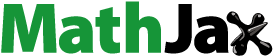
ABSTRACT
Our previous pot experiments showed that using Azolla either or both as dual and green manure with rice increases its yield or significantly reduces either or both methane (CH4) and nitrous oxide (N2O) emissions. To confirm these findings in an actual field, Azolla was either grown as a dual crop (herein Cover) or incorporated as green manure plus dual cropping (herein AGM + Cover) at the beginning of the experiment along with rice. Compared with the control (chemical fertilizer; herein NPK), NPK + Cover and AGM + Cover treatments did not influence cumulative CH4 emissions throughout the rice growth period. However, AGM + Cover treatment affected significant CH4 emissions at early, middle, and later rice growth stages by 140.6%, 24.6%, and 33.1%, respectively, compared with NPK + Cover treatment. These emissions were attributed to the readily available carbon substrate for methanogens following the incorporation of Azolla as green manure. Compared with NPK, NPK + Cover and AGM + Cover significantly increased N2O emissions by 645.9% and 816.2%, respectively, during the middle rice growth stage. No significant N2O emission differences were observed in the three treatments in the early or later rice growth stages. The higher N2O emissions from the middle rice growth stage were ascribed to high substrate availability from the dead Azolla by higher summer air temperature in the 2019 season. AGM + Cover significantly decreased rice yield by 37.5% (NPK) and 35.3% (NPK + Cover), with no significant differences between NPK and NPK + Cover. This reduction was attributed to nitrogen immobilization from the incorporated Azolla during the early stage. Therefore, to ascertain the potential of Azolla in paddy fields to address environmental safety while sustaining yield, emphasis on the interaction of different application methods with various management practices is necessary.
1. Introduction
Methane (CH4) and nitrous oxide (N2O) are two important greenhouse gases (GHGs) contributing to global warming and, consequently, climate change, with global warming potentials of 34 and 298 carbon dioxide-equivalents, respectively, on a 100-year time horizon (IPCC Citation2013). Rice feeds over half of the world’s population, with its paddies accounting for global CH4 (7%–17%) and total anthropogenic N2O (13%–24%) emissions annually (Scheehle and Kruger Citation2006). According to Seck et al. (Citation2012), rice production globally needs to increase by over 116 million Mg by 2035 to meet the demands of population growth. Consequently, it is predicted to increase emissions of CH4 (Anastasi, Dowding, and Simpson Citation1992), and N2O following higher application of nitrogenous fertilizers (Zou et al. Citation2009). CH4 is primarily produced through strictly anaerobic decomposition of organic matter (Malyan et al. Citation2016), whereas N2O emissions mainly depend on nitrification and heterotrophic denitrification processes (Butterbach-Bahl et al. Citation2013). However, GHG emissions from lowland rice paddies are intricate, making management practices necessary to mitigate tradeoffs between CH4 and N2O emissions (Li Citation2007; Oertel et al. Citation2016).
Azolla (A. filiculoides Lam.) is a free-floating aquatic fern in a symbiotic association with nitrogen (N)-fixing cyanobacterium Anabaena Azollae (Wagner Citation1997). Azolla is globally widespread and extensively used as green manure ―either incorporated into the soil before rice transplanting or grown as a dual crop along with rice― to improve soil properties and maintain the biological balance in lowland rice paddies (Yadav et al. Citation2014; Kollah, Patra, and Mohanty Citation2016). Recently, the application of Azolla either as dual or green manure accompanying rice has been proven to influence GHG emissions, mainly CH4 and N2O singly or together, from continuously flooded rice ecosystems (Chen et al. Citation1995, Citation1997; Bharati et al. Citation2000; Ying et al. Citation2000). However, these observations lack unanimity partly due to the soil, environmental, and experimental management practices (Snyder et al. Citation2009). According to previous pot experiments (Kimani et al. Citation2018, Citation2020), the application of Azolla either as a dual crop singly with rice or both as a dual crop and as green manure showed varying effects on the simultaneous emissions of CH4 and N2O from a continuously flooded paddy soil. The views on the emissions of either CH4 or N2O are varied, with few reports on the effects of Azolla application on simultaneous CH4 and N2O emissions from continuously flooded rice paddies. The objectives of this study were to determine the effects of Azolla application either as a dual crop without incorporation or incorporated as green manure in conjunction with dual cropping on the simultaneous emission of CH4 and N2O and rice growth in an actual rice field.
2. Materials and methods
2.1. Site description and preparation
A field experiment was conducted in 2019 on a newly constructed paddy field at the Experimental Farm in the Faculty of Agriculture campus, Yamagata University, Tsuruoka (38°44ʹN, 139°50ʹE; 16 m elevation). The field site was an unutilized upland field abandoned many years ago due to the high groundwater level. During the rice growth season (June 10 to September 17), the climate was hotter and sunnier than the historical average from 1981 to 2010 (). The average daily air temperature and sunshine time during the rice growth season were 23.6°C and 6.3 h, whereas the historical averages of 1981–2010 were 22.6°C and 5.75 h, respectively. Primarily, the average daily air temperature (28.3°C) and sunshine time (8.3 h) during the reproductive to ripening growth stages of rice (53–80 days after transplanting (DAT)) were significantly higher than historical averages of 1981–2010 (). Also, the total precipitation was 525 mm over the whole rice cultivation period, and the weather data were retrieved from the Japan Meteorological Agency database (http://www.data.jma.go.jp/obd/stats/etrn/index.php). The soil was classified as an Inceptisol according to the USDA Soil Taxonomy. The surface soil layer (approximately 0–20 cm depth) had a soil pH (1:5 H2O) and electrical conductivity of 5.55 and 125.7 µS cm−1, respectively. Soil organic carbon, total nitrogen, and its ratio (C/N) were 12.4 g C kg−1, 1.12 g N kg−1, and 11.05, respectively. Soil ammonium-N (NH4+-N), nitrate-N (NO3–N), and available phosphate (Troug method) were 10.06 mg N kg−1, 2.82 mg N kg−1, and 174.1 mg P2O5 kg−1, respectively. The soil properties were determined using the air-dried soil sample procedures (JSSSPN Citation1986). Methods described by Kimani et al. (Citation2018), Kimani et al. (Citation2020)) were used to analyze the initial soil samples.
Figure 1. Daily sunshine time (■) and average air temperature (●) during the experimental period from 10th June to 18 September 2019 in Tsuruoka, Japan. The bold and dashed lines crossing air temperature and sunshine time are the daily average values for 1981–2010, respectively. Data were derived from the Japan Meteorological Agency.
