ABSTRACT
Salt-affected reclaimed tidelands (RTLs) in coastal areas of South Korea are commonly used for rice (Oryza sativa L.) cultivation as excessive salts are removed through leaching by irrigation during rice growth. However, changes in soil properties and rice growth in RTLs by continuous rice cultivation are not well understood. In the present study, we investigated soil salinity indices (ECe, electrical conductivity of soil extracts; SAR, sodium adsorption ratio; ESP, exchangeable sodium percentage), fertility (mineral nitrogen (N), and available phosphorus (P) concentrations), and carbon (C) content as well as rice biomass and yield in 72 paddy fields within 10 RTL areas, which have been cultivated for different time periods (13–35 years). Continuous rice cultivation decreased ECe by 0.36 dS m−1 per year, indicating desalinization, but ESP and SAR were not changed. Available P concentration increased but mineral N concentration did not change with rice cultivation years. There was also an indication of increased soil organic C (SOC) concentration with rice cultivation. However, rice biomass and yield were not different among the RTLs. Instead, rice biomass and yield as well as SOC concentration were negatively correlated with ECe when all individual measurements from 72 sampling sites within the 10 RTLs were pooled, suggesting that salinity is a constraint for both rice production and SOC sequestration at field scales. Interestingly, soil mineral N concentration was positively correlated with ECe but negatively with rice biomass and yield. This result implies that heavy application of N fertilizer in highly saline soils may not benefit rice growth due to salinity stress. Our results show that long-term rice cultivation with fertilization and irrigation decreases salinity while increasing P availability. However, SOC content and rice biomass and yield were more responsive to the salinity status of each paddy field than during cultivation years, highlighting the need for field-specific soil management for improved rice production and enhanced SOC sequestration.
1. Introduction
Sustainable management of cropland soils is critical not only for food production but also to mitigate greenhouse gas emissions through soil carbon (C) sequestration under climate change (Lal Citation2004). However, soil quality of cropland is frequently degraded through soil erosion, contamination, desertification, and salinization; and among those, soil salinization is one of the typical land degradation processes, affecting about 20% of global cropland (FAO (Food and Agriculture Organization of the United Nations) Citation2017; Edrisi et al. Citation2021). Saline soils are mostly developed in arid and semi-arid regions where evaporation is higher than rainfall, and thus salts derived from irrigation and fertilization are rarely removed through leaching (Singh Citation2015). However, even in humid climatic zones, high salinity of cropland soils developed in coastal areas threatens sustainable food production (Lim et al. Citation2020).
In South Korea, reclaimed tidelands (RTLs) have been constructed in coastal areas since the 1980s to meet increasing social demand for food security, and currently RTLs account for 11.7% of the total cropland area (MOAF (Ministry of Agriculture, Food and Rural Affairs) Citation2019). RTL soils are not favorable for the growth of most crops due to not only high salinity but also low soil fertility and organic matter, poor soil physical properties, and high groundwater levels (Jeong et al. Citation2020; Lim et al. Citation2020). In spite of such unfavorable conditions, RTLs in South Korea are widely used for rice (Oryza sativa L.) cultivation under waterlogged conditions with excessive fertilization (Lim et al. Citation2020). Rice plant is not salt tolerant and rice yield starts to decrease when the electrical conductivity of soil saturated extracts (ECe) is over 3.0 dS m−1 (Maas and Hoffman Citation1977; Ayers and Westcot Citation1985; Francois and Maas Citation1999). However, leaching of excessive salts during rice growth via periodic irrigation of less saline water alleviates salinity stress for rice (Abrol, Yadav, and Massoud Citation1988), leading to rice yields comparable to those in non-saline soils (Lim et al. Citation2020).
Due to intensive agricultural management, such as irrigation, fertilization, and organic matter inputs, long-term cultivation of rice may change soil properties including salinity, fertility, and C content, as well as rice yield in RTLs (Lim et al. Citation2020). A few studies have investigated the changes in properties of paddy RTL soils under controlled experimental conditions with the aim to remediate saline soil properties by application of gypsum (Kim, Choo, and Cho Citation2017) and compost (Moon et al. Citation2011). However, there are few studies on the changes in soil properties, including C content, and rice yield of RTLs with different history of rice cultivation under conventional farmers’ agricultural practices. Understanding such variations in rice yield together with changes in soil properties under different histories of rice cultivation would advance our knowledge on the effects of long-term rice cultivation on desalinization and associated changes in the properties of saline soils (Shi et al. Citation2005). This information would further help to develop better agricultural management practices for RTLs soils to improve rice production as well as enhance C sequestration (Lim et al. Citation2020).
This study was conducted to investigate the variability of soil salinity and fertility, and their relationships with C content and rice yield of coastal RTLs soils under different rice cultivation period after the construction of RTLs. We hypothesized that 1) with increasing years of rice cultivation, soil salinity would decrease due to desalinization by irrigation and leaching of salts during rice growth, while soil fertility and C content may increase as a result of repeated application of fertilizer and organic matter inputs (including rice residue) and 2) rice yields may be higher in RTLs with longer rice cultivation history compared with those in RTLs with shorter cultivation history due to more enhanced desalinization as well as improved soil properties.
2. Materials and methods
2.1. Study sites
The RTLs in South Korea comprise 186,639 ha, which is equivalent to 11.7% of the total arable land and responsible for 22.1% of the national rice production. There are a total of 91 districts of RTLs in South Korea. In the present study, 10 RTLs located in southwestern coastal areas were selected based on accessibility to the sampling sites, geographical location, and the area and the year of construction ( and ). The 10 RTLs were Bojeon (BJ), Goheung (GH), Gunnae (GN), Mandeog (MD), Samsan (SS), Sanae (SN), Seosimcheon (SC), Unnam (UN), Wolcheon (WC), and Youngsangang (YS). The study area has a typical humid temperate climate with a monsoon period in the summer. The meteorological data for each RTL site were not available, but the annual mean temperature and annual precipitation in the meteorological station at Mokpo which is located within 70 km from the RTL sites, were 13.6°C and 1430 mm, respectively, during the last three decades (KMA (Korea Meteorological Administration) Citation2018). The initial soil organic C (SOC) contents before the start of rice cultivation in the study sites are not available; however, soil survey data reported that the typical soil series (Hasa, Yeompo, Gwanghwal, and Munpo series) of RTL soils had lower SOC (6.0‒9.2 g C kg−1) compared to soil series (Mangyuong, Sachon, Yongji, and Jeonbug series) of non-RTL soils (14.4‒21.2 g C kg−1) (RDA Citation2000).
