ABSTRACT
Ammonium nitrogen (NH4+-N) content in soil is a key factor affecting nitrous oxide (N2O) emissions due to its role as a primary substrate of nitrification. This study aimed at investigating the effects of different application rates of NH4+-N on N2O and CO2 emissions from two different types of manure compost-amended soil, along with analysis of relative abundances of narG and nosZ genes under aerobic conditions. Laboratory experiments were conducted using Kochi and Ushimado soils amended with mixed compost (MC: mixture of cattle, poultry, and swine manure) or cattle manure compost (CC) at 3% (dry weight basis). In no compost- and compost-amended soils, (NH4)2SO4 was added as a solution equivalent to 160, 200, and 400 mg-N kg−1 of soil. Soil samples were aerobically incubated at 70% water-holding capacity (WHC) and 25°C. Emissions of N2O and CO2 were measured on days 0, 3, 7, 15, 21, 28, and 42. The abundances of narG and nosZ genes in Kochi (day 7) and Ushimado (day 21) soils were estimated using qPCR tests. Emissions of N2O and CO2 were higher in MC-amended soil because of higher mineral N content and lower C/N ratio of MC than those of CC, regardless of NH4+-N rates. Emissions of N2O and CO2 were higher in compost-amended Kochi soil due to higher mineral N, total N and C, and clay contents, and possibly because of higher water-filled pore spaces than those in Ushimado soil at the same WHC. In both soils with CC and no compost, raising NH4+-N rate from 160 to 200 increased N2O emissions due to stimulation of nitrification. In contrast, increasing NH4+-N rate from 200 to 400 decreased N2O and CO2 emissions except for N2O emissions in MC- and CO2 emissions in CC- and no compost-amended Ushimado soil possibly due to osmotic stress on microorganisms and limited C availability. Emissions of N2O were positively related to narG gene copy numbers in Kochi soil (R2 = 0.78) due to high N and C contents. Our study revealed that NH4+-N rate 400 suppresses N2O and CO2 emissions from manure compost-amended soil under aerobic conditions.
1. Introduction
Nitrous oxide (N2O) is a major greenhouse gas with significant global warming potential and direct impacts on stratospheric ozone depletion (Deng et al. Citation2016). The main anthropogenic processes producing N2O emissions include the application of organic and inorganic fertilizers containing nitrogen (N) to agricultural soils and combustion of fossil fuels (Signor and Cerri Citation2013). In agricultural soils, biological N-fixation, crop residue, and sewage sludge are other sources of N, which can have profound impacts on N2O emissions (Mosier et al. Citation1998). Emissions of N2O have increased by 30% over the past four decades to 7.3 Tg-N per year, and two-thirds of total anthropogenic N2O emissions have come from agricultural soils (Tian et al. Citation2020; Wang et al. Citation2021).
N2O in soil is produced by microbial transformation of mineral N (ammonium N: NH4+-N and nitrate N: NO3−-N) through a series of processes of nitrification and denitrification (Deng et al. Citation2016). Nitrification is an aerobic process that is primarily regulated by autotrophic and aerobic bacteria (ammonia- and nitrite-oxidizing bacteria) to oxidize ammonium to NO3− (Parton et al. Citation2001). Denitrification is a chained reductive reaction of NO3− to N2, generating intermediate products of NO2−, NO, and N2O under anaerobic conditions by a wide range of heterotrophic bacteria, fungi, and archaea (Otte et al. Citation2019) and considered to be a main N2O formation pathway in soils (Sanchez-Garcia et al. Citation2014). In addition, there is increasing evidence that aerobic denitrification may play an important role when certain bacteria perform NO3− respiration in the presence of O2 (Bateman and Baggs Citation2005). In general, an abundance of nosZ-bearing bacteria promotes the reduction of N2O to N2, thereby decreasing the overall emissions of N2O, while narG, which encodes membrane-bound NO3− reductase is responsible for increased N2O emissions (Nie et al. Citation2016; Lazcano, Zhu-Barker, and Decock Citation2021).
Factors such as moisture, temperature, mineral N, decomposable organic matter, microorganism populations, soil texture, pH, and drainage capacity affect the production and emissions of N2O (Boeckx, Beheydt, and Cleemput Citation2005; Signor and Cerri Citation2013). The effects of moisture, temperature, and organic matter on N2O emissions have been examined as follows. Soil temperature and water content directly affect the production and consumption of N2O through their effects on the metabolic activity of microorganisms (Luo et al. Citation2013). A combined effect of moisture and temperature was explored by Kurganova and de Gerenyu (Citation2010), who stated that N2O emission rates from wet soils increased significantly in temperatures ranging from 10 to 15°C and from 20 to 25°C. Nitrification and denitrification processes are directly affected by organic matter, and therefore influence N2O emissions (Mosier Citation1994). Soil texture and drainage capacity affect gas diffusivity controlling the availability of O2 in soil for microbial processes, consequently influencing N2O emissions (Zhu et al. Citation2020).
The application of mineral N affects nitrification and denitrification, and thereby N2O emissions (Dobbie and Smith Citation2003) and NH4+-N is a preferred N form for most bacteria and fungi (Muller et al. Citation2006). Relatively higher N2O emissions were observed from ammonium sulphate [(NH4)2SO4]-amended arable soil due to the stimulation of nitrification (Velthof, Kuikman, and Oenema Citation2003). Hoang and Maeda (Citation2018) studied the interaction of NH4+-N and temperatures and found that NH4+-N application at high temperatures suppressed N2O emissions. Darrah, White, and Nye (Citation1986) investigated physiological and microbiological changes in a fine sandy loam soil following the application of ammonium salts and observed inhibited nitrification due to enhanced osmotic pressure. Effects of osmotic potential on N2O emissions were explored by Low, Stark, and Lynn (Citation1997) under elevated ammonium contents in a sandy loam soil. Microbial immobilization of N was observed by Sun et al. (Citation2016) under the application of ammonium fertilizer at an annual rate of 50 kg-N ha−1 year−1. Other studies discussed the effects of NH4+-N derived from organic amendment on N2O emissions. Kim et al. (Citation2019) observed a positive relationship between daily N2O emissions and soil contents of NH4+-N derived from animal manure. Similarly, Li et al. (Citation2017) found a positive correlation between N2O emissions and NH4+-N contents of cattle manure-amended soil. All these studies reported that a single application of mineral N fertilizer or organic amendment significantly affected N2O emissions.
However, N2O emissions can be caused by the combined application of ammonium fertilizer and organic amendment because NH4+-N is derived from both inorganic fertilizer and the organic amendment. Katoh, Hayashi, and Morikuni (Citation2008) and Jin et al. (Citation2010) observed increased NH4+-N contents following the combined application of ammonium fertilizer and cattle manure compost. The addition of mineral N fertilizer to composted swine manure-amended soil induced N2O emissions 2.9 times higher compared with mineral N fertilizer alone due to higher availability of N and labile organic carbon (C) for nitrifiers and denitrifiers (Qiao et al. Citation2014). In contrast, Liu et al. (Citation2020) found that the combined application of 25% raw manure-N plus 75% of NH4+-N mitigated N2O emissions with compared to a single application of ammonium fertilizer, but no yield reductions occurred in a maize and wheat rotation system. Cai, Ding, and Luo (Citation2013) also observed decreased N2O emissions after the application of compost (a mixture of wheat straw, oil cake, and cotton cake) and inorganic N fertilizer together because of the lower amount of mineral N released during the decomposition of compost. Apart from the contradictions in available studies, many of them were field experiments conducted on a single type of soil and did not compare the impacts of NH4+-N applications on different types of soil. Therefore, the effects of the addition of NH4+-N to different types of soil amended with manure compost remain uncertain.
Organic soil amendment can impact C and N dynamics to promote soil CO2 emissions (Grave et al. Citation2015). Ray et al. (Citation2020) found higher cumulative CO2 emissions from soils amended with chicken manure than those amended with cow manure. In contrast, Termorshuizen et al. (Citation2004) found that C in compost can be sequestered for a long time because labile organic compounds can be protected by hydrophobic humic substances, resulting in reduced CO2 emissions. Sainju et al. (Citation2012) found that urea-N application increased CO2 emissions from soil because of increases in the crop yield and the residue in soil. Sosulski et al. (Citation2020) suggested that NH4+-N increased CO2 emissions from soil as a result of availability of N for decomposers of soil organic matter. Ding et al. (Citation2007) revealed that N fertilization resulted in the reduction of CO2 emissions from soil. Gagnon et al. (Citation2016) found that the application of nitrate fertilizer at a rate of 150 kg-N ha−1 reduced CO2 emissions by 22% than those of ammonium fertilizer. A single application of urea had no significant effect on soil CO2 emissions in an experiment done by Lin et al. (Citation2021), indicating that oilseed rape cake was the main source of CO2 production in the combined application with urea. However, more detailed information about the effects of NH4+-N on CO2 emissions from manure compost-amended soil is rarely available.
Studies discussing the effects of NH4+-N additions on N2O and CO2 emissions from manure compost-amended soil are mandatory to establish strategies to mitigate N2O and CO2 emissions from fertilized agricultural soil. Furthermore, the relation of key denitrifying microbial genes to N2O emissions from soil amended with manure compost and supplemented with NH4+-N has not been analyzed in detail. Therefore, the objective of this study was to investigate the effects of different application rates of NH4+-N on N2O and CO2 emissions from two contrasting types of manure compost-amended soil under aerobic conditions. Further, we analyzed the relative abundances of narG and nosZ denitrifying genes responsible for N2O emissions from manure compost-amended soil. These two genes were selected for the analyses because they are the key genes controlling N2O or N2 emissions via NO3− reduction to N2O or N2O reduction to N2.