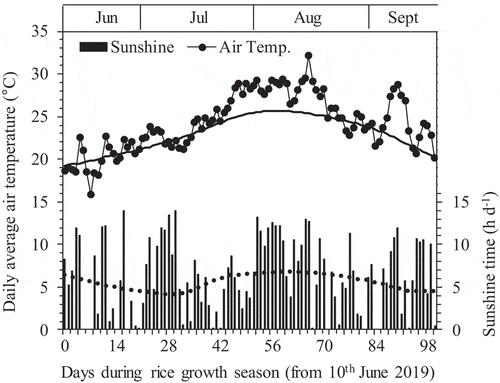
The new experimental field was plowed, flooded, and thoroughly puddled before transplanting. Twelve plots with an individual plot size of 1.35 m2 (0.9 m wide × 1.5 m long) were set up in the field for three treatments, as shown below. A spacing of 0.30 m and 2.5 m separated the plots and blocks, respectively. The individual plot borders were established with corrugated plastic sheets (Azenami) pressed into the soil to prevent water gain and loss within the plot.
2.2. Treatment and management practices
Three treatments, chemical fertilization (NPK), chemical fertilizer and Azolla, cover as a dual crop (NPK + Cover), and Azolla as green manure plus dual cropping without chemical fertilization (AGM + Cover); each was replicated four times and laid out in a randomized block design. On 10 June 2019, three seedlings per hill of Haenuki cv. (a popular rice cultivar in Yamagata, Japan) were transplanted at a rate of 22.2 hill m−2 (30 × 15 cm/hill). The Azolla (Azolla filiculoides Lam.) species IRRI code F1 1001 was used in this experiment (Cheng et al. Citation2015; Kimani et al. Citation2018).
One day before transplanting, NPK and NPK + Cover plots were fertilized using the nitrogen-phosphorus-potassium (NPK: 15–15-15) compound fertilizer at the recommended rate of 7.0 g N m−2 for the Haenuki rice cultivar in the field (Itoh et al. Citation2011), with no supplementary fertilizers added throughout the rice growth period in the two treatments. NPK + Cover treatments were inoculated once with 200 g fresh Azolla/plot as a dual crop on the date of rice transplanting. In contrast, AGM + Cover treatments were incorporated with 5.7 kg fresh Azolla/plot a day before rice transplanting. The Azolla cover present in the AGM + Cover plots emerged one week following the incorporation of Azolla as green manure. In the AGM + Cover treatments, fresh Azolla, at 3.3% N and 95% water content, was applied to supply 7.0 g N m−2.
The field was irrigated, and paddy water depth in the treatment plots was maintained at approximately 5–7 cm above the soil’s surface during the rice growth period. The rice crop was grown without any application of pesticides. Also, the heading date for the three treatments varied between 70 and 77 DAT. At maturity, 17 September 2019 (99 DAT), eight hills per plot were harvested to determine grain yield.
2.3. Measurements of CH4 and N2O fluxes and plant night respiration (CO2 flux)
Gas fluxes for CH4 and N2O, and plant nighttime respiration (CO2 flux) in the field were measured for each plot after every 2 weeks at 8–49 DAT and after 77 DAT, and once per week after 49–77 DAT and after 91 DAT, using closed static rectangular acrylic chambers (0.45 or 1.0 m high, 0.6 × 0.6 = 0.36 m2 area). Eight rice hills were included within the chamber, and their heights were adjusted to accommodate the increasing rice height. The bases of the chambers were inserted into the soils to an approximately 2 cm depth and left through the experimental period. Each chamber was fitted with an electric fan to mix the gas and a rubber septum to aid sample collection using a syringe (Itoh et al. Citation2011). The gas in the chamber per plot was withdrawn with a 30-ml plastic syringe in triplicate within 30 minutes (0, 15, 30 min) between 20:00 and 22:00 h and transferred immediately to 19-ml pre-evacuated injection vials fitted with butyl rubber stoppers and screw caps. The flux measurements were made from a metal-walk to reduce soil disturbance and prevent the flare-up of gases from the soil during the measurements. The vials were simultaneously analyzed for the concentrations CH4, N2O, and CO2 using gas chromatographs equipped with flame ionization detector(FID), electron capture detector (ECD), and thermal conductivity detector (TCD), respectively, at the Institute of Agro-Environmental Sciences, NARO. The fluxes were calculated from the linear increase in gas concentrations inside the chamber with time and atmospheric pressure (Sudo Citation2006; Cheng et al. Citation2008; Kimani et al. Citation2018). We also measured the CO2 respiration as well as CH4 and N2O fluxes at night time about 20:00–22:00, since the average daily CH4 flux from rice paddy was similar to that at day time 8:00–10:00 and night time 20:00–22:00 (Cheng et al. Citation2008; Minamikawa et al. Citation2015).
2.4. Measurements of CO2, CH4, and NO3−–N dissolved in soil solution
The method described by Kimani et al. (Citation2018) was used to determine the dissolved CH4 and CO2 concentrations in soil solutions sampled on the same day after gas measurements to understand the CH4 and N2O emissions with the C dynamics in the soil. Briefly, a 9.5-ml soil solution sample per plot was aspirated into a semi-evacuated 19-mL vial using a Rhizon soil solution sampler. The sampler was inserted vertically into the soil at a 10–15 cm depth between the rice plants placed inside the chamber base and the edges a day after rice transplanting. The gas concentrations in the headspace were analyzed using a gas chromatograph (GC-72A, Shimadzu, Kyoto, Japan) equipped with a thermal conductivity detector (TCD) and a flame ionization detector (FID) for CO2 and CH4, respectively. The respective concentrations in the headspace were calculated according to Henry’s law (Cheng et al. Citation2005, Citation2006). After analyzing the CO2 and CH4 in the headspace, the solution samples were stored in a freezer at −18°C before analyzing the NO3−–N concentrations dissolved in the soil solution. The NH4+-N was not detected in the solution.
2.5. Investigation of rice growth and grain yield
The rice height, tiller number, and top rice leaf greenness (SPAD value) of each rice plant per hill were measured once a week beginning at 8 DAT. The SPAD value was measured using a SPAD-502 Plus chlorophyll meter (Konica Minolta Inc., Tokyo, Japan). Data from eight hills per treatment/plot were averaged during the rice growth. Aboveground parts of the eight rice plants at the center of the plot for all treatments were harvested at 99 DAT then separated into grains and straw. All samples were air-dried for 1 month and weighed to determine total yield (Cheng et al. Citation2009).
2.6. Evaluation of global warming potential (GWP) and yield-scaled GWP emissions
The effects of Azolla as green manure incorporation or dual cropping on total GWP and yield-scaled GWP compared with NPK treatment were evaluated. First, the total GWP was calculated as g CO2 equivalents (CO2 eq) over a 100-year time horizon using radiative forcing potential of 34 for CH4 and 298 for N2O relative to CO2 (Formula 1, IPCC Citation2013). Then, yield-scaled GWP values were determined by dividing total GWP by grain yield (Formula 2).