Table 1. Information of 10 reclaimed tidelands (RTLs) investigated in this study
Figure 1. Geographical locations of reclaimed tideland (RTL) investigated in this study. Detailed information including the code names, reclaimed area, cultivation age, and the number of sampling paddy fields for each RTL are provided in Table 1.
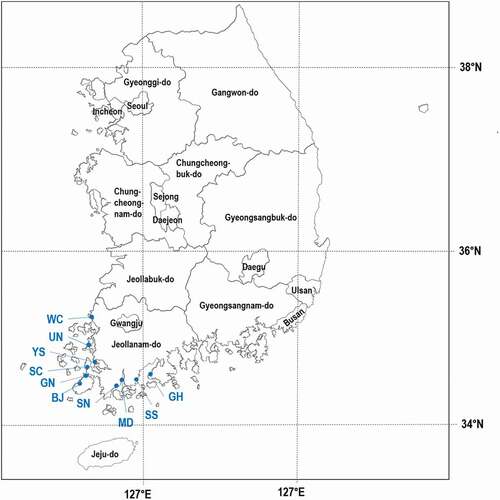
The area of the RTLs ranged from 238 to 20,249 ha, and rice has been cultivated for 13‒34 years after the construction of the RTLs (). According to the farmers’ interview, N-P-K compound fertilizers (N+ P2O5+ K2O) were applied at 272 (128, 64, and 81 kg ha−1 for N, P2O5, and K2O, respectively) to 605 (284, 142, and 180 kg ha−1, respectively) kg ha−1, together with cattle manure compost at 200‒250 kg ha−1. The N application rates in RTLs were higher than the standard rates for non-RTLs paddies (90‒110 kg N ha−1, depending on soil fertility) recommended by the Korean government (RDA (Rural Development Administration) Citation2017).
2.2. Sampling and analyses
In each RTL, 3 to 12 paddy fields (72 fields in total) were randomly selected (). The number of sampling fields was determined based on the area of RTLs, except for SC that has a narrow and long shape and needed more samples to account for the higher site heterogeneity. In March 2018, before the start of rice cultivation, surface mineral soil (0–20 cm) samples were collected from three randomly selected points in each paddy field and composited to represent each paddy field. In this study, we collected soil samples before fertilization for rice cultivation of the year to investigate the cumulative residual effects of long-term rice cultivation practices by avoiding the effects of current year’s fertilization. Therefore, the fertility of the soils reflects long-term rather than the current year’s fertilization.
The soil samples were air-dried, passed through a 2-mm sieve, and used for analyses. Particle size distributions were determined using the pipette method (Gee and Bauder Citation1986), pHe and ECe were measured using a conductivity meter (Orion 3 STAR, Thermo Fisher Scientific Korea, Seoul, Korea) after extracting solutions from saturated pastes of the soils (Rhoades Citation1996) using a vacuum extractor (SampleTek 24VE, MAVCO INDUSTRIES, Inc., Kentucky, USA). Soil organic C content was determined using the Walkley–Black method after removing chloride ions by adding Ag2SO4 (Nelson and Sommers Citation1982). Cation exchange capacity (CEC) of the soils were determined with the ammonium acetate method (pH 7.0) (Sumner and Miller Citation1996). Exchangeable Na+ concentration of the ammonium acetate extracts was analyzed with an atomic absorption spectrometer (Analyst 800, Perkin Elmer, Waltahm, USA) to determine the exchangeable sodium percentage (ESP) (Rhoades Citation1996). Soluble cation (Na+, Ca2+, and Mg2+) concentrations of the saturated paste extracts were also determined with the atomic absorption spectrometer to determine sodium adsorption ratio (SAR) (Rhoades Citation1996). The concentrations of soil mineral nitrogen (N) (i.e., NH4+ and NO3−) were determined with indophenol method (Mulvaney Citation1996) and vanadium reduction method (Doane and Horwáth Citation2003), respectively, after extracting mineral N using 2 mol L−1 KCl at 1-to-5 (soil-to-extract) ratio. Available phosphorus (P) concentrations of the soils were determined with Bray #2 methods (Kuo Citation1996).
At harvest in October 2018, three randomly selected sampling plots (1 m2) were established in the same paddy fields where soil samples were collected, and the rice plants within the plots were cut at 3 cm above the ground. Rice samples were washed with running tap water and air-dried in a greenhouse for 2 weeks. The total biomass and grain yield of the rice were determined after drying the pre-dried samples at 65°C for 2 days. The harvest index was calculated as the ratios of rice grain yield to total above-ground biomass.
2.3. Statistical analysis
Data were tested for normality of the distribution and homogeneity of variance using Shapiro-Wilk and Levene’s tests, respectively. Data transformation was not needed since no heterogeneity was detected and the distribution was normal. The difference between soil physicochemical variables and rice biomass and yield across the 10 RTLs were assessed by analysis of variance (ANOVA) using a General Linear Model. When their difference was significant, the means were separated by Duncan’s multiple-range test. The effects of rice cultivation period on soil properties and rice biomass and yield were also assessed by regression analyses. Inter-correlation between soil variables and the effects of soil variables on rice biomass and yield were explored by regression analyses in two ways: 1) using averaged data of each paddy field in the RTL (n = 10) to investigate the inter-RTL variations and 2) using individual measurements from 72 sampling fields of the 10 RTLs (n = 72) to explore inter-field variations. Multiple regression analyses were conducted to determine the relevant contributions of soil properties to rice biomass and yield. All statistical analyses were performed using the IBM SPSS Statistics 23 (IBM Corp., Armonk, New York, USA) and the level of significance for all statistical tests was set α =0.05.
3. Results
3.1. Properties of RTL soils
All soil physicochemical properties () as well as salinity characteristics () investigated in the present study differed among the 10 RTLs. Among the soil variables, pHe, ECe and soil available P concentration were correlated with rice cultivation period (). In accordance with our hypothesis, with increasing cultivation age, the soil pHe (range: 6.9 ‒ 8.2) and ECe (14.9 ‒ 2.4 dS m−1) decreased, and the available P concentration (2.3–36.7 mg P kg−1) increased. There is also an indication (P = 0.062) of an increased SOC concentration (6.6 ‒ 12.8 g C kg−1) with increases in cultivation period. Other soil variables were not correlated with cultivation period (data not shown).