2. Materials and methods
2.1. Soil sampling and preparation
Kochi and Ushimado soils with contrasting initial properties were used for laboratory incubation experiments under aerobic conditions. Kochi soil was collected from a vegetable greenhouse at the Faculty of Agriculture and Marine Science, Kochi University, Japan, in April, 2019. Before soil sampling, 18 kg m−2 of bark compost was applied to the field for the last five years. Ushimado soil was collected from a paddy field in Ushimado, Okayama Prefecture, Japan, in April, 2016, in which no manure or compost had been amended at least in the past five years. Kochi and Ushimado soils belong to eutric fluvisol and dystric gleysol soil groups (FAO), respectively (Cultivated soil classification committee Citation1995). Soil samples were air-dried, passed through a 2-mm sieve, and stored until the experiments. Soil texture and cation exchange capacity (CEC) were determined by the sieve and pipette method (Kettler, Doran, and Gilbert Citation2001) and the Schollenberger method at pH 7 (Sparks et al. Citation1996), respectively. Total N and C contents in soil were measured by the dry combustion method with a CN coder (MT-700, Yanaco, Japan). Kochi soil showed higher electrical conductivity (EC), CEC, and total N, C, and mineral N contents compared to Ushimado soil. Kochi soil was a sandy clay loam and Ushimado soil was a sandy loam in texture (). These two soil types were selected to reflect the effects of different properties of soil (total C, N, and mineral N contents) on N2O and CO2 emissions after being amended with manure compost at different NH4+-N rates.
Table 1. Initial properties of two types of soil and compost.
2.2. Manure compost collection and preparation
Two types of livestock manure compost, cattle manure compost (CC) and mixed compost (MC) were used as soil amendments. The CC containing sawdust was produced at the Research Institute for Livestock Science, Okayama Prefectural Agriculture, Forestry and Fisheries Research Center. The MC (a mixture of cattle, poultry, and swine manure at a rate of 6: 3: 1 on weight basis supplemented with sawdust, rice husk, and bark during the composting process) was produced at the Tetta Town Composting Center, Okayama. Both CC and MC were air-dried and ground into fine powders to assure uniform distribution in soils. Total N and mineral N contents were higher, and the total C content was slightly lower in MC than those in CC (). The higher C/N ratio and lower N contents of CC than those of MC indicate that CC was more resistant to decomposition and N mineralization.
2.3. Laboratory incubation experiments
Kochi and Ushimado soils were uniformly mixed with two compost types separately at 3% (by weight), considering a common organic matter application rate (approximately 30 Mg ha−1) to agricultural soils. No compost-amended control soil was used as a reference for the comparisons with compost-amended samples in each soil. The application rates of NH4+-N to CC-, MC-, and no compost-amended soil were adjusted to 160, 200, and 400 mg-N kg−1 of soil (corresponding to 160, 200, and 400 kg-N ha−1, respectively) using (NH4)2SO4 as a solution, except Kochi soil, which was amended with MC at 160, the original NH4+-N content. These application rates are common for fertilized greenhouse vegetables in Japan (Nishio Citation2001). Moisture contents of all treatments were adjusted to 70% water-holding capacity (WHC) (equivalent to water-filled pore space (WFPS) 69–72% for Kochi soil and 44–46% for Ushimado soil, which differed due to bulk densities and water absorption by compost particles). The initial WHC levels were maintained during the incubation period by adding deionized water to compensate the loss by evaporation. The treatments were named to K, KCC, and KMC for no compost-, CC-, and MC-amended Kochi soil and U, UCC, and UMC for no compost-, CC-, and MC-amended Ushimado soil followed by NH4+-N rates 160, 200, and 400 (−160, −200, and −400).
The experiment consisted of 18 treatments as described above in triplicate (n=3). Soil samples (10 g) were contained in 100-mL glass bottles covered with polyethylene film (0.02 mm thickness) to minimize evaporation, while maintaining gas exchange. The bottles were incubated at 25°C for 42 days and N2O and CO2 fluxes were determined on days 0 (incubation start date), 3, 7, 15, 21, 28, and 42.
2.4. Gas sampling and flux measurement
The incubation bottles were flushed for two minutes with atmospheric air at 50 mL s−1 with a mini-pump (MP-2 N, Shibata, Japan) to exchange gas inside the bottles with the ambient air prior to collecting gas samples. The bottles were sealed with butyl rubber septa tightened with aluminum caps for 2 h before collecting gas samples for N2O and CO2 measurements. Head space gas samples were injected to gas chromatographs (GC-8A, Shimadzu, Japan) equipped with an electron capture or thermal conductivity detector for N2O and CO2, respectively. The gas concentration was determined with respect to a calibration done using two standard gas samples separately for N2O (1.512×10−6 and 0.298×10−6 or 20.67×10−6 m3 m−3) and CO2 (0.35×10−3 and 2.19×10−3 m3 m−3). Gas emissions were calculated using the following equation:
N2O or CO2 emissions (μg-N or -C kg−1 h−1) = ρ×C×10−6×(Vg+VL×α)×273/(W×(273+T))/t
where ρ is the density of N2O-N (1.25 kg m−3) or CO2-C (0.5357 kg m−3), C (m3 m−3) is the concentration of N2O or CO2, Vg (m3) is the volume of the headspace, VL (m3) is the volume of the liquid phase, α is the Bunsen absorption coefficients for N2O (0.539) and CO2 (0.614) at 25°C, W (kg) is the oven-dried weight of soil, T is the incubation temperature (25°C), and t is an incubation period (2 h). Concentrations of N2O and CO2 in the ambient air were deducted from each measured concentration.
2.5. DNA extraction and quantification of relative abundances of narG and nosZ genes
The functional genes, narG and nosZ, were analyzed to identify the relation between relative abundances of those genes and N2O emissions from compost-amended soil under aerobic conditions. The samples on day 7 of Kochi soil and day 21 of Ushimado soil treatments were used for DNA and functional gene analysis. These sampling days were selected because maximum N2O emissions appeared within one and three weeks of incubation in Kochi and Ushimado soils, respectively. In addition, the original Kochi and Ushimado soils were also analyzed for reference.
Soil DNA was extracted from 0.4 g of moist soil (triplicates) using a DNA extraction kit (FastDNA Spin Kit for Soil, MP Biomedicals, USA). DNA Clean and ConcentratorTM-25 (Zymo Research, USA) was used to purify the eluted DNA (80 µL). Purified DNA was quantified using a spectrophotometer (NanoDrop One, Thermo Scientific, Japan).
For quantification of narG and nosZ functional genes, a qPCR system (Step One Plus Real-Time PCR, Applied Biosystems, USA) was used. Purified soil DNA samples were subjected to duplicate independent amplifications and genes were quantified according to the method described by Henry et al. (Citation2006). The primer pair narG 1960 m-2F, narG 2050 m-2R and nosZ-2F, nosZ-2R were used for quantification of narG and nosZ genes, respectively. The reaction mixture (20 µL) for narG or nosZ genes contained 10 µL of qPCR master mix (KAPA SYBR FAST), 1 or 2 µL of each primer, 2 or 1 µL of hundredfold-diluted soil DNA, and 6 or 5 µL of autoclaved ultra-pure water. Thermo-cycling quantitative PCR reaction conditions for narG genes were as follows: enzyme activation of 15 minutes at 95°C; 40 cycles of 15s at 95°C for denaturing, 30s at 58°C for annealing, 30s at 72°C for extension, and 15s at 81°C for final extension. Thermo-cycling conditions for nosZ genes were similar except the enzyme activation of 10 minutes, annealing of 15s at 60°C, and no final extension. The standard curves for quantification of narG and nosZ genes were generated using Pseudomonas stutzeri NBRC 14165.
2.6. Mineral N, pH, and EC of incubated soil
Soil samples in bottles after gas collections (1:10), the original soil (1:10), and compost samples (1:100) were extracted with 2 mol L−1 potassium chloride by shaking at 175 rpm for 1 h, then used to measure NH4+-N (the modified indophenol method) and NO3−N (the cadmium reduction method) contents using a continuous flow analyzer (QuAAtro 2-HR, Bltec, Japan, Eaton et al. Citation2005). The pH (H2O) and EC of water extractions (1:10) of samples were measured using a pH/Ion meter (F-23, Horiba, Japan) and conductivity meter (DS-14, Horiba, Japan), respectively after shaking at 175 rpm for 1 min (Hoang and Maeda Citation2018).
2.7. Data analysis
Cumulative N2O and CO2 emissions were calculated using trapezoidal integration of N2O and CO2 fluxes over time. Statistically significant differences in cumulative N2O and CO2 emissions among no compost- and compost-amended treatments at three NH4+-N rates were examined separately for two types of soil using analysis of variance (two-way ANOVA: compost type×NH4+-N rates, p <0.05, ) and Tukey test. Three-way ANOVA (compost type×soil type×NH4+-N rates, ) was performed on cumulative N2O and CO2 emissions over 42 days. All statistical calculations were performed using the R software (R Core Team Citation2019).
Table 2. Cumulative N2O and CO2 emissions in the incubation experiment.
Table 3. Responses of cumulative N2O and CO2 emissions to compost type, soil type, and NH4+-N rates by three-way ANOVA.
3. Results
3.1. Emissions of N2O from compost-amended soil at different NH4+-N rates
In no compost-amended Kochi soil (K-160, K-200, and K-400 treatments), peak N2O emissions appeared on day 3, then decreased gradually to day 42 (). The lowest and highest peak N2O emissions were seen in K-160 and K-200 treatments, respectively. In CC-amended Kochi soil (KCC-160, KCC-200, and KCC-400 treatments), the same pattern of N2O emissions as in no-compost treatments was observed (). The highest peak emission of N2O was in the KCC-200 treatment, while other treatments showed similar peak N2O emissions. In KMC-200 and KMC-400 treatments, peak N2O emissions appeared on day 3, whereas in the KMC-160 treatment, the peak appeared on day 7 (). The peak N2O emission in the KMC-400 treatment was significantly lower than that in the KMC-200 treatment (p <0.05). All N2O emissions gradually decreased with time except in the KMC-200 treatment, which had an intermediate peak emission on day 14. Among all Kochi soil treatments, MC-amended treatments had higher N2O emissions than those amended with CC and no compost irrespective of NH4+-N rates.
Figure 1. N2O emissions in the incubation experiment.The left (a)–(c) show results for Kochi soil (K) and the right (d)–(f) do for Ushimado soil (U). The upper (a) and (d) show results for no compost-, the middle (b) and (e) for cattle manure compost (CC)-, and the lower (c) and (f) for mixed compost (MC)-amended soils. The numbers (160, 200, and 400) indicate NH4+-N application rates to soil (mg-N kg−1). Error bars indicate ± standard deviation (n=3).