2.7. Statistical analysis
A repeated measure of analysis of variance (ANOVA) was used to test the effects of management practice (NPK, NPK + Cover, and AGM + Cover). The differences in the grain yield, harvest index, CH4 and N2O emission fluxes and cumulative emissions, total GWP, and yield-scaled GWP emissions were tested using the Tukey’s HSD (honestly significant difference) post hoc test at 0.05 probability level. The differences of AGM + Cover effect were tested using the Student’s t-test. The statistical analyses were conducted using SPSS Statistics v. 20.0 (SPSS Inc., Chicago, IL, USA). ANOVA results showed there were no significant block effects in this study (Table S1 and S2).
3. Results
3.1. CH4 and N2O fluxes and their cumulative emissions
The CH4 and N2O fluxes and their cumulative emissions during the rice-growing season for the three treatments were divided into three rice growth stages i.e., vegetative (before 35 DAT), reproductive (35–70 DAT), and grain filling to maturation (70–98 DAT), (, and ). Before 35 DAT, CH4 flux rates were between 0.47 and 17.2 mg C m−2 h−1 for NPK, 0.38–11.8 mg C m−2 h−1 for NPK + Cover, and 0.60–17.6 mg C m−2 h−1 for AGM + Cover treatments. In comparison to NPK and NPK + Cover treatments, AGM + Cover treatment had a significantly higher (at P< 0.01) CH4 flux peak at 21 DAT, and at 35 DAT (at P= 0.033) compared with NPK + Cover but not NPK treatment. The flux of CH4 increased continuously after 35–70 DAT under all treatments (22.6–36.3 mg C m−2 h−1) and attained the highest fluxes at 56 DAT of 33.6, 29.8, and 36.3 mg C m−2 h−1 for NPK, NPK + Cover, and AGM + Cover treatments, respectively. There were no significant flux differences among the treatments at 56 DAT. The CH4 flux rates at 70–98 DAT were 7.40–15.5 mg C m−2 h−1 (NPK), 9.98–18.0 mg C m−2 h−1 (NPK + Cover), and 10.5–21.7 mg C m−2 h−1 for AGM + Cover treatments. Compared to the other two treatments, AGM + Cover treatment recorded a slightly higher but not significantly different flux peak at 91 DAT (21.7 mg C m−2 h−1; at P = 0.074) ()).
Table 1. Cumulative CH4 and N2O emissions during early (before 35 DAT), middle (35–70 DAT), and later (70–98 DAT) rice growth stages, and seasonal total cumulative emissions as affected by Azolla application practices
Figure 2. Changes in CH4 (a) and N2O (b) fluxes from plots treated with chemical fertilizer (NPK), NPK plus Azolla cover (NPK + Cover), and Azolla as green manure plus Azolla cover (AGM + Cover) throughout the experimental period. Vertical bars indicate standard error (n = 4). Dashed lines show the early, middle and later rice growth stages.
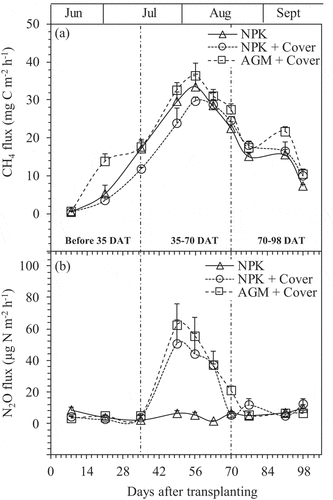
Unlike CH4, N2O fluxes under all treatments before 35 DAT (2.27–8.54 µg N m−2 h−1) were not significantly different ()). At 35–70 DAT, NPK + Cover (5.62–50.6 µg N m−2 h−1), and AGM + Cover (21.0–62.4 µg N m−2 h−1) treatments significantly increased N2O flux rates compared with NPK treatment (1.47–6.46 µg N m−2 h−1). However, N2O fluxes at 70–98 DAT under all treatments (between 4.79–12.3 µg N m−2 h−1) were not significantly different ()).
As shown in , cumulative CH4 emissions before 35 DAT and during the reproductive stage (35–70 DAT) for NPK + Cover treatment were significantly lower (P < 0.05) compared with AGM + Cover treatment but not NPK treatment. During these two rice growth stages, no significant cumulative CH4 emissions were observed between NPK and AGM + Cover treatments. Additionally, no significant cumulative CH4 emission differences were observed at 70–98 DAT among the three treatments (). Cumulative N2O emissions for all treatments before 35 DAT, or at 70–98 DAT were not significantly different, but at 35–70 DAT NPK + Cover and AGM + Cover treatments showed significantly higher cumulative N2O emissions compared with NPK. Also, no significant emission differences were observed between NPK + Cover and AGM + Cover treatments ().
The NPK + Cover treatment recorded significantly lower total cumulative CH4 emissions relative to AGM + Cover but not NPK treatments with no significant total cumulative CH4 emissions observed between NPK and AGM + Cover treatments over the single rice growth period. For the total cumulative N2O, NPK + Cover and AGM + Cover treatments recorded significantly higher total emissions than NPK treatment, whereas no significant total cumulative N2O emissions were observed between NPK + Cover and AGM + Cover treatments ().
3.2. CO2 night respiration
Night respiration of CO2 emitted mainly from rice plants in the NPK treatment plots and both rice plants and floating Azolla masses in the NPK + Cover and AGM + Cover treatment plots ranged from 21.5 to 341.0 mg C m¯2 h¯1 during the experimental period with variations within the rice growth stages (Figure S1(a)). The highest peak of night time CO2 respiration was found at 35–70 DAT in all treatments, mostly attributed to high daytime and night temperature at sampling (Figure S1(b)). The average values of CO2 respiration during the entire experiment period were 393.4, 487.8, 432.1 mg C m¯2 h¯1 from NPK, NPK + Cover, and AGM + Cover treatments, respectively.
3.3. Concentrations of CO2 and CH4 dissolved in soil solution
As shown in ), the concentration of dissolved CO2 in the soil solution of the AGM + Cover treatment was significantly higher than those in NPK and NPK + Cover treatments during the rice growth period. Before 35 DAT, AGM + Cover treatment recorded significantly (P < 0.05) higher dissolved CO2 in soil solution (at 195.9–307.4 µg C mL−1), compared with NPK (at 142.0–219.8 µg C mL−1), and, NPK + Cover (at 145.6–254.5 µg C mL−1). Similarly, at 35–70 DAT, dissolved CO2 in the soil solution of AGM + Cover (123.0–185.4 µg C mL−1) was significantly higher than that in NPK (86.5–124.4 µg C mL−1), and NPK + Cover (87.8–129.8 µg C mL−1) treatments. Additionally, at 70–98 DAT, dissolved CO2 in soil solution for AGM + Cover (86.8–122.9 µg C mL−1) was higher than that in NPK (69.2–73.3 µg C mL−1), and, NPK + Cover (57.4–79.6 µg C mL−1) treatments.