Table 2. Physico-chemical properties of the reclaimed tideland (RTL) soils
Table 3. Salinity characteristics of the reclaimed tideland (RTL) soils
Figure 2. Relationship between rice cultivation period and soil variables: (a) pH of saturated paste extracts (pHe), (b) electrical conductivity of saturated paste extracts (ECe), (c) soil organic carbon (SOC) concentration, and (d) available soil P concentration. Values are the means of replicates and vertical bars are standard errors of the means. The number replicated fields are provided in Table 1.
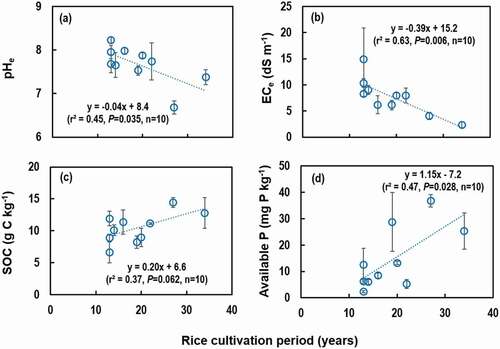
When inter-correlation of soil variables (n = 72) across 10 RTLs were explored, there was a significant correlation (r2 = 0.24, P < 0.001) between ECe and SOC concentration (); the SOC concentration increased with decreasing ECe. Soil mineral N () was positively correlated with ECe (r2 = 0.23, P < 0.001), and available P () was negatively correlated with ECe (r2 = 0.14, P = 0.001). The correlation between each soil variable and ECe was stronger (greater r2 values) when the average values of each RTL were used, compared with when all individual field data were used in the correlation though statistical significance (the P-values) were lowered due to the decreased number of data points when averaged (n = 10) (). Any other soil variables, which were not mentioned above, were not correlated with each other (data not shown).
Figure 3. Relationship between electrical conductivity of saturated paste extracts (ECe) and other soil variables across 10 RTLs: (a) and (b) soil organic carbon (SOC) concentration, (c) and (d) mineral N (NH4+ + NO3−) concentration, and (e) and (f) available P concentration. Single data values pooled across all sampling sites in all 10 RTLs (n = 72) were used for (a), (c), and (e), and averaged values of each RTL (n = 10) were used for (b), (d), and (f), in which standard errors of the means of soil variables were indicated by horizontal bars.
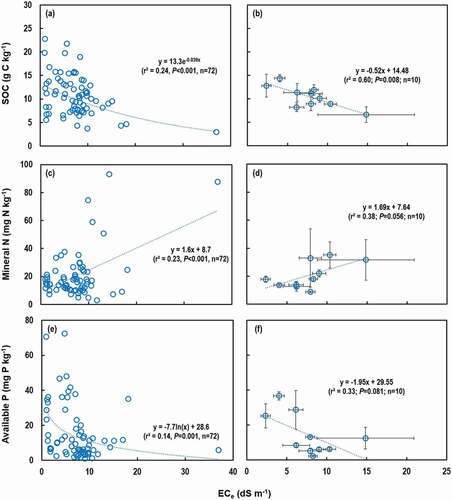
3.2. Rice biomass and yield, and their relationship with soil properties
The average above-ground biomass, grain yield, and harvest index of rice plants varied widely from 977 to 1792 g m−2, from 208 to 925 g m−2, and from 0.18 to 0.51, respectively, across the RTLs (). Neither biomass nor grain yield of rice was correlated with cultivation period (), and thus our hypothesis was rejected. However, both the biomass (r2 = 0.44, P < 0.001) and yield (r2 = 0.35, P < 0.001) were negatively correlated with ECe when the relationship between ECe and rice biomass and yield was explored using the whole data set (n = 72) across the 10 RTLs (). A similar pattern was also found when averaged data were used though statistical significance (P-values) decreased (r2 = 0.54, P = 0.016 for biomass and r2 = 0.37, P = 0.062 for yield) (). The relationship between harvest index and cultivation year or ECe were similar to those of biomass and yield; harvest index was not correlated with cultivation period () but was negatively correlated with ECe (r2 = 0.24, P < 0.001 for the whole data set and r2 = 0.21, P = 0.184 for the averaged data, data not shown). Interestingly, the rice biomass (r2 = 0.26, P < 0.001) and yield (r2 = 0.37, P < 0.001) were also negatively correlated with the mineral N concentrations (), but there was no relationship with the available P concentrations (data not shown). According to the multiple regression analyses, ECe negatively contributed to rice biomass (β = ‒0.594, P < 0.001) or yield (β = ‒0.384, P = 0.007), and there was also indication of negative contribution of NO3− concentration to rice yield (β = ‒0.249, P = 0.065), while contributions of other soil properties were negligible ().
Table 4. Above-ground biomass and grain yield of rice plants in the reclaimed tideland (RTL) soils
Table 5. Multiple regression analysis of rice biomass and yield with soil variables
Figure 4. Relationship between rice cultivation period and (a) above-ground rice biomass, (b) rice grain yield, and (c) harvest index. Values are the means of replicates and vertical bars are standard errors of the means. The number replicated fields are provided in Table 1.
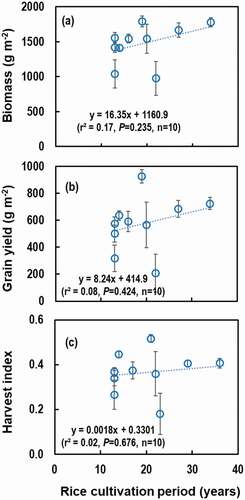
Figure 5. Relationship between soil variables and rice growth (above-ground biomass and grain yield) across 10 RTLs: (a) and (b) between electrical conductivity of saturated paste extracts (ECe) and rice biomass and yield, and (c) and (d) between mineral N (NH4+ + NO3−) concentration and rice biomass and yield. Single data values pooled across all sampling sites in all 10 RTLs (n = 72) were used for (a) and (c), and averaged values of each RTL (n = 10) were used for (b) and (d), in which standard errors of the means of soil variables were indicated by horizontal bars. In (a), 50% rice yield loss (508 g m−2) occurred at ECe of 7.4 dS m−1 as indicated by the black dotted line.