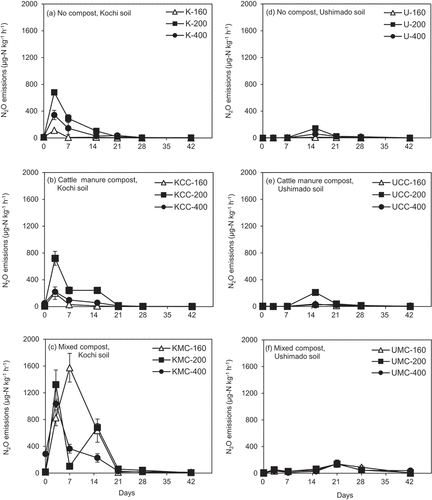
In no compost- and CC-amended treatments of Ushimado soil, peak N2O emissions appeared on day 14 (), where the highest peak N2O emissions were in U-200 and UCC-200 treatments. In MC-amended Ushimado soil, all peak N2O emissions appeared on day 21 and the smaller peaks on day 3 (). The peak heights in MC-amended Ushimado soil were similar irrespective of NH4+-N rates. When comparing two soil types, Kochi soil treatments had the highest temporal N2O emissions compared with Ushimado soil treatments ().
In no compost- and CC-amended Kochi soil, cumulative N2O emissions were greater in the order of K-200, KCC-200 > K-400, KCC-400 > K-160, KCC-160 (). Cumulative N2O emissions in MC-amended Kochi soil decreased gradually from NH4+-N rate 160 (KMC-160) to 400 (KMC-400) (p <0.05). In summary, cumulative N2O emissions in Kochi soil decreased when the NH4+-N rate increased from 200 to 400. Interestingly, in Ushimado soil, the same trends as in Kochi soil were observed at different NH4+-N rates, whereas in the UMC-200 treatment, cumulative N2O emission was not significantly different from those in UMC-160 and UMC-400 treatments (p >0.05, ). According to three-way ANOVA results, the interaction effects among compost type, soil type, and NH4+-N rates on cumulative N2O emissions were significant (p <0.05) in addition to their individual effects ().
3.2. Emissions of CO2 from compost-amended soil at different NH4+-N rates
In Kochi soil, peak CO2 emissions occurred within the first week of incubation, then decreased with time (). The MC-amended Kochi soil () showed the highest CO2 emissions compared with no compost- and CC-amended treatments irrespective of NH4+-N rates. In Ushimado soil, CO2 emissions showed similar patterns, which were slightly lower than those in corresponding Kochi soil treatments ().
Figure 2. CO2 emissions in the incubation experiment.The left (a)–(c) show results for Kochi soil (K) and the right (d)–(f) do for Ushimado soil (U). The upper (a) and (d) show results for no compost-, the middle (b) and (e) for cattle manure compost (CC)-, and the lower (c) and (f) for mixed compost (MC)-amended soils. The numbers (160, 200, and 400) indicate NH4+-N application rates to soil (mg-N kg−1). Error bars indicate ± standard deviation (n=3).
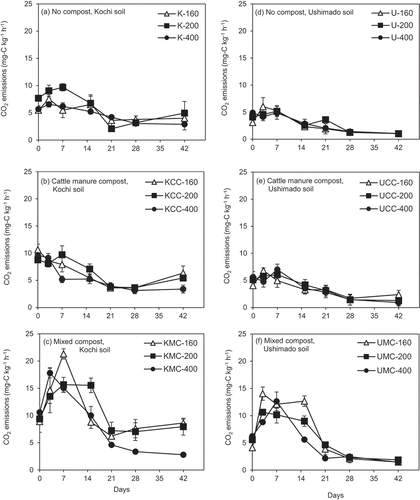
In Kochi soil, cumulative CO2 emissions at NH4+-N rate 160 were the same level as those at NH4+-N rate 200, while those at NH4+-N rate 400 were significantly lower than those at NH4+-N rate 200 (p <0.05, ). In Ushimado soil, significant differences in cumulative CO2 emissions across NH4+-N rates were observed only when amended with MC (). In those treatments, cumulative CO2 emissions were significantly higher in the order of UMC-160 > UMC-200 > UMC-400 (p <0.05). The individual effects of compost type, soil type, and NH4+-N rates on cumulative CO2 emissions were significant (p <0.001, ), whereas the interactions between three factors were not significant (p >0.05, ).
3.3. Soil NH4+-N and NO3−-N contents
In Kochi soil, NH4+-N contents increased until day 7 (K-200 and KMC-160 treatments) or 3 (the other treatments), then decreased towards day 42 (). Soil NH4+-N contents were almost extinct around day 21 in all treatments. The initial increment of NH4+-N contents was not prominent in the KMC-400 treatment, whereas the drastic reduction after day 3 was similar to the other treatments ().
Figure 3. NH4+-N contents in the incubation experiment.The left (a)–(c) show results for Kochi soil (K) and the right (d)–(f) do for Ushimado soil (U). The upper (a) and (d) show results for no compost-, the middle (b) and (e) for cattle manure compost (CC)-, and the lower (c) and (f) for mixed compost (MC)-amended soils. The numbers (160, 200, and 400) indicate NH4+-N application rates to soil (mg-N kg−1). Error bars indicate ± standard deviation (n=3).
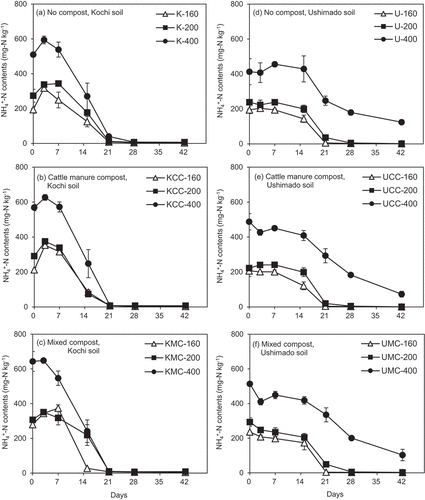
In Ushimado soil, the initial increment of NH4+-N contents was not clear. In no compost- and CC-amended treatments, NH4+-N contents started decreasing on day 7 (). In MC-amended treatments, NH4+-N contents tended to decrease from the beginning of the incubation (). Soil NH4+-N contents decreased rapidly from day 14 to day 21 and became extinct around day 21 at NH4+-N rates 160 and 200 as seen in Kochi soil. At NH4+-N rate 400 (U-400, UCC-400, and UMC-400 treatments), NH4+-N contents continuously decreased and remained between 100 and 200 mg-N kg−1 at the end of the experiment.
In Kochi soil, NO3−-N contents decreased until day 3 or 7, then started increasing with time in response to the reduction of NH4+-N contents (). In the KMC-160 treatment, the reduction in NO3−-N content from the beginning to day 7 was more prominent than those in the other treatments. After day 21 or 28, the values became flattened. In the KMC-160 treatment, a reduction in NO3−-N contents was observed from day 21 to 28 (p <0.05, ), indicating the possibility of denitrification during this period. The lowest NO3−-N content among all treatments was also recorded in the KMC-160 treatment.
Figure 4. NO3−-N contents in the incubation experiment.The left (a)–(c) show results for Kochi soil (K) and the right (d)–(f) do for Ushimado soil (U). The upper (a) and (d) show results for no compost-, the middle (b) and (e) for cattle manure compost (CC)-, and the lower (c) and (f) for mixed compost (MC)-amended soils. The numbers (160, 200, and 400) indicate NH4+-N application rates to soil (mg-N kg−1). Error bars indicate ± standard deviation (n=3).
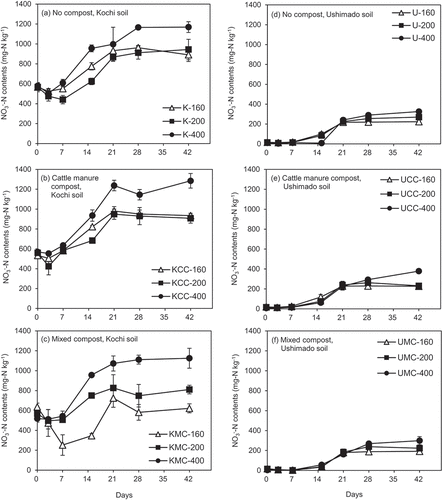
In Ushimado soil, NO3−-N contents were lower and the differences among treatments were smaller than those in Kochi soil (). All treatments showed increasing NO3−-N contents starting from day 7. At NH4+-N rates 160 and 200, NO3−-N contents became flattened after day 21. Soil NO3−-N contents at NH4+-N rate 400 increased to the end of the incubation (especially in the UCC-400 treatment).
3.4. Soil pH and EC
In Kochi soil, the pH range (5.5–6.6) was slightly lower than that in Ushimado soil (6.6–8.4) during the incubation (). In both soils, pH values did not vary considerably among treatments throughout the entire period and pH slightly increased or was stable until day 7, and then continuously decreased. The slight increase of pH was probably caused by denitrification, which was corresponded to the reduction of NO3−-N contents. The decrease of pH was more prominent in Ushimado soil () than that in Kochi soil (). This trend corresponded to the decreased NH4+-N contents () and increased NO3−-N contents () after day 7, suggesting that the decrease of pH was due to nitrification.
Figure 5. pH (H2O) values in the incubation experiment.The left (a)–(c) show results for Kochi soil (K) and the right (d)–(f) do for Ushimado soil (U). The upper (a) and (d) show results for no compost-, the middle (b) and (e) for cattle manure compost (CC)-, and the lower (c) and (f) for mixed compost (MC)-amended soils. The numbers (160, 200, and 400) indicate NH4+-N application rates to soil (mg-N kg−1). Error bars indicate ± standard deviation (n=3).
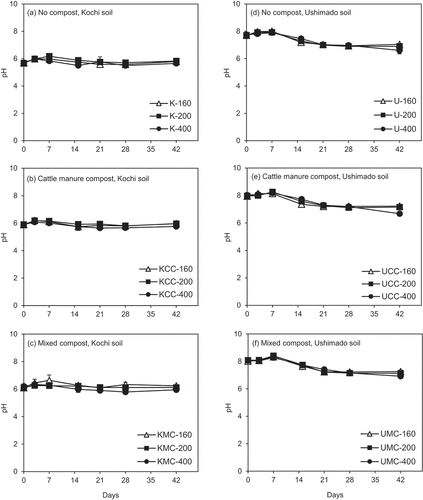
In Kochi soil, the EC range (0.96–1.7 dS m−1) was higher than that in Ushimado soil (0.2–0.77 dS m−1)during the incubation (). In Kochi soil, the highest EC values were observed at NH4+-N rate 400 (K-400, KCC-400, and KMC-400 treatments, ), where the values increased slightly until day 42. In no compost- and CC-amended Kochi soil, clear differences in EC among NH4+-N rates 160 and 200 were not seen. In MC-amended Kochi soil, the lowest EC was observed in the KMC-160 treatment.
