0.1 M) of potassium concentration applied to soil samples, Phocharoen et al. (Citation2018) experimented on the influence of potassium on SAS. They found that an increase in PAR and CROSS as influenced by potassium application resulted in greater stability of soil aggregates compared to the control treatment with no additional K. They also reported that a degree of the positive response of SAS due to the potassium depended on soil chemical properties and their clay mineralogy.
In the present study, we assumed that the maximum uptake of potassium was at the tasseling stage (55 days after planting), according to Aramrak et al. (Citation2007). However, the potassium concentration in the plant tissues in our experiment was about 1.7 g K kg−1 dry biomass or 0.17%, which was much lower than that reported by Halvin et al. (Citation2014), where the critical concentration of potassium was 3–5% at the earlier stage of corn (24–45 days after planting). This result suggested that even with a lower amount of potassium removal in the crop, the SAS had already significantly deteriorated due to the potassium dynamics. Consequently, we speculated that SAS would be much lower than 88% if the corn were harvested at its physical maturity under field conditions.
5. Conclusions
The soil aggregate stability dynamics within a soil-plant system relied on potassium obtained from both a fertilizer application and plant intake. The change in soil aggregate stability due to potassium uptake by a test plant confirmed that the potassium affected soil aggregate stability in a temporary and manageable way. Therefore, a regular potassium application should compensate for the potassium removed by the crop, based on soil analysis, which would maintain high aggregate stability (>90%), even for tropical kaolinitic soils with medium texture and low cation exchange capacity. Our findings also implied that soil aggregate stability is a sensitive index of the soil physical quality associated with potassium fertility.
Acknowledgments
Special thanks go to Mr. Yotsapon Phocharoen, Miss Apichaya Thammaroongroj, and Miss Thanyaporn Panawong for their substantial assistance in collecting soil and plant data.
Disclosure statement
No potential conflict of interest was reported by the author(s).
Additional information
Funding
References
- Amézketa, E. 1999. “Soil Aggregate Stability: A Review.” Journal of Sustainable Agriculture 14: 83–151. doi:10.1300/J064v14n02_08.
- Aramrak, S., J. Chanchareonsook, C. Suwannarat, and C. Sarobol. 2007. “Assessment of Multielement Extractants for Prediction of Available Potassium in Thai Soils.” Kasetsart Journal (Natural Science) 41 (3): 461–466.
- Arienzo, M., E. W. Christen, W. Quayle, and A. Kumar. 2009. “A Review of the Fate of Potassium in the Soil-plant System after Land Application of Wastewaters.” Journal of Hazardous Materials 164: 415–422. doi:10.1016/j.jhazmar.2008.08.095.
- Barthes, B., and E. Roose. 2002. “Aggregate Stability as an Indicator of Soil Susceptibility to Runoff and Erosion; Validation at Several Levels.” Catena 47: 133–149. doi:10.1016/S0341-8162(01)00180-1.
- Bronick, C. J., and R. Lal. 2005. “Soil Structure and Management: A Review.” Geoderma 124: 3–22. doi:10.1016/j.geoderma.2004.03.005.
- Brown, J. R., and D. Warnke. 1988. “Recommended Cation Tests and Measures of Cation Exchange Capacity.” In Recommended Chemical Soil Test Procedures for North Central Region, edited by W. C. Dahnke, 15–16. Fargo: North Dakota Agricultural Association.
- Chapman, H. D. 1965. “Cation Exchange Capacity.” In Methods of Soil Analysis. Part 1. Physical and Mineralogical Methods, edited by A. Klute, 891–913. Madison (WI): American Society of Agronomy.
- Collins, C. D., J. J. Stone, and I. C. Leonard. 2015. “Runoff and Sediment Yield Relationships with Soil Aggregate Stability for a State-and-transition Model in Southeastern Arizona.” Journal of Arid Environments 117: 96–103. doi:10.1016/j.jaridenv.2015.02.016.