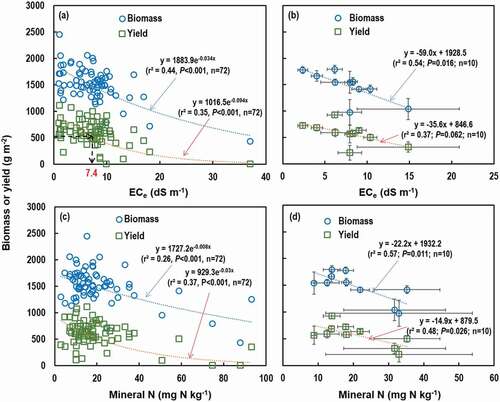
4. Discussion
4.1. Soil salinity and fertility
Our results showed that continuous rice cultivation decreased soil pHe () and ECe () though ESP and SAR did not change. As mentioned earlier, such decreases in salinity and pH with rice cultivation period should that reflect desalinization via salt leaching by irrigation of less saline water during the rice cultivation period (Guo et al. Citation2015; Lim et al. Citation2020; Xu et al. Citation2020). It has also been reported that tillage breaks soil clods and crystalized salts, which facilitates leaching of salts (Nam and Kwon Citation1999; Pearce et al. Citation1999; Wilson et al. Citation2000). The regression equation (; ECe = −0.36×[cultivation period] + 14.9) for the relationship between rice cultivation period and ECe further suggested that it may take approximately 30 years to decrease soil ECe from 14.9 dS m−1 (y-intercept of the equation) to <4.0 dS m−1, which is the threshold ECe for the classification of saline soils (Horneck et al. Citation2007). However, it is cautioned that this estimation would differ with field conditions such as soil properties (e.g., macropores and texture) and agricultural practices (i.e., irrigation and tillage intensities) that affect salt leaching (Guo et al. Citation2015; Lim et al. Citation2020).
While salinity decreased, continuous rice cultivation increased available P concentration () but did not change mineral N concentration. Such different patterns between N and P in responses to rice cultivation reflect the difference in the mobility and loss potential of N and P in the soils. It is well understood that mineral N is susceptible to loss via NH3 volatilization, leaching, and denitrification (Choi et al. Citation2020); whereas P is easily immobilized in the soils through sorption onto clay particles and precipitation with metal ions (Nishigaki et al. Citation2018). In a previous study, we found that leaching of NO3− following active nitrification is a major pathway of N loss in RTL soils (Kwak et al. Citation2008). The increased available P concentration of soils with rice cultivation () as well as the negative correlation between soil ECe and available P concentration () could be attributed to accumulation of available P in the soils under long-term rice cultivation with fertilization and compost application (Ando et al. Citation2021). In the present study, the concentrations of available P (2.3‒36.7 mg P kg−1) were much lower than those (114.4 ± 15.9 mg P kg−1) in non-RTL paddy soils in South Korea (Lim et al. Citation2020) and were also below the lower limits of the optimum level (35‒52 mg P kg−1) of available P concentration recommended by the Korean government (RDA (Rural Development Administration) Citation2017). Therefore, P may be necessary to be supplemented for sustainable rice production.
Unlike the pattern of available P, mineral N concentrations were positively correlated with ECe () though high salinity did not necessarily increase mineral N concentration. Instead, this pattern might reflect heavy fertilization by farmers to increase rice growth in more saline soils or in early period of rice cultivation. The lack of stimulative effect of increased mineral N concentration on rice biomass and yield () contradicts to other studies (Kim et al. Citation2011, Citation2012) that reported that increased N fertilization from 140 kg N ha−1 to 200 kg N ha−1 improved rice yield by 5‒10%. However, in these studies (Kim et al. Citation2011, Citation2012), the experiments were conducted using less saline soils with ECe between 0.75 and 2.5 dS m−1 compared to the soils in the present study. Therefore, our results together with the findings of Kim et al. (Citation2011), Kim et al. (Citation2012), it could be suggested that heavy N fertilization may not improve rice production in highly saline soils.
4.2. Soil organic C content
Though statistically not significant (P = 0.062), rice cultivation tended to increase SOC concentration (), probably reflecting organic matter inputs such as rice residue and compost (Lim et al. Citation2020). In the studied RTLs, rice straw was often removed from the fields for use in livestock farming sectors such as cattle forage and bedding materials (RDA (Rural Development Administration) Citation2019). However, rice root biomass, which comprises about 34% of the total rice residue biomass in South Korea (Kim et al. Citation2009), might directly contribute to increased SOC concentration. In a previous study, we found that 5‒30% of rice root was decomposed in a 60-day incubation experiment, indicating that at least 70% of rice root remains annually though the decomposition rate might vary with soil conditions and periods (Park et al. Citation2020). Under saline and waterlogged conditions, roots might be decomposed more slowly compared to the conditions of non-saline and aerobic conditions due to salinity stress and O2 limitation (Elmajdoub and Marschner Citation2015). In addition, rice straw, which was removed from the field after harvest, is eventually returned to the field as cattle manure compost, and thus could serve as another C source. It has been reported that application of manure compost is efficient in increasing SOC in rice paddies as compost contains recalcitrant C, and organic C decomposition is slow under waterlogged anaerobic conditions compared to upland soils (Sahrawat Citation2004; Rui and Zhang Citation2010; Lim et al. Citation2017). Therefore, it could be postulated that returns of rice straw as manure composts and annual incorporation of rice root residues into soils might contribute to the increased SOC contents of RTL soils. The negative relationship between ECe and SOC concentration () in combination with the negative relationship between ECe and rice biomass () also implies that increased rice biomass with decreasing soil salinity might directly contribute to the increased SOC in RTLs with longer rice cultivation history.
Despite increased SOC concentration with rice cultivation, the SOC concentrations (6.6 ‒ 14.4 g C kg−1) of the studied RTLs were lower than those (14.8 ± 0.3 g C kg−1) of non-RTL paddy soils reported in South Korea (Lim et al. Citation2020) as well as the optimum SOC levels recommended by the Korean government (11.6–17.4 g C kg−1) (RDA (Rural Development Administration) Citation2017). Therefore, in the view of SOC sequestration, RTL soils are considered to have a greater potential of SOC storage compared with non-saline paddy soils, though relevant studies are still lacking (Lim et al. Citation2020). Recently, inorganic C sequestration through carbonate precipitation of CO2 with divalent cations such as Ca2+ and Mg2+ in soils are receiving special interests (Beerling et al. Citation2020). As carbonate precipitation reactions are facilitated by high pH and the presence of divalent cations (Wong et al. Citation2010), saline soils with inherently high pH and divalent cation concentrations may favor inorganic C sequestration (Lim et al. Citation2012). Therefore, further studies to investigate the potential of RTL soils to form carbonate precipitation may be helpful in understanding soil C dynamics including both organic and inorganic C under high pH and salinity for developing soil C sequestration strategies in RTL soils.