Figure 6. Electrical conductivity values in the incubation experiment.The left (a)–(c) show results for Kochi soil (K) and the right (d)–(f) do for Ushimado soil (U). The upper (a) and (d) show results for no compost-, the middle (b) and (e) for cattle manure compost (CC)-, and the lower (c) and (f) for mixed compost (MC)-amended soils. The numbers (160, 200, and 400) indicate NH4+-N application rates to soil (mg-N kg−1). Error bars indicate ± standard deviation (n=3).
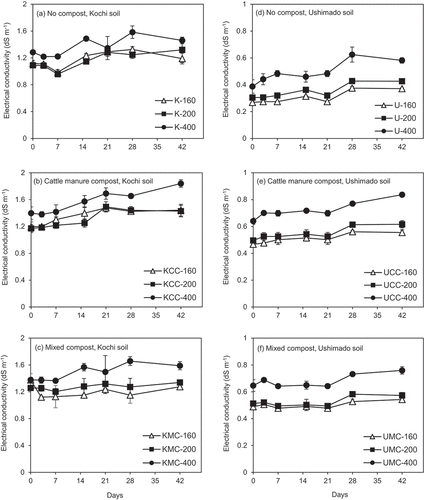
In Ushimado soil, no compost-amended samples showed the lowest EC values in range of 0.2–0.6 dS m−1 (), while both CC- and MC-amended soils showed the higher EC values (). Among all treatments, the lowest and highest EC values were at NH4+-N rates 160 and 400, respectively (). At NH4+-N rate 400 (U-400, UCC-400, and UMC-400 treatments), EC slightly increased over time.
3.5. Relative abundances of narG and nosZ genes in soil
In Kochi and Ushimado soil treatments, narG and nosZ gene copy numbers increased relative to the original soil (see the footnote in ) during the incubation. Both no compost- and MC-amended Ushimado soil () contained higher copy numbers of the narG gene than respective treatments of Kochi soil (). Even the original Ushimado soil contained more copy numbers of the narG gene than the original Kochi soil. In contrast, CC-amended Kochi soil () had higher copy numbers of the narG gene than respective samples of Ushimado soil (). Except KMC-160 and KMC-200 treatments, higher copy numbers of the nosZ gene were found in Kochi soil than respective treatments of Ushimado soil ().
Figure 7. Cumulative N2O emissions until day 7 or 21, narG, and nosZ gene copy numbers in the incubation experiment.The left (a)–(c) show results for Kochi soil (K) and the right (d)–(f) do for Ushimado soil (U). The upper (a) and (d) show results for no compost-, the middle (b) and (e) for cattle manure compost (CC)-, and the lower (c) and (f) for mixed compost (MC)-amended soils. The numbers (160, 200, and 400) indicate NH4+-N application rates to soil (mg-N kg−1). Error bars indicate ± standard deviation (n=3).
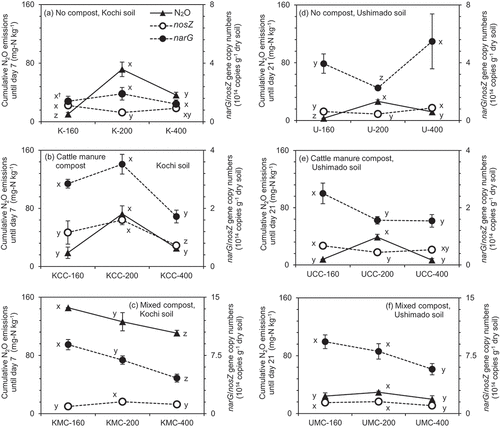
3.6 Relationships between relative abundances of functional genes, nosZ or narG, and cumulative N2Oemissions untilday 7 (Kochi soil) or 21 (Ushimado soil) at different NH4+-N rates
In no compost-amended Kochi soil, copy numbers of the narG gene were the same across NH4+-N rates (). Copy numbers of the nosZ gene significantly decreased from NH4+-N rate 160 (K-160) to 200 (K-200) (p <0.05) and those in the K-400 treatment were not different from both other treatments. Overall, cumulative N2O emissions until day 7 were negatively correlated with copy numbers of the nosZ gene across NH4+-N rates (R2 = 0.66, p <0.05), while they were not with those of the narG gene.
In CC-amended Kochi soil, copy numbers of the narG gene in the KCC-400 treatment were significantly lower than those in KCC-160 and KCC-200 treatments, while copy numbers of the nosZ gene significantly increased from NH4+-N rate 160 (KCC-160) to 200 (KCC-200) and decreased in the KCC-400 treatment (p <0.05, ). Cumulative N2O emissions until day 7 were positively correlated only with copy numbers of the narG gene at NH4+-N rates 200 and 400 (R2 = 0.91, p <0.05).
In MC-amended Kochi soil, copy numbers of the narG gene continuously and significantly decreased from NH4+-N rate 160 (KMC-160) to 400 (KMC-400), while copy numbers of the nosZ gene significantly increased from NH4+-N rate 160 (KMC-160) to 200 (KMC-200) and decreased from NH4+-N rate 200 (KMC-200) to 400 (KMC-400) (p <0.05, ). Cumulative N2O emissions until day 7 were positively correlated with copy numbers of the narG gene across NH4+-N rates (R2 = 0.67, p <0.05), while they were not with those of the nosZ gene.
In no compost-amended Ushimado soil, copy numbers of the narG gene significantly decreased from NH4+-N rate 160 (U-160) to 200 (U-200) and increased in the U-400 treatment, while copy numbers of the nosZ gene were significantly higher in the U-400 treatment than those in U-160 and U-200 treatments (p <0.05, ). Cumulative N2O emissions until day 21 were negatively correlated with copy numbers of the nosZ gene at NH4+-N rates 200 and 400 (R2 = 0.74, p <0.05), while they were not with those of the narG gene.
In CC-amended Ushimado soil, copy numbers of both narG and nosZ genes significantly decreased from NH4+-N rate 160 (UCC-160) to 200 (UCC-200) (p <0.05), while those were not different between UCC-200 and UCC-400 treatments (). Cumulative N2O emissions until day 21 and copy numbers of the nosZ gene at NH4+-N rates 160 and 200 were negatively correlated (R2 = 0.90, p <0.05).
In MC-amended Ushimado soil, copy numbers of both narG and nosZ genes at NH4+-N rate 160 (UMC-160) were not different from those at NH4+-N rate 200 (UMC-200), whereas a significant decrease in both genes was observed from NH4+-N rate 200 (UMC-200) to 400 (UMC-400) (p <0.05, ). In those samples, cumulative N2O emissions until day 21 were not significantly correlated (p >0.05) with gene copy numbers of either narG or nosZ regardless of NH4+-N rates.
When comparing two types of soil, although no compost- and MC-amended Ushimado soil contained higher copy numbers of the narG gene than respective samples of Kochi soil (), N2O emissions were considerably lower than those in Kochi soil (). In Kochi soil, N2O emissions were positively correlated with copy numbers of the narG gene (R2 = 0.78, p <0.05, ), whereas they were not in Ushimado soil (R2 = 0.39, p >0.05).
4. Discussion
4.1. N2O and CO2 emissions from manure compost-amended soil
In MC-amended treatments of both soils, higher cumulative N2O and CO2 emissions occurred than those in respective no compost- and CC-amended samples at each NH4+-N rate (). Relatively higher NH4+-N contents in MC than those in CC () might be a dominant contributing factor to the higher N2O emissions. A positive relationship between daily N2O emissions and soil NH4+-N contents was observed by Kim et al. (Citation2019), because NH4+-N is a primary substrate of N2O formation through nitrification (Hu et al. Citation2020). Furthermore, MC contained a higher NO3−-N content (), which would have promoted denitrification to produce N2O. High N2O emissions can be related to soil NO3−-N contents when soil WFPS is up to 70% because N2O was predominantly produced by denitrification (Zanatta et al. Citation2010). In addition, because MC was characterized by a low C/N ratio compared with that of CC (), MC would have easily decomposed, resulting in higher N2O and CO2 emissions. Organic amendment with a low C/N ratio resulted in release of mineral N via mineralization, whereas those with a high C/N ratio led to temporary N immobilization (Binh and Shima Citation2018). Toma and Hatano (Citation2007) observed high N2O emissions from soil amended with plant materials having a low C/N ratio due to faster N mineralization. Mohammed-Nour et al. (Citation2021) reported low cumulative CO2 emission from composted cattle manure with a high C/N ratio, which agreed with our results.
Of the two types of soil, all Kochi soil treatments showed higher cumulative N2O and CO2 emissions than respective treatments of Ushimado soil (). Ushimado soil contained lower contents of mineral N, total N, and C than those in Kochi soil. Similar to our results of Ushimado soil, Feng et al. (Citation2003) observed low N2O emissions in a soil with a low organic matter content and Mazzarino et al. (Citation1991) found that N mineralization in soil was limited with low total N contents in soil. Chiyoka et al. (Citation2011) observed that higher N2O emissions occurred in a soil with high clay and organic matter contents, which favored the formation of anaerobic micro-sites, promoting denitrification under aerobic conditions similar to the results of Kochi soil. The presence of readily metabolized organic matter is closely correlated to the rate of biological denitrification and hence the potential N2O emissions from soil (Wlodarczyk, Glinski, and Kotowska Citation2004). Generally, nitrification is optimum within the pH range of 4.9–7.2 (Stams, Flameling, and Marnette Citation1990). In Kochi soil, pH values of 5.5–6.6 were favorable for nitrifying activities than those in Ushimado soil, in which pH ranged between 6.6 and 8.4. Wang et al. (Citation2018) detected higher N2O emissions in a soil with pH 5 than in an alkaline soil with pH 8. In all Kochi soil treatments, peak N2O emissions appeared within the first week of incubation (). Velthof, Kuikman, and Oenema (Citation2003) documented that metabolization of volatile fatty acids in manure occurred within a few days after the incorporation into soil by denitrification. Jager et al. (Citation2011) reported increased N2O emissions during a shorter period after the application of organic fertilizer because easily available organic matter fractions promoted formation of anoxic soil micro sites, triggering N2O emissions by providing easily available substrates for nitrification and denitrification. Ushimado soil showed peak N2O emissions comparatively later than those of Kochi soil () probably because of the delayed growth of microorganisms in Ushimado soil due to low N and organic C availability. In addition, higher WFPS of Kochi soil (69–72%) compared with Ushimado soil (44–46%) at the same WHC has resulted in higher N2O emissions. Davidson et al. (Citation1991) suggested that a threshold between denitrification and nitrification existed in about 60% WFPS. These results confirmed that earlier peak and higher N2O emissions in Kochi soil might be due to nitrification, nitrifier-denitrification, and/or denitrification presumably promoted by high contents of mineral N, total N and C, and clay, favorable levels of soil pH, and possibly because of higher WFPS than those in Ushimado soil at the same WHC. Thus, the effects of compost type and soil type on N2O emissions were significant (p <0.001, ).