Figure 3. Changes in the concentration of CO2 (a) and CH4 (b) dissolved in the soil solution in plots treated with chemical fertilizer (NPK), NPK plus Azolla cover (NPK + Cover), and Azolla as green manure plus Azolla cover (AGM + Cover) throughout the experimental period. Vertical bars indicate standard error (n = 4). Dashed lines show the early, middle and later rice growth stages.
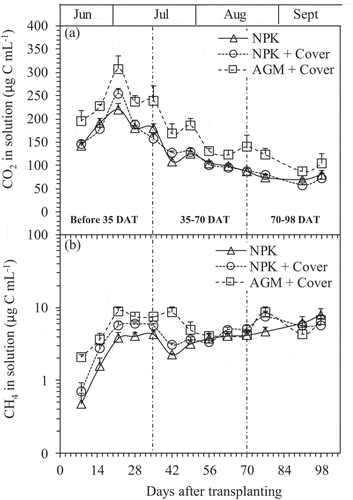
Also, as shown in ), the dissolved CH4 in soil solution for AGM + Cover treatment (2.07–8.90 µg C mL−1) at 8–21 DAT was significantly higher (P < 0.05) compared with that of NPK (0.47–3.80 µg C mL−1), and, NPK + Cover (0.69–5.65 µg C mL−1) treatments. Additionally, AGM + Cover treatment recorded significantly higher (P < 0.05) dissolved CH4 in soil solution flux at 42 DAT compared with NPK and NPK + Cover treatments; and at 77 DAT compared with NPK but not NPK + Cover treatment. No significant relationships were observed between the daily CH4 and N2O emission fluxes and CO2 and CH4 concentrations dissolved in the soil solution, as shown in Figure S2 and S3.
3.4. Rice plant growth parameters and grain yield
Weekly changes in rice growth parameters (i.e., height, tiller number, and leaf color) for all treatments from transplanting to harvesting (about 98 DAT) are as shown in , respectively. Rice plant height increased steadily for all treatments until 77 DAT, with a significantly lower height in AGM + Cover treatment than NPK and NPK + Cover treatments during 15–56 DAT ()). At harvest, the rice shoot height was 100.4, 104.7, and 102.9 cm for NPK, NPK + Cover, and AGM + Cover treatments, respectively. There were no significant rice shoot height differences among all treatments between 63 and 98 DAT.
Figure 4. Plant height (a), tiller number (b), and leaf color measured in SPAD values (c) of rice plants from plots treated with chemical fertilizer (NPK), NPK plus Azolla cover (NPK + Cover), and Azolla as green manure plus Azolla cover (AGM + Cover) throughout the experimental period. Vertical bars indicate standard error (n = 4). Dashed lines show the early, middle and later rice growth stages.
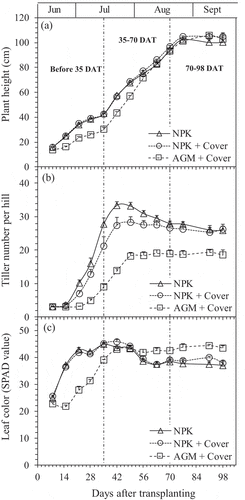
The rice tiller numbers for NPK, NPK + Cover, and AGM + Cover treatments reached maxima of 33.3, 28.3, and 19.4 at 42, 49, and 91 DAT, respectively ()). The productive tillers per hill at harvest were 25.8, 26.2, and 18.6 for NPK, NPK + Cover, and AGM + Cover treatments, respectively. NPK and NPK + Cover treatments recorded significantly higher (P < 0.01) tiller numbers per hill than AGM + Cover treatment during 21–98 DAT, with no significant differences between NPK and NPK + Cover treatments.
The top leaf SPAD value reached its maximum value at 35, 42, and 91 DAT for NPK, NPK + Cover, and AGM + Cover treatments, respectively, as shown in ), whereas the maximum values were 44.7 (NPK), 45.8 (NPK + Cover), and 44.2 (AGM + Cover). The NPK and NPK + Cover treatments maintained significantly (P < 0.01) higher SPAD values than AGM + Cover treatment during 15–35 DAT. In contrast, the AGM + Cover treatment maintained significantly higher leaf greenness during 63–98 DAT than NPK and NPK + Cover treatments ()). NPK and NPK + Cover treatments also maintained significantly higher grain yields than AGM + Cover treatment at harvest; however, there were no significant harvest index differences among the treatments ().
Table 2. Rice grain yield, harvest index, GWP (CH4 and N2O emissions), and yield-scaled GWP as affected by Azolla application practices
3.5. Global warming potentials of CH4 and N2O and yield-scaled emissions
As shown in , the total combined (CH4 plus N2O) global warming potential (GWP) of NPK treatment was not significantly different compared with either the NPK + Cover or AGM + Cover treatments. However, NPK + Cover significantly decreased (P < 0.05) the total GWP compared with AGM + Cover. The estimated per yield-scaled GWP calculated as total GWP divided by rice grain yield was significantly lower in NPK and NPK + Cover treatments than in AGM + Cover treatment. Also, shows no significant yield-scaled GWP emissions between NPK and NPK + Cover treatments.
4. Discussion
4.1. Effect of Azolla application on CH4 emissions from a paddy field
In this study, the field application of Azolla as a cover without incorporation in conjunction with chemical fertilizer at basal (i.e., NPK + Cover), numerically but not significantly decreased the total cumulative CH4 emissions throughout the rice growth period compared to the NPK treatment (). In our previous pot experiment, we found that Azolla cover (similar to NPK + Cover in this study) significantly decreased the seasonal CH4 emission by 34.7% compared with the control treatment without Azolla cover (identical to NPK in this study), with the bulk of CH4 emitted after rice heading stage (Kimani et al. Citation2018). We did not find dead Azolla during middle and later rice growth stages in the pot experiment. However, the decrease in CH4 emissions was likely attributed to an increase in dissolved oxygen concentration and redox potential at the soil-water interface between flooding water and soil surface (Kimani et al. Citation2018). However, in the current study, we observed a lot of dead Azolla cover from the middle rice growth stage due to the higher daily temperatures in the summer of 2019, as shown in . This result implies that the numerical but insignificant decrease in CH4 emission by Azolla cover was due to the Azolla growth situation. Azolla cover dual crop with rice decreases CH4 emissions due to; (1) dissolved oxygen enrichment at the soil–water interface and along the soil’s gradient favoring CH4 oxidation, and the higher soil redox potential limiting methanogenesis (Bharati et al. Citation2000; Xu et al. Citation2017); either both or (2) an increase in CH4 emissions from continuously flooded rice paddies attributed to the death and decomposition of floating Azolla masses during the rice growth period (Chen et al. Citation1997; Ying et al. Citation2000).