4.3. Rice biomass and yield
Though neither rice biomass nor yield was correlated with the rice cultivation period (), the negative correlations between ECe and rice biomass and yield () suggest that rice growth is inhibited by soil salinity. Harvest indices were within the range (0.17‒0.56) for many rice cultivars reported in the previous studies (Bueno and Lafarge Citation2009; Ju et al. Citation2009). Though rice is a major crop cultivated in the saline RTLs, rice is known to be sensitive to salinity stress, particularly during seedling and reproductive stages (Bernstein Citation1964; Heenan, Lewin, and McCaffery Citation1988; Zeng, Shannon, and Lesch Citation2001). For example, Maas and Hoffman (Citation1977) reported that rice yield started to decrease at ECe of 3 dS m−1 and 50% yield loss occurred at ECe between 6 and 7 dS m−1. Meanwhile, Rashed, Khlifa, and Fahmy (Citation2003) reported that the threshold ECe was 6 dS m−1 and 50% yield loss was found at ECe around 20 dS m−1. Different magnitude of rice yield loss by salinity stress could be ascribed to different rice cultivation conditions including soil types, cultivars, and water management (Lim et al. Citation2020). In the present study, 50% rice yield loss (508 g m−2) occurred at ECe of 7.4 dS m−1 (), and this result coincides with the findings of Maas and Hoffman (Citation1977). The predominant impact of ECe on rice growth over other soil variables was also confirmed by multiple regression analysis between rice growth and soil variables ().
The wide variations in rice biomass and yield within a narrow ECe range () further suggest that rice growth may be affected not only by osmotic stress resulting from total ion concentrations (measured as ECe) but also by specific ion species including sodium (Na+), chloride (Cl−), boron (B), and sulfate (SO42-) (Lim et al. Citation2020) though the impacts of these specific ions on rice growth were not assessed in the present study. A high Na+ concentration suppresses potassium (K+) uptake (Nemati et al. Citation2011) and Cl− has toxicity, which causes wilting (Philip and Broadley Citation2001). Boron may also hamper rice growth by inhibiting tillering (Ochiai et al. Citation2008) and hydrogen sulfide (H2S) produced from SO42- under anaerobic conditions inhibits root respiration (Gao, Tanji, and Scardaci Citation2003). In addition, it was also experimentally proven that cultivation management including N fertilization, gypsum application, organic amendments, irrigation water salinity, irrigation interval, and drainage may also affect rice biomass and yield as summarized by Lim et al. (Citation2020).
However, in the present study, it was not possible to assess the effects of these factors on rice biomass and yield due to lack of relevant information. Nevertheless, a lack of correlation of rice biomass and yield with SOC, SAR, and ESP (data not shown) in the present study implies that the positive effects of relevant cultivation management practices (e.g., organic amendments and gypsum application) on rice growth are not evident under field conditions where other complicated constraints exist. It is also interesting to see the negative correlation between mineral N concentration and rice biomass and yield (). As N is the most important nutrient for plant growth, this result was not expected. Though many studies (Back et al. Citation2005; Kim et al. Citation2011, Citation2012; Choudhary and Yaduvanshi Citation2016) reported that increased N application rate enhanced rice yield in saline soils, our results indicates that heavy application of N fertilizer in high saline soils may not improve rice growth due to other constraints including salinity. Although the casual relationship between fertilizer N application and rice growth is not clear, rice plants might have not uptake N efficiently due to poor root growth under highly saline conditions (Abdelgadir, Oka, and Fujiyama Citation2005), resulting in accumulation of residual N derived from fertilizer and soil N mineralization. Soil pH can also affect rice plant uptake of N and thus mineral N concentration of saline soils (Huang et al. Citation2017). In a pot experiment using soils with different salinity (2, 6, and 10 dS m−1) and pH (7, 8, and 9), Huang et al. (Citation2017) found that increased N application could improve rice growth even under highly saline conditions (6 and 10 dS m−1) when soil pH was 7, but did not benefit N uptake and rice growth when pH was 8 and 9 regardless of salinity levels. In the present study, though the impact of soil pH on rice growth was not evident (), most RTL soils had pH > 7.0 (), which might have collaborated with high EC to decrease rice uptake of N.
5. Conclusions
Our results show that long-term rice cultivation with fertilization and irrigation significantly decreased soil salinity, while available soil P concentration increased. There was also an indication of increased SOC content by continuous rice cultivation. However, such changes in soil properties under long-term rice cultivation did not translate into increased rice biomass and yield. Instead, the negative relationship between ECe and SOC content and between ECe and rice biomass and yield across the fields from 10 RTLs suggested that not only rice cultivation years but also field-specific rice cultivation practices might affect desalinization and associated changes in soil properties as well as rice biomass and yield. This in turn implies that the adverse impact of soil salinity on rice growth could be alleviated by proper rice cultivation practices. Literature has suggested that N fertilization, gypsum application, organic amendments, frequent irrigation with less saline water, and drainage are effective in increasing rice growth, though relevant information is not available in the present study. In the context of soil N management, our results indicated that poor rice growth under highly saline conditions might have decreased rice plant uptake of mineral N, resulting in a high mineral N concentration of soils. Therefore, our study highlights the necessity of further studies on the relationship among N fertilization rates, salinity, and rice yield to find an optimum N management including fertilization rate for both improved rice production and enhanced SOC sequestration in coastal RTL soils.
Acknowledgments
This work was carried out with the support of the “Cooperative Research Program of Agriculture Science and Technology Development (Project No. PJ0138732021),” Rural Development Administration, Republic of Korea. This study was also supported by research funds provided to newly appointed professors (J.H. Kwak) of Jeonbuk National University in 2019.
Disclosure statement
No potential conflict of interest was reported by the author(s).