4.2. Effects of different NH4+-N rates on N2O emissions
In no compost- and CC-amended Kochi and Ushimado soils, the lowest and highest cumulative N2O emissions occurred at NH4+-N rates 160 (K-160, KCC-160, U-160, and UCC-160 treatments) and 200 (K-200, KCC-200, U-200, and UCC-200 treatments), respectively (). According to Wang et al. (Citation2021), NH4+-N content is a key factor affecting N2O emissions because it is directly subjected to nitrification. Well et al. (Citation2008) also found that N2O fluxes in an aerobic soil were significantly increased by NH4+-N additions from 0 to 40 mg-N kg−1 (p <0.001) because N2O emissions were governed by NH4+-N availability. In addition, higher nitrification rates lead to increased availability of NH2OH, NO, and NO3−, as well as promote O2 consumption, which can evoke denitrification and subsequent release of N2O (Signor and Cerri Citation2013; Lazcano, Zhu-Barker, and Decock Citation2021). Thus, increased NH4+-N contents induced nitrification directly and denitrification indirectly to emit N2O in the present study.
In all treatments except in MC-amended Ushimado soil, cumulative N2O emissions at NH4+-N rate 400 were significantly lower than those at NH4+-N rate 200 (p <0.05, ), which was probably due to the suppression of microbial activities by higher NH4+-N contents. The lower N2O peak heights at NH4+-N rate 400 than those at NH4+-N rate 200 also hinted the suppression of microbial activities at the highest NH4+-N content (). In Kochi and Ushimado soil treatments, EC at NH4+-N rate 400 (1.2–1.8 and 0.4–0.8 dS m−1, respectively) was higher than those at NH4+-N rate 200 (0.9–1.4 and 0.3–0.6 dS m−1, respectively, ). Smith and Doran (Citation1996) suggested that nitrification and denitrification activities were suppressed at EC >0.6 dS m−1 and the EC threshold imposing microbial stress can vary due to the addition of decomposable organic amendments. Furthermore, Adviento-Borbe et al. (Citation2006) observed decreased nitrification within the EC range of 0.5–2.0 dS m−1. Meng et al. (Citation2020) detected negative impacts of EC of 1.24 to 12.96 dS m−1 on denitrification. Based on these findings, the EC range in NH4+-N rate 400 treatments seemed to enforce more salt stress on microorganisms than that in NH4+-N rate 200 treatments, resulting in low N2O emissions in our study. Deppe et al. (Citation2017) observed inhibition of net and gross nitrification at NH4+-N contents higher than 450 mg-N kg−1 with concurrent inhibition of N2O production. According to their experiment, nitrification was negligible at 5000 mg-N kg−1 NH4+-N content, probably due to osmotic pressure. They also suggested an inhibition effect of high NH4+-N contents due to pH changes in soil. However, in our experiment, considerable differences in pH values across NH4+-N rates were not observed (). Muller et al. (Citation2006) also found that bacterial growth was impaired by the addition of (NH4)2SO4 due to an enhanced osmolarity of the medium. Tong and Xu (Citation2012) reported that nitrification was inhibited due to the inhibition of ammonia oxidizing bacteria populations, while maintaining a constant soil pH by the addition of 300 and 400 mg-N kg−1 as (NH4)2SO4. Wang et al. (Citation2020) found that (NH4)2SO4 did not affect soil pH but reduced net nitrification rates mainly due to the acidic nature of the fertilizer at the maximum application rate of 200 mg-N kg−1 soil. Hoang and Maeda (Citation2018) observed increased cumulative N2O emissions with increasing NH4+-N application rates from 0 to 800 mg-N kg−1 and then declined at 1200 mg-N kg−1 and concluded that N2O emissions were not exponentially correlated with NH4+-N application rates. All these studies reported the inhibitory effect of NH4+-N on N2O emissions from mineral N fertilizer added soils and the inhibitory NH4+-N contents were either higher or lower than those of our study. Menyailo, Stepanov, and Umarov (Citation1997) observed a dominance of N2O emissions in a saline soil, possibly due to the inhibition of N2O reductase by salts, which was different from our study, in which N2O emissions became lower at NH4+-N rate 400 than those at NH4+-N rate 200.
Kim, Lee, and Keller (Citation2006) suggested that nitrification was inhibited by free ammonia (NH3). At high pH, some NH4+-N can be converted to NH3 (Liu et al. Citation2019; Mohammed-Nour, Al-Sewailem, and El-Naggar Citation2019). Hence, the presence of free NH3 probably reduced N2O emissions in Ushimado soil at NH4+-N 400 (U-400 and UCC-400 treatments) under high pH of 7–8.2 and high NH4+-N conditions. In addition to the effects of compost and soil types, NH4+-N rates had significant effects on N2O emissions (p <0.05, ). Our study reports the suppression of N2O emissions at NH4+-N rate 400 in different soil types amended with compost for the first time to the best of our knowledge, which is a novel finding.
4.3. Effects of different NH4+-N rates on CO2 emissions
Due to the suppression of cumulative CO2 emissions by increasing NH4+-N contents from NH4+-N rate 200 to 400 in manure compost-amended Kochi and Ushimado soils (p <0.05, ) except in CC-amended Ushimado soil, the effects of NH4+-N rates were significant (p <0.001, ). In all treatments, CO2 emissions started decreasing after peak emissions (). The low availability of easily decomposable C is a reason for the decrease of CO2 emissions with time. The soil C content is a crucial factor to increase CO2 emissions from soil microbial respiration (Ray et al. Citation2020). Pelster et al. (Citation2012) observed high CO2 emissions from poultry manure-amended soil due to a higher available C content. In Kochi soil, cumulative CO2 emissions at NH4+-N rate 160 were the same level as those at NH4+-N rate 200 and cumulative CO2 emissions at NH4+-N rate 400 were significantly lower than those at NH4+-N rate 200 (p <0.05, ). Treseder (Citation2008) documented declining CO2 emissions in concert with declines in microbial populations under N fertilization by induced osmotic potentials, which became toxic, owing to the additional ions from N fertilizer. In addition, nitrogenous compounds from fertilizer can condense with carbohydrates producing melanoidins, and they can increase the polymerization of polyphenols to ‘brown compounds.’ Both melanoidins and brown compounds are recalcitrant to decomposition, limiting C availability for microbial respiration (Cao et al. Citation2021). Hence, the lowest cumulative CO2 emissions at NH4+-N rate 400 of Kochi soil in our experiment were probably due to osmotic stress and low C availability for microorganisms.
In Ushimado soil, significant differences in cumulative CO2 emissions across NH4+-N rates were observed only when amended with MC. The cumulative CO2 emissions in the UMC-160 treatment were higher than those in UMC-200 and UMC-400 treatments (p <0.05, ). In CC-amended treatments, less total C content in Ushimado soil and mineralization-resistant CC might have limited C available for microorganisms regardless of NH4+-N rates. In easily decomposable MC-amended Ushimado soil, cumulative CO2 emissions were much lower at NH4+-N rate 400 than those at the other NH4+-N rates, possibly due to osmotic stress.
4.4. Relation of temporal variation of NH4+-N and NO3−-N contents to N2O emissions
In Kochi soil, NH4+-N contents started decreasing from day 3, except in K-200 and KMC-160 treatments, in which the reduction started on day 7 (). The reduction of NH4+-N contents indicated the occurrence of nitrification. The beginning date of nitrification was similar to the day of peak N2O emissions in Kochi soil treatments (). All Kochi soil treatments those received (NH4)2SO4 showed earlier nitrification than the KMC-160 treatment that contained only the original NH4+-N contents. Nitrification rates probably became faster with the addition of (NH4)2SO4 because extra NH4+-N is a substrate for ammonia oxidation (Jones et al. Citation2007; Yin et al. Citation2019). In our study, the KMC-160 treatment showed a delayed start of nitrification, in which N mineralization was larger than in the other treatments because of no stress on microorganisms by the addition of NH4+-N.
Ushimado soil treatments also showed prominent nitrification within the first 3 weeks of incubation (), although the nitrification rate was slower than that in Kochi soil. Unlike the others, in U-400, UCC-400, and UMC-400 treatments, NH4+-N contents continuously decreased because of more NH4+-N, which remained between 100 and 200 mg-N kg−1 even at the end of the experiment. This indicated that NH4+-N in those treatments was not totally nitrified within 42 days of incubation. This was probably due to low C availability in Ushimado soil. Silva et al. (Citation2019) and Zhang, Müller, and Cai (Citation2015) reported the existence of heterotrophic nitrifying microorganisms. Organic C serves as energy and electron sources for heterotrophic nitrifiers (Yang et al. Citation2016). Hence, in Ushimado soil at NH4+-N rate 400, the activities of heterotrophic nitrifiers could be restricted by low C contents, resulting in reduced nitrification.
In Kochi soil, initial NO3−-N contents decreased until day 3 or 7, on which peak N2O emissions occurred, and then started increasing with time (), concurrently with the reduction of NH4+-N contents. The initial reduction of NO3−-N suggested the occurrence of denitrification. Congreves, Phan, and Farrell (Citation2019) suggested that at 53% to 78% WFPS, N2O emission from soil was attributable to denitrification. Zhu et al. (Citation2013) reported that denitrification can occur following the addition of urea or (NH4)2SO4 to loam, clay loam, and sandy loam soils, resulting in N2O emissions. Therefore, N2O emissions in the first week in Kochi soil treatments (69–72% WFPS) were due to both nitrification and denitrification. The KMC-160 treatment showed the lowest NO3−-N content among all MC-amended Kochi soil treatments (), revealing that at NH4+-N rate 160, more NO3−-N contents were subjected to denitrification. This can be a reason for the highest cumulative N2O emissions in the KMC-160 treatment than those in KMC-200 and KMC-400 treatments (). With increasing NH4+-N rate from 200 to 400, denitrification decreased remaining high NO3−-N contents throughout the incubation period in all Kochi soil treatments (). Similarly, Deppe et al. (Citation2017) suggested that N2O emissions during denitrification can be inhibited at high NH4+-N contents.