Previously, from our other pot experiment (Kimani et al. Citation2020), the incorporation of Azolla as green manure plus its subsequent growth as a dual crop, in conjunction with chemical fertilizers, either at basal or top-dressing along with rice, significantly increased cumulative CH4 emissions by 123.3% (AGM) and 176.7% (NPK + AGM) at early rice growth stages (before heading); and total cumulative CH4 emissions by 31.5% (AGM) and 43.5% (NPK + AGM), compared with the NPK only treatment after 112 DAT. This result was mainly attributed to the easy degradability of organic amendments, including Azolla, their incorporation and subsequent decomposition offering a predominant pool of substrates for the methanogenic community in the soil (Yagi and Minami Citation1990; van der Gon and Neue Citation1995; Malyan et al. Citation2016). However, under field conditions, compared with NPK treatment, the incorporation of Azolla as green manure plus its subsequent growth as a cover crop without NPK application (i.e., AGM + Cover) did not affect significantly higher cumulative CH4 emissions throughout the rice growth stages and the total seasonal cumulative emissions (). Conversely, compared with NPK + Cover treatment, AGM + Cover significantly increased CH4 emissions at the early and middle rice growth stages by 140.6% and 24.6%, respectively, and subsequently stimulated greater total cumulative CH4 emissions significantly by 33.1% during the rice growth season (). The high cumulative CH4 emissions in the early rice growth stages for the AGM + Cover treatment relative to those of NPK + Cover treatments coincided with the active period of the incorporated Azolla decomposition (Watanabe et al. Citation1989) also in tandem with significantly greater (at P < 0.05) dissolved CO2 and CH4 concentrations in the soil solution (). Similarly, Bharati et al. (Citation2000) reported a significantly higher CH4 flux during the first 20 days following the incorporation of fresh Azolla at N equivalent rate of 30 kg N ha¯1.
Generally, it is well established that greater than 75% of the total seasonal cumulative CH4 emissions measured in continuously flooded rice paddies during the reproductive and ripening stages of rice are due to the easily decomposable organic inputs from rice photosynthesis, with approximately 80%–90% emitted through plant aerenchyma tissue ―the main CH4 emission pathway in rice fields― (Kimura et al. Citation1992; Huang, Sass, and Fisher Citation1997). According to our study, the ratio of cumulative CH4 emissions during the middle and later rice growth stages to the total emissions were 86.3%, 90.2%, and 81.8% for NPK, NPK + Cover, and AGM + Cover treatments, respectively (). Furthermore, these emissions were significantly related to the night plant respirations after 35 DAT in all treatments (), coinciding with the higher physical rice growth characteristics of both the rice height and tiller number (), therefore confirming our explanation above. These observations were consistent with our previous pot conclusions, where the initially incorporated Azolla as green manure and a consequent observation of masses of dead Azolla cover did not affect CH4 emissions at the late rice growth stages (Kimani et al. Citation2020). Therefore, management practices are necessary to reduce bulk of seasonal CH4 emissions consistent in the later rice growth stages, irrespective of application and/or incorporation of Azolla as green manure.
4.2. Effect of Azolla application on N2O emissions from a paddy field
In this study, compared with NPK treatment, the application of Azolla either as a dual or both incorporated as green manure did not affect significant N2O fluxes before 35 DAT or after 70 DAT among all treatments. However, significantly higher emission fluxes were observed for both NPK + Cover (35–63 DAT) and AGM + Cover (35–70 DAT) treatments (), ). These findings were contrary to our pot experiment observations, where Azolla as a cover did not significantly influence N2O emissions (Kimani et al. Citation2018). However, incorporating Azolla as green manure plus dual cropping significantly decreased the total seasonal cumulative N2O emissions by 70.9% (AGM) and 78.2% (NPK + AGM) compared with the NPK treatment (Kimani et al. Citation2020). The N2O was most emitted during the first 4 weeks after rice transplanting in the two pot experiments. Additionally, cultivated soils were flooded a day before transplanting, and significant N2O emissions were contributed due to the denitrification of high initial NO3−–N in moist bulk soils (NO3−–N concentration at 80–102 mg N kg−1 dry soil) (Kimani et al. Citation2018, Citation2020). However, in this experiment, the new field was flooded about 3 weeks earlier, and the initial NO3−–N was lower than 3 mg N kg−1 dry soil. Hence, no significant N2O emissions were observed in the field experiment before 35 DAT ()). Notably, not only NPK and NPK + Cover, also AGM + Cover treatment did not emit N2O before 35 DAT, implying that fresh AGM was decomposed and immobilized by soil microorganisms. Compared with NPK and NPK + Cover treatments, the low height of rice, tiller number, and leaf color in AGM + Cover treatment confirmed that N mineralization (NH4+-N production) from AGM was weak during the early rice growth stage, and, therefore less N2O emission was detected from AGM + Cover treatment since there was no surplus NH4+-N for nitrification or subsequent denitrification for N2O production.
Conversely, significant N2O emissions were detected from NPK + Cover and AGM + Cover treatments during the middle rice growth stage ()). We also observed the masses of dead Azolla cover in the two treatments’ surface water and soil interface. The result showed that the death and decomposition of the floating Azolla masses were attributed to high summer temperatures during the middle stages in the rice growth period in 2019 (, S1(b)). The rate of Azolla growth and biomass production depends on temperature, with ambient to optimal conditions of about 30°C considered favorable. However, short-term temperature increase above the optimum by about 2°C to 5°C, as well as extreme weather events, particularly in the summer period, dramatically impacts the productivity and survival of the Azolla (Lumpkin and Plucknett Citation1980; Wagner Citation1997). This study showed that the daily maximum temperatures during the middle rice growth stage were between 27.9°C and 33.6°C with a higher temperature range recorded at 56–77 DAT (32.5°C to 33.6°C) (Figure S1(b)).
Additionally, according to Wrage et al. (Citation2001), anoxic environments with sufficient labile organic C and nitrate (NO3−– N) release high quantities of N2O. In this study, although substrate availability was not limiting, lower concentrations of dissolved NO3−– N were detected in the pore-water samples for all treatments (Figure S4), compared with a previous report showing that low NO3−– N contents in lowland soil inhibit N2O reduction to N2 (Senbayram et al. Citation2012). Moreover, incomplete denitrification in mangrove sediments was attributed to 43%–93% of the N2O production (Fernandes et al. Citation2010). Therefore, while the presence of Azolla as a cover crop did not decrease seasonal N2O emissions as reported here, a better understanding of the relationship between leguminous cover crops presently promoted as a GHG mitigation strategy (particularly Azolla) and their effects on factors influencing N2O emissions are necessary as these effects are intricate and interact differently with diverse agricultural management practices (Eagle and Olander Citation2012).