Additional information
Funding
References
- Abdelgadir, E. M., M. Oka, and H. Fujiyama. 2005. “Nitrogen Nutrition of Rice Plants under Salinity.” Biologia Plantarum 49 (1): 99‒104. doi:https://doi.org/10.1007/s10535-005-0104-8.
- Abrol, I. P., J. S. P. Yadav, and F. I. Massoud. 1988. Salt-affected Soils and Their Management. FAO Soils Bulletin, 39. Rome, Itally: Food and Agriculture Organization of the United Nations.
- Ando, K., N. Yamaguchi, Y. Nakamura, M. Kasuya, and K. Taki. 2021. “Speciation of Phosphorus Accumulated in Fertilized Cropland of Aichi Prefecture in Japan with Different Soil Properties by Sequential Chemical Extraction and P K-edge XANES.” Soil Science and Plant Nutrition. doi:https://doi.org/10.1080/00380768.2021.1874249.
- Ayers, R. S., and D. W. Westcot. 1985. Water Quality for Agriculture. FAO Irrigation and Drainage Paper 29 Rev. 1. Rome, Italy: Food and Agriculture Organization of the United Nations.
- Back, N. H., W. Y. Cho, J. C. Ko, J. K. Nam, H. K. Park, J. I. Choung, S. S. Kim, and K. G. Park. 2005. “Proper Nitrogen Fertilizer Level for Improving the Rice Quality at Reclaimed Saline Land in the Southwestern Area.” Korean Journal of Medicinal Crop Science 50: 46‒50. (In Korean).
- Beerling, D. J., E. P. Kantzas, M. R. Lomas, P. Wade, R. M. Eufrasio, P. Renforth, B. Sarkar. 2020. “Potential for Large-Scale CO2 Removal via Enhanced Rock Weathering with Croplands.” Nature 583:242‒248. doi:https://doi.org/10.1038/s41586-020-2448-9.
- Bernstein, L. 1964. Salt Tolerance of Plants. USDA Information Bull, 283. Washington, D.C.: USDA.
- Bueno, C. S., and T. Lafarge. 2009. “Higher Crop Performance of Rice Hybrids than of Elite Inbreds in the Tropics. 1. Hybrids Accumulate More Biomass during Each Phenological Phase.” Field Crops Research 112: 229‒237. doi:https://doi.org/10.1016/j.fcr.2009.03.006.
- Choi, W. J., J. H. Kwak, H. J. Park, H. I. Yang, S. I. Park, Z. Xu, S. M. Lee, S. S. Lee, and S. X. Chang. 2020. “Land-Use Type, and Land Management and Disturbance Affect Soil δ15N: A Review.” Journal of Soils and Sediments 20: 3283‒3299. doi:https://doi.org/10.1007/s11368-020-02708-x.
- Choudhary, O. P., and N. P. S. Yaduvanshi. 2016. “Nutrient Management in Salt-affected Soils.” Indian Journal of Fertilizer 12: 20‒35.
- Doane, T., and W. R. Horwáth. 2003. “Spectrophotometric Determination of Nitrate with a Single Reagent.” Analytical Letters 36 (12): 2713‒2722. doi:https://doi.org/10.1081/AL-120024647.
- Edrisi, S. A., V. Tripathi, R. K. Chaturvedi, D. K. Dubey, G. Patel, and P. C. Abhilash. 2021. “Saline Soil Reclamation Index as an Efficient Tool for Assessing Restoration Progress of Saline Land.” Land Degradation & Development 32 (1): 123–138. doi:https://doi.org/10.1002/ldr.3641.
- Elmajdoub, B., and P. Marschner. 2015. “Response of Soil Microbial Activity and Biomass to Salinity after Repeated Additions of Plant Residues.” Pedosphere 25: 177‒185. doi:https://doi.org/10.1016/S1002-0160(15)60002-9.
- FAO (Food and Agriculture Organization of the United Nations). 2017. Global Network on Integrated Soil Management for Sustainable Use of Salt Affected Soils. Rome: FAO.
- Francois, L. E., and E. V. Maas. 1999. “Crop Response and Management of Salt-affected Soils.” In Hand Book of Plant and Crop Stress, edited by M. Pessarakli, 169‒201. New York: Marcel Dekker.
- Gao, S., K. K. Tanji, and S. C. Scardaci. 2003. “Incorporating Straw May Induce Sulfide Toxicity in Paddy Rice.” California Agriculture 57 (2): 55‒59. doi:https://doi.org/10.3733/ca.v057n02p55.
- Gee, G. W., and J. W. Bauder. 1986. “Particle-Size Analysis.” In Methods of Soil Analysis, Part 1 Physical and Mineralogical Methods, edited by G. S. Campbell, D. R. Nielsen, R. D. Jackson, A. Klute, and M. M. Mortland, 383–412. Wisconsin: American Society of Agronomy and Soil Science Society of America.
- Guo, Y., J. Huang, Z. Shi, and H. Li. 2015. “Mapping Spatial Variability of Soil Salinity in a Coastal Paddy Field Based on Electromagnetic Sensors.” PLoS One 10: e0127996. doi:https://doi.org/10.1371/journal.pone.0127996.
- Heenan, D. P., L. G. Lewin, and D. W. McCaffery. 1988. “Salinity Tolerance in Rice Varieties at Different Growth Stages.” Australian Journal of Experimental Agriculture 28 (3): 343–349. doi:https://doi.org/10.1071/EA9880343.
- Horneck, D. A., J. W. Ellsworth, B. G. Hopkins, D. M. Sullivan, and R. G. Stevens. 2007. Managing Salt-affected Soils for Crop Production. A Pacific Northwest Extension Publication (Oregon State University, University of Idaho, and Washington State University). Corvallis, Oregon: Oregon State University.
- Huang, L., X. Liu, Z. Wang, Z. Liang, M. Wang, M. Liu, and D. L. Suarez. 2017. “Interactive Effects of pH, EC and Nitrogen on Yields and Nutrient Absorption of Rice (Oryza Sativa L.).” Agricultural Water Management 194: 48‒57. doi:https://doi.org/10.1016/j.agwat.2017.08.012.
- Jeong, Y. J., S. S. Lim, H. J. Park, B. S. Seo, S. I. Park, J. H. Ryu, K. S. Lee, et al. 2020. “Evaluation of Crop Suitability for Reclaimed Tideland Soils Using Soil and Water Salinity and Soil Texture.” Korean Journal of Soil Science and Fertilizer 53 :70–81. doi:https://doi.org/10.7745/KJSSF.2020.53.1.070.