In Ushimado soil, NO3−-N contents were lower than those in Kochi soil, and large differences among treatments were not observed (). Denitrification potential of Ushimado soil was lower than that of Kochi soil possibly due to low C and N availability in Ushimado soil. In addition, lower WFPS of Ushimado soil may not create favorable anaerobic conditions for high denitrifying activities than that of Kochi soil.
4.5. Relation between relative abundances of functional genes, nosZ or narG, and cumulative N2O emissions
Both no compost- and MC-amended treatments of Ushimado soil at each NH4+-N rate contained higher copy numbers of the narG gene than respective treatments of Kochi soil (). In contrast, N2O emissions in Ushimado soil were considerably lower than those in Kochi soil (). This may be due to less C and N contents in Ushimado soil (), because the activities of denitrifiers are highly affected by the supply of NO3−-N and labile C (Lazcano, Zhu-Barker, and Decock Citation2021). Additionally, lower WFPS of Ushimado soil was not favorable for high denitrifying microbial activities than those of Kochi soil. This reveals that even if denitrifying genes are abundant in soil, N2O emissions are determined by their activities, which are affected by the availability of C and N substrates. The ability of denitrifying bacteria to produce N2O depends on abiotic conditions such as O2 level, pH, temperature, moisture, and the availability of C and N (Yang, Zhang, and Ju Citation2017; Usyskin-Tonne, Hadar, and Minz Citation2019). Röling (Citation2007) suggested that gene pools may not always reflect rates of N2O emissions due to subsequent controls over gene transcription and enzyme activities. Accordingly, gene abundances may reflect only genetic potential within the cropping systems for N2O emissions (Duan et al. Citation2018).
The high activities of narG genes in Kochi soil were confirmed by higher NO3−-N contents available in the treatments with a substrate for bacteria with narG genes than those in Ushimado soil during the incubation (). Therefore, a stronger positive linear relationship (R2 = 0.78, ) between cumulative N2O emissions and copy numbers of the narG gene was observed in Kochi soil than that in Ushimado soil (R2 = 0.39). Yang, Zhang, and Ju (Citation2017) observed a significant correlation between N2O emissions and copy numbers of the narG gene in urea-based fertilizer and/or cattle manure-amended soil. High NH4+-N from urea-based fertilizers stimulated the growth of ammonia oxidizers, leading to microoxic or anoxic conditions, which in turn induced denitrification by heterotrophic denitrifiers, leading to high N2O emissions. We observed a similar relationship between copy numbers of the narG gene and cumulative N2O emissions in Kochi soil with high C and N contents amended with manure compost and supplemented with extra NH4+-N at higher WFPS than those in Ushimado soil at the same WHC.
According to the results in a clear inhibitory effect of high NH4+-N contents on both narG and nosZ genes in compost-amended Kochi and MC-amended Ushimado soils was observed due to significantly lower gene copy numbers at NH4+-N rate 400 than those at NH4+-N rate 200 (p <0.05). Dincer and Kargi (Citation1999) reported that NaCl concentrations above 20 g L−1 resulted in significant reductions in performances of both nitrification and denitrification, while denitrification was more sensitive to high salt contents. Hence, the salt stress at NH4+-N rate 400 was a possible reason for the reduced narG and nosZ gene copy numbers.
5. Conclusions
We aimed at determining the effects of different application rates of NH4+-N on N2O and CO2 emissions from two contrasting types of manure compost-amended Kochi and Ushimado soils under aerobic conditions. Further, we analyzed the relationships between relative abundances of narG or nosZ genes and N2O emissions from compost-amended soil. Results showed that N2O and CO2 emissions in this experiment were higher in both soil types when MC with a higher mineral N content and a lower C/N ratio was amended than those when CC was amended. Emissions of N2O and CO2 were higher in MC- or CC-amended Kochi soil because of higher mineral N, total N and C, and clay contents, favorable levels of soil pH, and greater WFPS than those of Ushimado soil at the same WHC. Additionally, in no compost- and CC-amended Kochi and Ushimado soils, raising NH4+-N rate from 160 to 200 increased cumulative N2O emissions due to the stimulation of nitrification directly by the supply of NH4+-N. A decrease in N2O emissions at NH4+-N rate 400 was observed comparing with those at NH4+-N rate 200 due to the suppression of microorganisms by the osmotic stress in all Kochi soil and no compost- and CC-amended Ushimado soil. A significant decrease in CO2 emissions at NH4+-N rate 400 was observed in all Kochi soil and MC-amended Ushimado soil treatments comparing with those at NH4+-N rate 200 possibly due to osmotic stress and C limitation. Increasing copy numbers of the narG gene increased N2O emissions (R2 = 0.78) in Kochi soil with higher available N and C contents, and greater WFPS than those in Ushimado soil at the same WHC. Our study revealed that NH4+-N rate 400 can suppress N2O and CO2 emissions from compost-amended soil under aerobic conditions, suggesting that soil NH4+-N contents must be considered for estimation of N2O and CO2 emissions from manure compost-amended soil.
Type of contribution
Full-length paper
Division of the manuscript
Environment
Acknowledgments
We acknowledge Masaya Ooya (Okayama Prefectural Technology Center for Agriculture, Forestry and Fisheries) for the supply of compost and related information for the study. We would like to thank the Ministry of Education, Culture, Sports, Science, and Technology, Japan (MEXT) for offering a scholarship for a Doctoral course.
Disclosure statement
No potential conflict of interest was reported by the author(s).
Additional information
Funding
References
- Adviento-Borbe, M. A. A., J. W. Doran, R. A. Drijber, and A. Dobermann. 2006. “Soil Electrical Conductivity and Water Content Affect Nitrous Oxide and Carbon Dioxide Emissions in Intensively Managed Soils.” Journal of Environmental Quality 35 (6): 1999–2010. doi:10.2134/jeq2006.0109.
- Bateman, E. J., and E. M. Baggs. 2005. “Contributions of Nitrification and Denitrification to N2O Emissions from Soils at Different water-filled Pore Space.” Biology and Fertility of Soils 41 (6): 379–388. doi:10.1007/s00374-005-0858-3.
- Binh, N. T., and K. Shima. 2018. “Nitrogen Mineralization in Soil Amended with Compost and Urea as Affected by Plant Residues Supplements with Controlled C/N Ratios.” Journal of Advanced Agricultural Technologies 5 (1): 8–13. doi:10.18178/joaat.5.1.8-13.
- Boeckx, P., D. Beheydt, and O. V. Cleemput. 2005. “Nitrous Oxide Emissions from Soils: From the Bottle to the Model.” Environmental Sciences 2 (2–3): 109–115. doi:10.1080/15693430500402259.
- Cai, Y. J., W. X. Ding, and J. F. Luo. 2013. “Nitrous Oxide Emissions from Chinese maize-wheat Rotation Systems: A 3-year Field Measurement.” Atmospheric Environment 65: 112–122. doi:10.1016/j.atmosenv.2012.10.038.
- Cao, R., L. Chen, X. Hou, X. Lu, and H. Li. 2021. “Nitrogen Addition Reduced Carbon Mineralization of Aggregates in Forest Soils but Enhanced in Paddy Soils in South China.” Ecological Processes 10 (45). doi:10.1186/s13717-021-00319-z.
- Chiyoka, W. L., X. Hao, F. Zvomuya, and X. Li. 2011. “Nitrous Oxide Emissions from Chernozemic Soils Amended with Anaerobically Digested Beef Cattle Feedlot Manure: A Laboratory Study.” Animal Feed Science and Technology 166-167: 492–502. doi:10.1016/j.anifeedsci.2011.04.035.
- Congreves, K. A., T. Phan, and R. E. Farrell. 2019. “A New Look at an Old Concept: Using N2O Isotopomers to Understand the Relationship between Soil Moisture and N2O Production Pathways.” Soil 5 (2): 265–274. doi:10.5194/soil-5-265-2019.
- Cultivated soil classification committee. 1995. “Classification of Cultivated Soils in Japan (3 Approximation).” Miscellaneous Publication of the National Institute of Agro-environmental Sciences 17: 1–79.
- Darrah, P. R., R. E. White, and H. Nye. 1986. “Simultaneous Nitrification and Diffusion in Soil. II. The Effects at Levels of Ammonium Chloride Which Inhibit Nitrification.” Journal of Soil Science 37 (1): 41–52. doi:10.1111/j.1365-2389.1986.tb00005.x.
- Davidson, E. A., S. C. Hart, C. A. Shanks, and M. K. Firestone. 1991. “Measuring Gross Nitrogen Mineralization, and Nitrification by N Isotopic Pool Dilution in Intact Soil Cores.” European Journal of Soil Science 42 (3): 335–349. doi:10.1111/j.1365-2389.1991.tb00413.x.
- Deng, Q., D. Hui, J. Wang, C. L. Yu, C. Li, K. C. Reddy, and S. Dennis. 2016. “Assessing the Impacts of Tillage and Fertilization Management on Nitrous Oxide Emissions in a Cornfield Using the DNDC Model.” Journal of Geophysical Research: Biogeosciences 121 (2): 337–349. doi:10.1002/2015JG003239.
- Deppe, M., R. Well, A. Giesemann, O. Spott, and H. Flessa. 2017. “Soil N2O Fluxes and Related Processes in Laboratory Incubations Simulating Ammonium Fertilizer Depots.” Soil Biology & Biochemistry 104: 68–80. doi:10.1016/j.soilbio.2016.10.005.
- Dincer, A. R., and F. Kargi. 1999. “Salt Inhibition of Nitrification and Denitrification in Saline Wastewater.” Environmental Technology 20 (11): 1147–1153. doi:10.1080/09593332008616912.
- Ding, W., Y. Cai, Z. Cai, K. Yagi, and X. Zheng. 2007. “Soil Respiration under Maize Crops: Effects of Water, Temperature, and Nitrogen Fertilization.” Soil Science Society of America Journal 71: 944–951. doi:https://doi.org/10.2136/sssaj2006.0160.