- Dexter, A. R. 1988. “Advances in Characterization of Soil Structure.” Soil and Tillage Research 11: 199–238. doi:10.1016/0167-1987(88)90002-5.
- Emami, H., A. R. Astaraei, A. Fotovat, and M. Khotabaei. 2014. “Effect of Soil Conditioners on Cation Ratio of Soil Structural Stability, Structural Stability Indicators in a Sodic Soil, and on Dry Weight of Maize.” Arid Land Research and Management 28: 325–339. doi:10.1080/15324982.2013.856357.
- Gee, G. W., and J. W. Bauder. 1986. “Particle-size Analysis.” In Methods of Soil Analysis. Part 1. Physical and Mineralogical Methods, edited by A. Klute, 383–409. Madison (WI): American Society of Agronomy.
- Halvin, J. L., S. L. Tisdale, W. L. Nelson, and J. D. Beaton. 2014. Soil Fertility and Fertilizers-An Introduction to Nutrient Management. 8th ed. City (NJ): Pearson Education .
- Herrick, J. E., W. G. Whitford, A. G. De Soyza, J. W. Van Zee, K. M. Havstad, C. A. Seybold, and M. Walton. 2001. “Field Soil Aggregate Stability Kit for Soil Quality and Rangeland Health Evaluations.” Catena 44: 27–35. doi:10.1016/S0341-8162(00)00173-9.
- Holthusen, D., S. Peth, and R. Horn. 2010. “Impact of Potassium Concentration and Matric Potential on Soil Stability Derived from Theological Parameters.” Soil and Tillage Research 111: 75–85. doi:10.1016/j.still.2010.08.002.
- Hu, F. N., C. Y. Xu, H. Li, S. Li, Z. H. Yu, Y. Li, and X. H. He. 2015. “Particle Interaction Forces and Their Effects on Soil Aggregates Breakdown.” Soil and Tillage Research 147: 1–9. doi:10.1016/j.still.2014.11.006.
- Hu, F. N., J. F. Liu, C. Y. Xu, Z. L. Wang, G. Liu, H. Li, and S. W. Zhao. 2018. “Soil Internal Forces Initiate Aggregate Breakdown and Splash Erosion.” Geoderma 320 (26): 43–51. doi:10.1016/j.geoderma.2018.01.019.
- Huang, P. M., Y. Li, and M. E. Sumner. 2011. Handbook of Soil Sciences Properties and Processes. 2nd ed. Boca Raton (FL): CRC Press.
- IUSS Working Group WRB. 2015. “World Reference Base for Soil Resources 2014, Update 2015 International Soil Classification System for Naming Soils and Creating Legends for Soil Maps.” World Soil Resources Reports No. 106. Rome: FAO.
- Kay, B. D. 1990. “Rates of Change of Soil Structure under Different Cropping Systems.” In Advances in Soil Science 12, edited by B. A. Stewart, 1–52. New York (NY): Springer.
- Kemper, W. D., and R. C. Rosenau. 1986. “Aggregate Stability and Size Distribution.” In Methods of Soil Analysis. Part 1. Physical and Mineralogical Methods, edited by A. Klute, 425–442. Madison (WI): American Society of Agronomy.
- Kuo, S. 1996. “Phosphorus.” In Methods of Soil Analysis. Part 3. Chemical Methods, edited by D. L. Sparks, 869–919. Madison (WI): American Society of Agronomy.
- Land Classification Division and FAO Project Staff. 1973. Soil Interpretation Handbook for Thailand. Bangkok, Thailand: Department of Land Development. Ministry of Agriculture Cooperatives.
- Laurenson, S., N. S. Bolan, E. Smith, and M. McCarthy. 2011. “Soil Structural Changes following Irrigation with a Potassium Rich Winery Wastewater.” Paper presented at the 24th Annual FLRC Workshop, Palmerston North, New Zealand: Massey University, February 9.
- Lindsay, W. L., and W. A. Norvell. 1978. “Development of a DTPA Soil Test for Zn, Fe, Mn and Cu.” Soil Science Society of America Journal 42: 421–428. doi:10.2136/sssaj1978.03615995004200030009x.