4.3. Effect of Azolla application on GWP and Yield-scaled GWP emissions
In the present study, field application of Azolla either as dual cropping with rice (NPK + Cover) or both as dual cropping and incorporation (AGM + Cover) did not significantly influence the combined GWP (CH4 plus N2O emissions) compared with the NPK treatment (). However, AGM + Cover increased the combined GWP significantly by 33.0% compared with NPK + Cover treatment. This increase was attributed to the higher seasonal CH4 emissions from AGM + Cover treatment than NPK + Cover treatment (). Generally, the contribution of CH4 emissions to the combined GWP is more significant than that of N2O emissions, and effective CH4 emission management practices are essential toward the mitigation of GWP in rice paddies (Mosier et al. Citation2005; Hwang et al. Citation2017).
Accordingly, the application of Azolla as a dual crop along with rice did not significantly influence rice grain yield compared with NPK treatment. However, AGM + Cover significantly decreased rice grain yield by 37.5% (NPK) and 35.3% (NPK + Cover), with no significant harvest index differences observed among the three treatments (). Incorporating leguminous green manures in lowland paddies increases rice grain yield and N supply to the rice crop and favorable soil physical, chemical, and biological soil conditions (Bista and Dahal Citation2018). In this study, the low rice grain yield in AGM + Cover treatment was primarily affected by the low rice plant performance (i.e., low plant height and tiller numbers) during early rice growth stages (). This result indicated that fresh Azolla green manure applied a day before transplanting and its subsequent growth as a cover suppressed the growth of rice seedlings and tiller formation. Additionally, during the early rice growth stage, the leaf color (SPAD value) of AGM + Cover treatment was significantly lower than NPK and NPK + Cover, which implied that N nutrition was limited by the Azolla incorporation ()). Though the SPAD value of AGM + Cover treatment was higher than NPK and NPK + Cover toward the later stages of rice growth, the rice yield could not improve at harvest due to limited tiller number (). Azolla suppresses rice growth in the early stages by effectively outcompeting young rice plants for the applied chemical fertilizers and/or temporarily immobilizing available soil N during the initial decomposition (Wagner Citation1997; Basche et al. Citation2014). Moreover, the formation and accumulation of phytotoxic substances following the rapid anaerobic breakdown of incorporated organic matter, which coincides with the early rice growth stages, retard root elongation, shoot growth, and nutrient uptake by rice plants (Cannell and Lynch Citation1984).
Evaluation of the yield-scaled GWP emission is important in considering management options that can improve and sustain yield while reducing GHG emissions (Gao et al. Citation2016). Our results indicated that incorporating fresh Azolla as green manure a day before rice transplanting suppressed rice tiller formation during the early stage and decreased rice yield at last. Consequently, AGM + Cover significantly increased yield-scaled GWP by 96.0% and 104.2% compared with NPK and NPK + Cover treatments, respectively (). Overall, it is evident that the push to include alternative fertilizer sources, for example, Azolla as green manure, to address environmental safety ―herein to mitigate GHG emissions from conventionally flooded rice paddies― while maintaining a sustainable rice production, requires further understanding of the interaction of different Azolla application methods with various agricultural management practices.
The field results reported were characterized by, but not limited to, insufficient biological, chemical and physical soil changes data during the rice growth period. Additionally, the study site was a newly constructed field limited to one soil type under permanent flooding conditions. Further studies should therefore investigate primary changes in a range of soil types and the resultant changes in soil properties under different flooding scenarios. Additionally, studies should include different kinds of organic matter inputs under different management practices and environmental changes. This may significantly impact rice yield productivity and the production and consumption of CH4 and N2O gases in paddy ecosystems.
5. Conclusions
The application of Azolla either as a dual crop in conjunction with chemical fertilizer (NPK + Cover) or incorporated as green manure plus dual cropping (AGM + Cover) along with rice in the actual field did not significantly affect the cumulative CH4 emissions throughout the rice growth period compared with NPK treatment. Conversely, NPK + Cover and AGM + Cover treatments significantly increased cumulative N2O emissions at the middle rice growth stages and the total seasonal cumulative. This was partly attributed to higher summer air temperatures. Additionally, the AGM + Cover treatment significantly reduced grain yield by 37.5% and 35.3% compared with the NPK and NPK + Cover treatments, respectively, due to low tiller numbers, lack of balance between the plant N demand and green manure N supply, or retardation of root elongation, shoot growth and nutrient uptake by rice plants following the anaerobic decomposition of incorporated green manure. These observations were contrary to our previous pot observations that showed Azolla application as dual or green manure significantly reducing or increasing CH4 emissions, respectively, with no effect on N2O emissions or rice grain yield. Therefore, alternative application methods of fresh green manure are necessary to mitigate simultaneous CH4 and N2O emissions from lowland rice paddies while maintaining sustainable yield.
Supplemental Material
Download Zip (144.8 KB)Acknowledgments
First, we thank all members in the Soil Science and Plant Nutrition Laboratory (Faculty of Agriculture, Yamagata University) for their assistance in field and laboratory research managements. Secondly, we thank Mrs. Kazuhiro Nagasawa and Masashi Tanno of the Yamagata Integrated Agricultural Research Center for providing us with the gas measurements chambers. We also thank members of the Institute of Agro-Environmental Sciences, NARO, Tsukuba, Japan, for their assistance with gas analysis. This work was supported by a 2019 Student Research Support Fund from The United Graduate of Agricultural Sciences, Iwate University, Japan, and partly supported by Yamagata University YU-COE(C) programs of “Yamagata University Carbon Neutral Research Center (YUCaN)” and “Food, Agriculture, and Environmental Researches for SDGs.”
Disclosure statement
No potential conflict of interest was reported by the author(s).
Supplementary material
Supplemental data for this article can be accessed here.
References
- Anastasi, C., M. Dowding, and V. J. Simpson. 1992. “Future CH4 Emissions from Rice Production.” Journal of Geophysical Research 97 (D7): 7521–7525. doi:10.1029/92JD00157.
- Basche, A. D., F. E. Miguez, T. C. Kaspar, and M. J. Castellano. 2014. “Do Cover Crops Increase or Decrease Nitrous Oxide Emissions? A Meta-Analysis.” Journal of Soil and Water Conservation 69 (6): 471–482. doi:10.2489/jswc.69.6.471.