- Dobbie, K. E., and K. A. Smith. 2003. “Nitrous Oxide Emission Factors for Agricultural Soils in Great Britain: The Impact of Soil Water filled-pore Space and Other Controlling Variables.” Global Change Biology 9 (2): 204–218. doi:10.1046/j.1365-2486.2003.00563.x.
- Duan, Y., S. Hallin, C. M. Jones, A. Prieme, R. Labouriau, and S. O. Petersen. 2018. “Catch Crop Residues Stimulate N2O Emissions during Spring, without Affecting the Genetic Potential for Nitrite and N2O Reduction.” Frontiers in Microbiology 9 (2629). doi:10.3389/fmicb.2018.02629.
- Eaton, A. D., L. S. Clesceri, E. W. Rice, and Greenberg A.E. 2005. “Flow Injection Analysis.” In Standard Methods for the Examination of water and wastewater, 21 ed. Washington, DC: American Public Health Association 116–129 .
- Feng, K., F. Yan, B. N. Hutsch, and S. Schubert. 2003. “Nitrous Oxide Emission as Affected by Liming an Acidic Mineral Soil Used for Arable Agriculture.” Nutrient Cycling in Agroecosystems 67 (3): 283–292. doi:10.1023/B:FRES.0000003664.51048.0e.
- Gagnon, B., N. Ziadi, P. Rochette, M. H. Chantigny, D. A. Angers, N. Bertrand, and W. N. Smith. 2016. “Soil-surface Carbon Dioxide Emission following Nitrogen Fertilization in Corn.” Canadian Journal of Soil Science 96 (2): 219–232. doi:10.1139/cjss-2015-0053.
- Grave, R. A., R. S. Nicoloso, P. C. Cassol, C. Aita, J. C. Correa, M. D. Costa, and D. D. Fritz. 2015. “Short-term Carbon Dioxide Emission under Contrasting Soil Disturbance Levels and Organic Amendments.” Soil and Tillage Research 146: 184–192. doi:10.1016/j.still.2014.10.010.
- Henry, S., D. Bru, B. Stres, S. Hallet, and L. Philippot. 2006. “Quantitative Detection of the nosZ Gene, Encoding Nitrous Oxide Reductase, and Comparison of the Abundances of 16s rRNA, narG, nirK, and nosZ Genes in Soils.” Applied and Environmental Microbiology 72 (8): 5181–5189. doi:10.1128/AEM.00231-06.
- Hoang, V. N. T., and M. Maeda. 2018. “Interactive Effects of Ammonium Application Rates and Temperature on Nitrous Oxide Emission from Tropical Agricultural Soil.” Soil Science and Plant Nutrition 64 (6): 767–773. doi:10.1080/00380768.2018.1517280.
- Hu, Y., M. P. Gabner, A. Weber, M. Schraml, and U. Schmidhalter. 2020. “Direct and Indirect Effects of Urease and Nitrification Inhibitors on N2O-N Losses from Urea Fertilization to Winter Wheat in Southern Germany.” Atmosphere 11 (782). doi:10.3390/atmos11080782.
- Jager, N., C. F. Stange, B. Ludwig, and H. Flessa. 2011. “Emission Rates of N2O and CO2 from Soil with Different Organic Matter Content from Three long-term Fertilization experiments-a Laboratory Study.” Biology and Fertility of Soils 47 (5): 483–494. doi:10.1007/s00374-011-0553-5.
- Jin, T., M. Shimizu, S. Marutani, A. R. Desyatkin, N. Iizuka, H. Hata, and R. Hatano. 2010. “Effect of Chemical Fertilizer and Manure Application on N 2 O Emission from Reed Canary Grassland in Hokkaido, Japan.” Soil Science and Plant Nutrition 56 (1): 53–65. doi:10.1111/j.1747-0765.2010.00447.x.
- Jones, S. K., R. M. Rees, U. M. Skiba, and B. C. Ball. 2007. “Influence of Organic and Mineral N Fertiliser on N2O Fluxes from a Temperate Grassland.” Agriculture, Ecosystems & Environment 121 (1–2): 74–83. doi:10.1016/j.agee.2006.12.006.
- Katoh, M., Y. Hayashi, and H. Morikuni. 2008. “Effect of Combined Applications of N-labeled Ammonium Sulfate and Cattle Manure Composts on Nitrogen Supply Potential of Soil to Spinach.” Japanese Journal of Soil Science and Plant Nutrition 79 (3): 283–289. doi:https://doi.org/10.20710/dojo.79.3_283.
- Kettler, T. A., J. W. Doran, and T. L. Gilbert. 2001. “Simplified Method for Soil particle-size Determination to Accompany soil-quality Analyses.” Soil Science Society of America Journal 65 (3): 849–852. doi:10.2136/sssaj2001.653849x.
- Kim, D., D. Lee, and J. Keller. 2006. “Effect of Temperature and Free Ammonia on Nitrification and Nitrite Accumulation in Landfill Leachate and Analysis of Its Nitrifying Bacterial Community by FISH.” Bioresource Technology 97 (3): 459–468. doi:10.1016/j.biortech.2005.03.032.
- Kim, S. U., C. Ruangcharus, S. Kumar, H. H. Lee, H. J. Park, E. S. Jung, and C. O. Hong. 2019. “Nitrous Oxide Emission from Upland Soil Amended with Different Animal Manures.” Applied Biological Chemistry 62 (1). doi:10.1186/s13765-019-0409-5.
- Kurganova, I. N., and V. O. L. de Gerenyu. 2010. “Effect of the Temperature and Moisture on the N2O Emission from Some Arable Soils.” Eurasian Soil Science 43 (8): 919–928. doi:10.1134/S1064229310080090.
- Lazcano, C., X. Zhu-Barker, and C. Decock. 2021. “Effects of Organic Fertilizers on the Soil Microorganisms Responsible for N2O Emissions: A Review.” Microorganisms 9 (983). doi:10.33990/microorganisms9050983.
- Li, P., M. Lang, C. Li, and X. Hao. 2017. “Nitrous Oxide and Carbon Dioxide Emissions from Soils Amended with Compost and Manure from Cattle Fed Diets Containing Wheat Dried Distillers’ Grains with Solubles.” Canadian Journal of Soil Science 97: 522–531. doi:10.1139/CJSS-2016-0068.
- Lin, S., S. Zhang, G. Shen, M. Shaaban, W. Ju, Y. Cui, C. Duan, and L. Fang. 2021. “Effects of Inorganic and Organic Fertilizers on CO2 and CH4 Fluxes from Tea Plantation Soil.” Elementa: Science of the Anthropocene 9 (1). doi:10.1525/elementa.2021.090.
- Liu, Y., H. H. Ngo, W. Guo, L. Peng, D. Wang, and B. Ni. 2019. “The Roles of Free Ammonia (FA) in Biological Wastewater Treatment Processes: A Review.” Environment International 123: 10–19. doi:10.1016/j.envint.2018.11.039.
- Liu, D., H. Sun, X. Liao, J. Luo, S. Lindsey, J. Yuan, T. He, M. Zaman, and W. Ding. 2020. “N2O and NO Emissions as Affected by the Continuous Combined Application of Organic and Mineral N Fertilizer to a Soil on the North China Plain.” Agronomy 10 (12): 1965. doi 10.3390/agronomy10121965.
- Low, A. P., J. M. Stark, and M. Lynn. 1997. “Effects of Soil Osmotic Potential on Nitrification, Ammonification, N-assimilation, and Nitrous Oxide Production.” Soil Science 162 (1): 16–27. doi:10.1097/00010694-199701000-00004.
- Luo, G. J., R. Kiese, B. Wolf, and K. Butterbach-Bahl. 2013. “Effects of Soil Temperature and Moisture on Methane Uptake and Nitrous Oxide Emissions across Three Different Ecosystem Types.” Biogeosciences 10 (5): 3205–3219. doi:10.5194/bg-10-3205-2013.
- Mazzarino, M. J., L. Oliva, A. Abril, and M. Acosta. 1991. “Factors Affecting Nitrogen Dynamics in a Semiarid Woodland (Dry Chaco, Argentina).” Plant and Soil 138 (1): 85–98. doi:10.1007/BF00011811.
- Meng, Y., Z. He, B. Liu, L. Chen, P. Lin, and W. Luo. 2020. “Soil Salinity and Moisture Control the Processes of Soil Nitrification and Denitrification in a Riparian Wetland in an Extremely Arid Regions in Northwestern China.” Water 12 (2815). doi:10.3390/w12102815.
- Menyailo, O. V., A. L. Stepanov, and M. Umarov. 1997. “The Transformation of Nitrous Oxide by Denitrifying Bacteria in Solonchaks.” Eurasian Soil Science 30 (2): 178–180.
- Mohammed-Nour, A., M. Al-Sewailem, and A. H. El-Naggar. 2019. “The Influence of Alkalization and Temperature on Ammonia Recovery from Cow Manure and the Chemical Properties of the Effluents.” Sustainability 11 (8): 2441. doi:10.3390/su11082441.
- Mohammed-Nour, A., M. Al-Sewailem, A. H. El-Naggar, M. H. El-Saeid, A. A. Aly, and J. Elfaki. 2021. “Carbon and Nitrogen Dynamics, and CO2 Efflux in the Calcareous Sandy Loam Soil Treated with Chemically Modified Organic Amendments.” Molecules 26 (16): 4707. doi:10.3390/molecules26164707.
- Mosier, A. R. 1994. “Nitrous Oxide Emissions from Agricultural Soils.” Fertilizer Research 37 (3): 191–200. doi:10.1007/BF00748937.
- Mosier, A., C. Kroeze, C. Nevison, O. Oenema, S. Seitzingers, and O. V. Cleemput. 1998. “Closing the Global N2O Budget: Nitrous Oxide Emissions through the Agricultural Nitrogen Cycle.” Nutrient Cycling in Agroecosystems 52 (2/3): 225–248. doi:10.1023/A:1009740530221.
- Muller, T., B. Walter, A. Wirtz, and A. Burkovski. 2006. “Ammonium Toxicity in Bacteria.” Current Microbiology 52 (5): 400–406. doi:10.1007/s00284-005-0370-x.
- Nie, Y., L. Li, R. Isoda, M. Wang, R. Hatano, and Y. Hashidoko. 2016. “Physiological and Genotypic Characteristics of Nitrous Oxide (N2O)-emitting Pseudomonas Species Isolated from Dent Corn Andisol Farmland in Hokkaido, Japan.” Microbes and Environments 31 (2): 93–103. doi:10.1264/jsme2.ME15155.