- Ju, J., Y. Yamamoto, Y. L. Wang, Y. H. Shan, G. C. Dong, A. Miyazaki, and T. Yoshida. 2009. “Genotypic Differences in Dry Matter Accumulation, Nitrogen Use Efficiency and Harvest Index in Recombinant Inbred Lines of Rice under Hydroponic Culture.” Plant Production Science 12 (2): 208–216. doi:https://doi.org/10.1626/pps.12.208.
- Kim, G. Y., B. G. Ko, H. C. Jeong, K. A. Roh, K. M. Shim, J. T. Lee, D. B. Lee, S. Y. Hong, and S. I. Kwon. 2009. “Estimating Carbon Fixation of 14 Crops in Korea.” Korean Journal of Soil Science and Fertilizer 42: 460‒466. (In Korean).
- Kim, Y. D., M. K. Choi, K. D. Lee, M. G. Baek, B. I. Ku, S. G. Kang, H. K. Park, and B. K. Kim. 2012. “Growth and Yield of Rice in Levels of Nitrogen and Water Management of Reclaimed Saline Soils in Southwestern Area.” Korean Journal of Medicinal Crop Science 57: 203‒208. (In Korean). doi:https://doi.org/10.7740/kjcs.2012.57.3.203.
- Kim, Y. D., M. K. Choi, M. G. Baek, B. I. Ku, S. G. Kang, H. K. Park, W. Y. Choi, and J. K. Ko. 2011. “Growth and Yield of Direct Seeded Rice as Influenced by Time of Basal Application of Nitrogen in Southwestern Reclaimed Saline Land.” Korean Journal of International Agriculture 23: 415‒419. (In Korean).
- Kim, Y. J., B. K. Choo, and J. Y. Cho. 2017. “Effect of Gypsum and Rice Straw Compost Application on Improvements of Soil Quality during Desalination of Reclaimed Coastal Tideland Soils: Ten Years of Long-term Experiments.” Catena 156: 131–138. doi:https://doi.org/10.1016/j.catena.2017.04.008.
- KMA (Korea Meteorological Administration). 2018. Annual Climatological Report. Seoul: KMA.
- Kuo, S. 1996. “Phosphorus.” In Methods of Soil Analysis, Part 2 Chemical Methods, edited by D. L. Sparks, A. L. Page, P. A. Helmke, R. H. Loeppert, P. N. Soltanpour, M. A. Tabatabai, C. T. Johnston, and M. E. Sumner, 869–919. Wisconsin: American Society of Agronomy and Soil Science Society of America.
- Kwak, J. H., W. J. Choi, S. S. Lim, S. H. Lee, S. M. Lee, S. X. Chang, J. W. Jung, K. S. Yoon, and S. M. Choi. 2008. “Sources and Transformations of N in Reclaimed Coastal Tidelands: Evidence from Soil δ15N Data.” Environmental Geology 53: 1331‒1338. doi:https://doi.org/10.1007/s00254-007-0741-7.
- Lal, R. 2004. “Soil Carbon Sequestration to Mitigate Climate Change.” Geoderma 123 (1–2): 1‒22. doi:https://doi.org/10.1016/j.geoderma.2004.01.032.
- Lim, S. S., H. I. Yang, H. J. Park, S. I. Park, B. S. Seo, K. S. Lee, S. H. Lee, et al. 2020. “Land-use Management for Sustainable Rice Production and Carbon Sequestration in Reclaimed Coastal Tideland Soils of South Korea: A Review.” Soil Science and Plant Nutrition 66 :60–75. doi:https://doi.org/10.1080/00380768.2019.1674121.
- Lim, S. S., W. J. Choi, K. S. Lee, and H. M. Ro. 2012. “Reduction in CO2 Emission from Normal and Saline Soils Amended with Coal Fly Ash.” Journal of Soils and Sediments 12: 1299‒1308. doi:https://doi.org/10.1007/s11368-012-0545-6.
- Lim, S. S., W. J. Choi, S. X. Chang, M. A. Arshad, K. S. Yoon, and H. Y. Kim. 2017. “Soil Carbon Changes in Paddy Fields Amended with Fly Ash.” Agriculture, Ecosystems & Environment 245: 11–21. doi:https://doi.org/10.1016/j.agee.2017.03.027.
- Maas, E. V., and G. J. Hoffman. 1977. “Crop Salt Tolerance – Current Assessment.” ASCE Journal of the Irrigation and Drainage Division 103: 115‒134.
- MOAF (Ministry of Agriculture, Food and Rural Affairs). 2019. Agriculture, Food and Rural Affairs Statistics Yearbook. Sejong: MOAF.
- Moon, Y. H., Y. R. Kwon, B. K. Ahn, D. H. Kim, and S. S. Han. 2011. “Impact of Compost Application on Improvement of Rice Productivity and Quality in Reclaimed Soil.” Korean Journal of Soil Science and Fertilizer 44: 808–813. (In Korean). doi:https://doi.org/10.7745/KJSSF.2011.44.5.808.
- Mulvaney, R. L. 1996. “Nitrogen-Inorganic Forms.” In Methods of Soil Analysis, Part 2 Chemical Methods, edited by D. L. Sparks, A. L. Page, P. A. Helmke, R. H. Loeppert, P. N. Soltanpour, M. A. Tabatabai, C. T. Johnston, and M. E. Sumner, 1123–1284. Wisconsin: American Society of Agronomy and Soil Science Society of America.
- Nam, J. K., and T. O. Kwon. 1999. “Influence of Tillage Methods on the Growth and Yield of Rice for Direct Seeding on Wet Paddy Surface of Reclaimed Saline Land.” Journal of Life Sciences and Natural Resources 21: 22‒40. (In Korean).
- Nelson, D. W., and L. E. Sommers. 1982. “Total Carbon, Organic Carbon, and Organic Matter.” In Methods of Soil Analysis, Part 2 Chemical Methods, edited by D. L. Sparks, A. L. Page, P. A. Helmke, R. H. Loeppert, P. N. Soltanpour, M. A. Tabatabai, C. T. Johnston, and M. E. Sumner, 539–580. Wisconsin: American Society of Agronomy and Soil Science Society of America.