- Bharati, K., S. R. Mohanty, D. P. Singh, V. R. Rao, and T. K. Adhya. 2000. “Influence of Incorporation or Dual Cropping of Azolla on Methane Emission from a Flooded Alluvial Soil Planted to Rice in Eastern India.” Agriculture, Ecosystems & Environment 79 (1): 73–83. doi:10.1016/S0167-8809(99)00148-6.
- Bista, B., and S. Dahal. 2018. “Cementing the Organic Farming by Green Manures.” International Journal of Applied Sciences and Biotechnology 6 (2): 87–96. doi:10.3126/ijasbt.v6i2.20427.
- Butterbach-Bahl, K., E. M. Baggs, M. Dannenmann, R. Kiese, and S. Zechmeister-Boltenstern. 2013. “Nitrous Oxide Emissions from Soils: How Well Do We Understand the Processes and Their Controls?” Philosophical Transactions of the Royal Society B: Biological Sciences 368 (1621): 20130122. doi:10.1098/rstb.2013.0122.
- Cannell, R. Q., and J. M. Lynch. 1984. “Possible Adverse Effects of Decomposing Crop Residues on Plant Growth , and .” In Organic Matter and Rice, edited by F. N. Ponnamperuma and S. Banta, 455–475. Los Banos, Laguna, Philippines: International Rice Research Institute.
- Chen, G. X., G. Huang, B. Huang, J. Wu, K. W. Yu, H. Xu, X. Xue, and Z. Wang. 1995. “CH4 and N2O Emission from a Rice Field and Effect of Azolla and Fertilization on Them.” Chinese Journal of Applied Ecology 6 (4): 378–382.
- Chen, G. X., G. H. Huang, B. Huang, K. W. Yu, J. Wu, and H. Xu. 1997. “Nitrous Oxide and Methane Emissions from Soil–Plant Systems.” Nutrient Cycling in Agroecosystems 49 (1): 41–45. doi:10.1023/A:1009758900629.
- Cheng, W., H. Sakai, A. Hartley, K. Yagi, and T. Hasegawa. 2008. “Increased Night Temperature Reduces the Stimulatory Effect of Elevated Carbon Dioxide Concentration on Methane Emission from Rice Paddy Soil.” Global Change Biology 14 (3): 644–656. doi:10.1111/j.1365-2486.2007.01532.x.
- Cheng, W., H. Sakai, K. Yagi, and T. Hasegawa. 2009. “Interactions of Elevated [CO2] and Night Temperature on Rice Growth and Yield.” Agricultural and Forest Meteorology 149 (1): 51–58. doi:10.1016/j.agrformet.2008.07.006.
- Cheng, W., M. Takei, C. Sato, V. Kautsar, Y. Sasaki, S. Sato, K. Tawaraya, and H. Yasuda. 2015. “Combined Use of Azolla and Loach Suppressed Paddy Weeds and Increased Organic Rice Yield: Second Season Results.” Journal of Wetlands Environmental Management 3 (1): 1–13. doi:10.20527/jwem.v3i1.3.
- Cheng, W., K. Yagi, H. Sakai, and K. Kobayashi. 2006. “Effects of Elevated Atmospheric CO2 Concentrations on CH4 and N2O Emission from Rice Soil: An Experiment in Controlled-Environment Chambers.” Biogeochemistry 77 (3): 351–373. doi:10.1007/s10533-005-1534-2.
- Cheng, W., K. Yagi, H. Xu, H. Sakai, and K. Kobayashi. 2005. “Influence of Elevated Concentrations of Atmospheric CO2 on CH4 and CO2 Entrapped in Rice-Paddy Soil.” Chemical Geology 218 (1–2): 15–24. doi:10.1016/j.chemgeo.2005.01.016.
- Eagle, A. J., and L. P. Olander. 2012. “Greenhouse Gas Mitigation with Agricultural Land Management Activities in the United States—A Side-by-Side Comparison of Biophysical Potential.” Advances in Agronomy 115: 79–179. doi:10.1016/B978-012-394276-0.00003-2.
- Fernandes, S. O., P. A. Loka Bharathi, P. C. Bonin, and V. D. Michotey. 2010. “Denitrification: An Important Pathway for Nitrous Oxide Production in Tropical Mangrove Sediments (Goa, India).” Journal of Environmental Quality 39 (4): 1507–1516. doi:10.2134/jeq2009.0477.
- Gao, X., A. Lv, S. Wang, S. Liantai, P. Zhou, and Y. An. 2016. “Greenhouse Gas Intensity and Net Ecosystem Carbon Budget following the Application of Green Manures in Rice Paddies.” Nutrient Cycling in Agroecosystems 106 (2): 169–183. doi:10.1007/s10705-016-9797-7.
- Huang, Y., R. Sass, and F. Fisher. 1997. “Methane Emission from Texas Rice Paddy Soils. 2. Seasonal Contribution of Rice Biomass Production to CH4 Emission.” Global Change Biology 3 (6): 491–500. doi:10.1046/j.1365-2486.1997.00106.x.
- Hwang, H. Y., G. W. Kim, S. Y. Kim, M. M. Haque, M. I. Khan, and P. J. Kim. 2017. “Effect of Cover Cropping on the Net Global Warming Potential of Rice Paddy Soil.” Geoderma 292 (April): 49–58. doi:10.1016/j.geoderma.2017.01.001.
- IPCC. 2013. “Working Group I Contribution to the Fifth Assessment Report of the Intergovernmental Panel on Climate Change.” In Climate Change 2013 – The Physical Science Basis by Intergovernmental Panel on Climate Change, edited by T. F. Stocker, D. Qin, G. K. Plattner, M. Tignor, S. K. Allen, J. Boschung, A. Nauels, Y. Xia, V. Bex, and P. M. Midgley, 1535. Cambridge, UK and New York, NY, USA: Cambridge University Press. doi:10.1017/CBO9781107415324.
- Itoh, M., S. Sudo, S. Mori, H. Saito, T. Yoshida, Y. Shiratori, S. Suga, et al. 2011. “Mitigation of Methane Emissions from Paddy Fields by Prolonging Midseason Drainage.” Agriculture, Ecosystems & Environment 141 (3–4): 359–372. doi:10.1016/j.agee.2011.03.019.
- JSSSPN. 1986. Soil Normal Analysis Methods, Hakuyusha Press, Tokyo (In Japanese). Tokyo: Japanese Society of Soil Science and Plant Nutrition.
- Kimani, S. M., P. O. Bimantara, S. Hattori, K. Tawaraya, S. Sudo, and W. Cheng. 2020. “Azolla Incorporation and Dual Cropping Influences CH4 and N2O Emissions from Flooded Paddy Ecosystems.” Soil Science and Plant Nutrition 66 (1): 152–162. doi:10.1080/00380768.2019.1705736.