- Moncada, M. P., D. Gabriels, W. Cornelis, and D. Lobo. 2013. “Comparing Aggregate Stability Tests for Soil Physical Quality Indicators.” Land Degradation and Development 26 (8): 843–852. doi:10.1002/ldr.2225.
- National Soil Survey Center. 1996. Soil Survey Laboratory Methods Manual. Washington (DC): United States Department of Agriculture.
- Phocharoen, Y., S. Aramrak, N. Chittamart, and W. Wisawapipat. 2018. “Potassium Influence on Soil Aggregate Stability.” Communications in Soil Science and Plant Analysis 49 (17): 2162–2174. doi:10.1080/00103624.2018.1499752.
- Rengasamy, P., and M. E. Sumner. 1998. “Processes Involved in Sodic Behaviour.” In Sodic Soils: Distribution, Properties, Management, and Environmental Consequences, edited by M. E. Sumner and R. Naidu, 35–50. New York (NY): Oxford University Press.
- Rengasamy, P. 2002. “Clay Dispersion.” In Soil Physical Measurement and Interpretation for Land Evaluation, edited by B. M. McKenzie, K. Coughlan, and H. Cresswell, 200–210. Collingwood (VIC) Australia: Commonwealth Scientific and Industrial Research Organisation.
- Rengasamy, P., and A. Marchuk. 2011. “Cation Ratio of Soil Structural Stability (CROSS).” Soil Research 49 (3): 280–285. doi:10.1071/SR10105.
- Smiles, D. E. 2006. “Sodium and Potassium in Soils of the Murray-Darling Basin: A Note.” Australian Journal of Soil Research 44: 727–730. doi:10.1071/SR06057.
- Smiles, D. E., and C. J. Smith. 2004. “A Survey of the Cation Content of Piggery Effluents and Some Consequences of Their Use to Irrigate Soils.” Australian Journal of Soil Research 42 (2): 231–246. doi:10.1071/SR03059.
- Soil Survey Division Staff. 1993. Soil Survey Manual. Washington (DC): United States Department of Agriculture.
- Soil Survey Staff. 2014a. Kellogg Soil Survey Laboratory Methods Manual. Lincoln (NE): United States Department of Agriculture.
- Soil Survey Staff. 2014b. Keys to Soil Taxonomy. 12th ed. Washington (DC): United States Department of Agriculture.
- Talsma, T. 1968. “Soil Salinity.” In Environmental Studies of the Coleambally Irrigation Area and Surrounding District, edited by S. Pels, M. Stannard, and T. Talsma, 35–48. City (NSW): Water Conservation and Irrigation Commission.
- Thailand Department of Agriculture. 2005. “Fertilizer Recommendations for Economic Crops.” Thailand Department of Agriculture. Bangkok, Thailand: Thailand Ministry of Agriculture and Cooperatives.
- United States Salinity Laboratory Staff. 1954. Diagnosis and Improvement of Saline and Alkaline Soil. Washington (DC): United States Department of Agriculture Handbook 60.
- Walkley, A., and I. A. Black. 1934. “An Examination of Degtjareff Method for Determining Soil Organic Matter and a Proposed Modification of the Chronic Acid Titration Method.” Soil Science 37: 29–38. doi:10.1097/00010694-193401000-00003.
- Wall, L. L., C. W. Gehrke, and J. Suzuki. 1980. “An Automated Turbidimetric Method for Total Sulfur in Plant Tissue and Sulfate Sulfur in Soils.” Communications in Soil Science and Plant Analysis 11 (11): 1087–1103. doi:10.1080/00103628009367107.
- Xu, C. Y., Z. H. Yu, and H. Li. 2015. “The Coupling Effects of Electric Field and Clay Mineralogy on Clay Aggregate Stability.” Journal of Soils and Sediments 15 (5): 1159–1168. doi:10.1007/s11368-015-1063-0.