- Nemati, I., F. Moradi, S. Gholizadeh, M. A. Esmaeili, and M. R. Bihamata. 2011. “The Effect of Salinity Stress on Ions and Soluble Sugars Distribution in Leaves, Leaf Sheaths and Roots of Rice (Oryza Sativa L.) Seedlings.” Plant, Soil and Environment 57: 26–33. doi:https://doi.org/10.17221/71/2010-PSE.
- Nishigaki, T., S. Sugihara, K. Kobayashi, Y. Hashimoto, M. Kilasara, H. Tanaka, T. Watanabe, and S. Funakawa. 2018. “Fractionation of Phosphorus in Soils with Different Geological and Soil Physicochemical Properties in Southern Tanzania.” Soil Science and Plant Nutrition 64 (3): 291‒299. doi:https://doi.org/10.1080/00380768.2018.1436406.
- Ochiai, K., S. Uemura, A. Shimizu, Y. Okumoto, and T. Matoh. 2008. “Boron Toxicity in Rice (Oryza Sativa L.) I. Quantitative Trait Locus (QTL) Analysis of Tolerance to Boron Toxicity.” Theoretical and Applied Genetics 117: 125–133. doi:https://doi.org/10.1007/s00122-008-0758-7.
- Park, H. J., S. S. Lim, J. H. Kwak, K. S. Lee, H. I. Yang, H. Y. Kim, S. M. Lee, and W. J. Choi. 2020. “Biomass, Chemical Composition, and Microbial Decomposability of Rice Root and Straw Produced under Co-elevated CO2 and Temperature.” Biology and Fertility of Soils 56: 991–1005. doi:https://doi.org/10.1007/s00374-020-01471-y.
- Pearce, A. D., C. R. Dillon, T. C. Keisling, and C. E. Wilson. 1999. “Economic and Agronomic Effects of Four Tillage Practices on Rice Produced on Saline Soils.” Journal of Production Agriculture 12 (2): 305‒312. doi:https://doi.org/10.2134/jpa1999.0305.
- Philip, J. W., and M. R. Broadley. 2001. “Chloride in Soils and Its Uptake and Movement within the Plant: A Review.” Annals of Botany 88: 967‒988. doi:https://doi.org/10.1006/anbo.2001.1540.
- Rashed, A. A., E. Khlifa, and H. S. Fahmy. 2003. “Paddy Rice Cultivation in Irrigated Water Managed Saline Sodic Lands under Reclamation, Egypt.” Paper no. 071, 9th International Drainage Workshop, Utrecht, The Netherlands, September 10 –13.
- RDA (Rural Development Administration). 2000. Taxonomical Classification of Korean Soils. Suwon, Wanju, Republic of Korea: RDA.
- RDA (Rural Development Administration). 2017. Standards of Crop Fertilization Management. Wanju, Republic of Korea: RDA. (In Korean).
- RDA (Rural Development Administration). 2019. Livestock Statistics. Wanju, Republic of Korea: RDA. (In Korean).
- Rhoades, J. D. 1996. “Salinity: Electrical Conductivity and Total Dissolved Solids.” In Methods of Soil Analysis, Part 3 Chemical Methods, edited by D. L. Sparks, A. L. Page, P. A. Helmke, R. H. Loeppert, P. N. Soltanpour, M. A. Tabatabai, C. T. Johnston, and M. E. Sumner, 417‒436. Wisconsin: American Society of Agronomy and Soil Science Society of America.
- Rui, W. Y., and W. J. Zhang. 2010. “Effect Size and Duration of Recommended Management Practices on Carbon Sequestration in Paddy Field in Yangtze Delta Plain of China: A Meta-Analysis.” Agriculture, Ecosystems & Environment 135 (3): 199–205. doi:https://doi.org/10.1016/j.agee.2009.09.010.
- Sahrawat, K. L. 2004. “Organic Matter Accumulation in Submerged Soils.” Advances in Agronomy 81: 169–201. doi:https://doi.org/10.1016/S0065-2113(03)81004-0.
- Shi, Z., Y. Li, R. Wang, and F. Makeschine. 2005. “Assessment of Temporal and Spatial Variability of Soil Salinity in a Coastal Saline Field.” Environmental Geology 48 (2): 171–178. doi:https://doi.org/10.1007/s00254-005-1285-3.
- Singh, A. 2015. “Soil Salinization and Waterlogging: A Threat to Environment and Agricultural Sustainability.” Ecological Indicators 57: 128–130. doi:https://doi.org/10.1016/j.ecolind.2015.04.027.
- Sumner, M. E., and W. P. Miller. 1996. “Cation Exchange Capacity and Exchange Coefficients.” In Methods of Soil Analysis, Part 3 Chemical Methods, edited by D. L. Sparks, A. L. Page, P. A. Helmke, R. H. Loeppert, P. N. Soltanpour, M. A. Tabatabai, C. T. Johnston, and M. E. Sumner, 1201–1229. Wisconsin: American Society of Agronomy and Soil Science Society of America.
- Wilson, C. E., T. C. Keisling, D. M. Miller, C. R. Dillon, A. D. Pearce, D. L. Frizzell, and P. A. Counce. 2000. “Tillage Influence on Soluble Salt Movement in Silt Loam Soils Cropped to Paddy Rice.” Soil Science Society of America Journal 64 (5): 1771‒1776. doi:https://doi.org/10.2136/sssaj2000.6451771x.
- Wong, V. N. L., R. S. B. Greene, R. C. Dalal, and B. W. Murphy. 2010. “Soil Carbon Dynamics in Saline and Sodic Soils: A Review.” Soil Use and Management 26 (1): 2–11. doi:https://doi.org/10.1111/j.1475-2743.2009.00251.x.
- Xu, Z., T. Shao, Z. Lv, Y. Yue, A. Liu, X. Long, Z. Zhou, X. Gao, and Z. Rengel. 2020. “The Mechanisms of Improving Coastal Saline Soils by Planting Rice.” Science of the Total Environment 703: 135529. doi:https://doi.org/10.1016/j.scitotenv.2019.135529.
- Zeng, L., M. C. Shannon, and S. M. Lesch. 2001. “Timing of Salinity Stress Affects Rice Growth and Yield Components.” Agricultural Water Management 48 (3): 191–206. doi:https://doi.org/10.1016/S0378-3774(00)00146-3.