- Kimani, S. M., W. Cheng, T. Kanno, T. Nguyen-Sy, R. Abe, A. Z. Oo, K. Tawaraya, and S. Sudo. 2018. “Azolla Cover Significantly Decreased CH4 but Not N2O Emissions from Flooding Rice Paddy to Atmosphere.” Soil Science and Plant Nutrition 64 (1): 68–76. doi:10.1080/00380768.2017.1399775.
- Kimura, M., Y. Miura, A. Watanabe, J. Murase, and S. Kuwatsuka. 1992. “Methane Production and Its Fate in Paddy Fields.” Soil Science and Plant Nutrition 38 (4): 665–672. doi:10.1080/00380768.1992.10416696.
- Kollah, B., A. K. Patra, and S. R. Mohanty. 2016. “Aquatic Microphylla Azolla: A Perspective Paradigm for Sustainable Agriculture, Environment and Global Climate Change.” Environmental Science and Pollution Research 23 (5): 4358–4369. doi:10.1007/s11356-015-5857-9.
- Li, C. 2007. “Quantifying Greenhouse Gas Emissions from Soils: Scientific Basis and Modeling Approach.” Soil Science & Plant Nutrition 53 (4): 344–352. doi:10.1111/j.1747-0765.2007.00133.x.
- Lumpkin, T. A., and D. L. Plucknett. 1980. “Azolla: Botany, Physiology, and Use as a Green Manure.” Economic Botany 34 (2): 111–153. doi:10.1007/BF02858627.
- Malyan, S. K., A. Bhatia, A. Kumar, D. K. Gupta, R. Singh, S. S. Kumar, R. Tomer, O. Kumar, and N. Jain. 2016. “Methane Production, Oxidation and Mitigation: A Mechanistic Understanding and Comprehensive Evaluation of Influencing Factors.” Science of the Total Environment 572 (December): 874–896. doi:10.1016/j.scitotenv.2016.07.182.
- Minamikawa, K., T. Tokida, S. Sudo, and A. Chidthaisong. 2015. Guidelines for Measuring CH4 and N2O Emissions from Rice Paddies by a Manually Operated Closed Chamber Method. Tsukuba, Japan: National Institute for Agro-Environmental Sciences.
- Mosier, A. R., A. D. Halvorson, G. A. Peterson, G. P. Robertson, and L. Sherrod. 2005. “Measurement of Net Global Warming Potential in Three Agroecosystems.” Nutrient Cycling in Agroecosystems 72 (1): 67–76. doi:10.1007/s10705-004-7356-0.
- Oertel, C., J. Matschullat, K. Zurba, F. Zimmermann, and S. Erasmi. 2016. “Greenhouse Gas Emissions from Soils—A Review.” Geochemistry 76 (3): 327–352. doi:10.1016/j.chemer.2016.04.002.
- Scheehle, E., and D. Kruger. 2006. “Global Anthropogenic Methane and Nitrous Oxide Emissions.” The Energy Journal 27 ( Special Issue #3): 33–44. doi:10.5547/0195-6574-EJ-VolSI2006-NoSI3-2.
- Seck, P. A., A. Diagne, S. Mohanty, and M. C. S. Wopereis. 2012. “Crops that Feed the World 7: Rice.” Food Security 4 (1): 7–24. doi:10.1007/s12571-012-0168-1.
- Senbayram, M., R. Chen, A. Budai, L. Bakken, and K. Dittert. 2012. “N2O Emission and the N2O/(N2O+N2) Product Ratio of Denitrification as Controlled by Available Carbon Substrates and Nitrate Concentrations.” Agriculture, Ecosystems & Environment 147 (January): 4–12. doi:10.1016/j.agee.2011.06.022.
- Snyder, C. S., T. W. Bruulsema, T. L. Jensen, and P. E. Fixen. 2009. “Review of Greenhouse Gas Emissions from Crop Production Systems and Fertilizer Management Effects.” Agriculture, Ecosystems & Environment 133 (3): 247–266. doi:10.1016/j.agee.2009.04.021.
- Sudo, S. 2006. “Method and Instrument for Measuring Atmospheric Gas.” Industrial Property Digital Library, Patent of Japan (No. 2006–275844).
- van der Gon, H. A. C. D., and H. U. Neue. 1995. “Influence of Organic Matter Incorporation on the Methane Emission from a Wetland Rice Field.” Global Biogeochemical Cycles 9 (1): 11–22. doi:10.1029/94GB03197.
- Wagner, G. M. 1997. “Azolla: A Review of Its Biology and Utilization.” The Botanical Review 63 (1): 1–26. doi:10.1007/BF02857915.
- Watanabe, I., W. Ventura, G. Mascariña, and D. L. Eskew. 1989. “Fate of Azolla Spp. And Urea Nitrogen Applied to Wetland Rice (Oryza Sativa L.).” Biology and Fertility of Soils 8 (2): 102–110. doi:10.1007/BF00257752.
- Wrage, N., G. L. Velthof, M. L. van Beusichem, and O. Oenema. 2001. “Role of Nitrifier Denitrification in the Production of Nitrous Oxide.” Soil Biology & Biochemistry 33 (12–13): 1723–1732. doi:10.1016/S0038-0717(01)00096-7.
- Xu, H., B. Zhu, J. Liu, D. Li, Y. Yang, K. Zhang, Y. Jiang, Y. Hu, and Z. Zeng. 2017. “Azolla Planting Reduces Methane Emission and Nitrogen Fertilizer Application in Double Rice Cropping System in Southern China.” Agronomy for Sustainable Development 37 (4): 29. doi:10.1007/s13593-017-0440-z.
- Yadav, R. K., G. Abraham, Y. V. Singh, and P. K. Singh. 2014. “Advancements in the Utilization of Azolla-Anabaena System in Relation to Sustainable Agricultural Practices.” Proceedings of the Indian National Science Academy 80 (2): 301–316. doi:10.16943/ptinsa/2014/v80i2/55108.
- Yagi, K., and K. Minami. 1990. “Effect of Organic Matter Application on Methane Emission from Some Japanese Paddy Fields.” Soil Science and Plant Nutrition 36 (4): 599–610. doi:10.1080/00380768.1990.10416797.
- Ying, Z., P. Boeckx, G. X. Chen, and O. Van Cleemput. 2000. “Influence of Azolla on CH4 Emission from Rice Fields.” Nutrient Cycling in Agroecosystems 58 (1–3): 321–326. doi:10.1023/A:1009871308968.
- Zou, J., Y. Huang, Y. Qin, S. Liu, Q. Shen, G. Pan, Y. Lu, and Q. Liu. 2009. “Changes in Fertilizer-Induced Direct N2O Emissions from Paddy Fields during Rice-Growing Season in China between 1950s and 1990s.” Global Change Biology 15 (1): 229–242. doi:10.1111/j.1365-2486.2008.01775.x.