- Nishio, M. 2001. “Analysis of the Actual State of Nitrogen Application in Japan as Seen in the Agricultural Production Environment Survey.” Japanese Society of Soil Science and Plant Nutrition 72 (4): 513–521.
- Otte, J. M., N. Blackwell, R. Ruser, A. Kappler, S. Kleindienst, and C. Schmidt. 2019. “N2O Formation by nitrite-induced (Chemo)denitrification in Coastal Marine Sediment.” Scientific Reports 9 (10691). doi:10.1038/s41598-019-47172-x.
- Parton, W. J., E. A. Holland, S. J. Del Grosso, M. D. Hartman, R. E. Martin, A. R. Mosier, D. S. Ojima, and D. Schimel. 2001. “Generalized Model for NOx and N2O Emissions from Soils.” Journal of Geophysical Research 106 (D15): 403–419. doi:10.1029/2001JD900101.
- Pelster, D. E., M. H. Chantigny, P. Rochette, D. A. Angers, C. Rieux, and A. Vanasse. 2012. “Nitrous Oxide Emissions Respond Differently to Mineral and Organic Nitrogen Sources in Contrasting Soil Types.” Journal of Environmental Quality 41 (2): 427–435. doi:10.2134/jeq2011.0261.
- Qiao, Y. F., S. J. Miao, X. Z. Han, M. Y. You, X. Zhu, and A. R. Horwath. 2014. “The Effect of Fertilizer Practices on N Balance and Global Warming Potential of maize-soybean-wheat Rotations in Northeastern China.” Field Crops Research 161: 98–106. doi:10.1016/j.fcr.2014.03.005.
- R Core Team. 2019. R: A Language and Environment for Statistical Computing. Vienna, Austria: R foundation for statistical computing. https://www.R-project.org/.
- Ray, R. L., R. W. Griffin, A. Fares, A. Elhassan, R. Awal, S. Woldesenbet, and E. Risch. 2020. “Soil CO2 Emission in Response to Organic Amendments, Temperature, and Rainfall.” Scientific Reports 10 (5849). doi:10.1038/s41598-020-62267-6.
- Röling, W. F. 2007. “Do Microbial Numbers Count? Quantifying the Regulation of Biogeochemical Fluxes by Population Size and Cellular Activity.” FEMS Microbiology Ecology 62 (2): 202–210. doi:10.1111/j.1574-6941.2007.00350.x.
- Sainju, U. M., W. B. Stevens, T. Caesar-Tonthat, and M. A. Liebig. 2012. “Soil Greenhouse Gas Emissions Affected by Irrigation, Tillage, Crop Rotation, and Nitrogen Fertilization.” Journal of Environmental Quality 41 (6): 1774–1786. doi:10.2134/jeq2012.0176.
- Sanchez-Garcia, M., A. Roig, M. A. Sanchez-Monedero, and M. L. Cayuela. 2014. “Biochar Increases Soil N2O Emissions Produced by nitrification-mediated Pathways.” Frontiers in Environmental Science 2 (25). doi:10.3389/fenvs.2014.00025.
- Signor, D., and C. E. P. Cerri. 2013. “Nitrous Oxide Emissions in Agricultural Soils: A Review.” Pesquisa Agropecuaria Tropical 43 (3): 322–338. doi:10.1590/S1983-40632013000300014.
- Silva, L. C. F., H. S. Lima, T. A. O. Mendes, A. Sartoratto, M. P. Sousa, R. S. Souza, S. O. Paula, et al. 2019. “Heterotrophic nitrifying/aerobic Denitrifying Bacteria: Ammonium Removal under Different physical-chemical Conditions and Molecular Characterization.” Journal of Environmental Management 248 (109294). doi:10.1016/j.jenvman.2019.109294.
- Smith, J. L., and J. W. Doran. 1996. “Measurement and Use of pH and Electrical Conductivity.” pp. 169–185. In Methods for Assessing Soil Quality. 49 (Soil Science Society of America Inc), edited by J. W. Doran and A. J. Jones, Madison, .
- Sosulski, T., W. Stepien, A. Was, and M. Szymanska. 2020. “N2O and CO2 Emissions from Bare Soil: Effect of Fertilizer Management.” Agriculture 10 (12): 602. doi:10.3390/agriculture10120602.
- Sparks, D. L., A. L. Page, R. A. Helmke, R. H. Loeppert, P. N. Soltanpour, M. A. Tabatabai, C. T. Johnston, and M. E. Summer. 1996. Methods of Soil Analysis: Chemical Methods. Madison, Wisconsin, USA: Soil Science Society of America Inc.
- Stams, A. J. M., E. M. Flameling, and E. C. L. Marnette. 1990. “The Importance of Autotrophic versus Heterotrophic Oxidation of Atmospheric Ammonium in Forest Ecosystems with Acid Soil.” FEMS Microbiology Ecology 74 (4): 337–344. doi:10.1111/j.1574-6968.1990.tb04080.x.
- Sun, J., B. Peng, L. Wei, Q. Guifang, D. Weiwei, G. Dai, J. Ping, S. Han, and E. Bai. 2016. “Effects of Nitrogen Addition on Potential Soil nitrogen-cycling Processes in a Temperate Forest Ecosystem.” Soil Science 181 (1): 29–38. doi:10.1097/SS.0000000000000134.
- Termorshuizen, A. J., S. W. Moolenaar, A. H. M. Veeken, and W. J. Blok. 2004. “The Value of Compost.” Reviews in Environmental Science and Bio-technology 3 (4): 343–347. doi:10.1007/s11157-004-2333-2.
- Tian, H., R. Xu, J. G. Canadell, R. L. Thompson, W. Winiwarter, P. Suntharalingam, E. A. Davidson, et al. 2020. “A Comprehensive Quantification of Global Nitrous Oxide Sources and Sinks.” Nature 586 (7828): 248–256. doi:10.1038/s41586-020-2780-0.
- Toma, Y., and R. Hatano. 2007. “Effect of Crop Residue C:N Ratio on N 2 O Emissions from Gray Lowland Soil in Mikasa, Hokkaido, Japan.” Soil Science and Plant Nutrition 53 (2): 198–205. doi:10.1111/j.1747-0765.2007.00125.x.
- Tong, D., and R. Xu. 2012. “Effects of Urea and (NH4)2SO4 on Nitrification and Acidification of Ultisols from Southern China.” Journal of Environmental Sciences 24 (4): 682–689. doi:10.1016/S1001-0742(11)60832-2.
- Treseder, K. K. 2008. “Nitrogen Additions and Microbial Biomass: A meta-analysis of Ecosystem Studies.” Ecology Letters 11 (10): 1111–1120. doi:10.1111/j.1461-0248.2008.01230.x.
- Usyskin-Tonne, A., Y. Hadar, and D. Minz. 2019. “Altering N2O Emissions by Manipulating Wheat Root Bacterial Community.” Scientific Reports 9 (7613). doi:10.1038/s41598-019-44124-3.
- Velthof, G. L., P. J. Kuikman, and O. Oenema. 2003. “Nitrous Oxide Emission from Animal Manures Applied to Soil under Controlled Conditions.” Biology and Fertility of Soils 37 (4): 221–230. doi:10.1007/s00374-003-0589-2.
- Wang, Y., J. Guo, R. D. Vogt, J. Mulder, J. Wang, and X. Zhang. 2018. “Soil pH as the Chief Modifier for Regional Nitrous Oxide Emissions: New Evidence and Implications for Global Estimates and Mitigation.” Global Change Biology 24 (2): 617–626. doi:10.1111/gcb.13966.
- Wang, J., X. Tu, H. Zhang, J. Cui, K. Ni, J. Chen, Y. Chen, J. Zhang, and S. X. Chang. 2020. “Effects of ammonium-based Nitrogen Addition on Soil Nitrification and Nitrogen Gas Emissions Depend on fertilizer-induced Changes in pH in a Tea Plantation Soil.” The Science of the Total Environment 747 (141340). doi:10.1016/j.scitotenv.2020.141340.
- Wang, C., B. Amon, K. Schulz, and B. Mehdi. 2021. “Factors that Influence Nitrous Oxide Emissions from Agricultural Soils as Well as Their Representation in Simulation Models: A Review.” Agronomy 11 (4) 770. doi:https://doi.org/10.3390/agronomy11040770. .
- Well, R., H. Flessa, L. Xing, J. Xiaotang, and V. Romheld. 2008. “Isotopologue Ratios of N2O Emitted from Microcosms with NH4 Fertilized Arable Soils under Conditions Favoring Nitrification.” Soil Biology & Biochemistry 40 (9): 2416–2426. doi:10.1016/j.soilbio.2008.06.003.
- Wlodarczyk, T., J. Glinski, and U. Kotowska. 2004. “N2O Emission from Mineral soils-Reviews.” Research in Agricultural Engineering 50 (3): 117–122. doi:10.17221/4937-RAE.
- Yang, L., Y. X. Ren, S. Q. Zhao, X. Liang, and J. Wang. 2016. “Isolation and Characterization of Three Heterotrophic nitrifying-aerobic Denitrifying Bacteria from a Sequencing Batch Reactor.” Annals of Microbiology 66 (2): 737–747. doi:10.1007/s13213-015-1161-7.
- Yang, L., X. Zhang, and X. Ju. 2017. “Linkage between N2O Emission and Functional Gene Abundance in an Intensively Managed Calcareous fluvo-aquic Soil.” Scientific Reports 7 (43283). doi:10.1038/srep43283.
- Yin, M., X. GaO, M. Tenuta, W. Kuang, D. Gui, and F. Zeng. 2019. “Manure Application Increased Denitrifying Gene Abundance in a drip-irrigated Cotton Field.” PeerJ 7 (e7894). doi:10.7717/peerj.7894.
- Zanatta, J. A., C. Bayer, F. C. B. Vieira, J. Gomes, and M. Tomazi. 2010. “Nitrous Oxide and Methane Fluxes in South Brazilian Gleysol as Affected by Nitrogen Fertilizers.” Soil Fertility and Plant Nutrition 34 (5): 1653–1665. doi:https://doi.org/10.1590/S0100-06832010000500018.
- Zhang, J., C. Müller, and Z. Cai. 2015. “Heterotrophic Nitrification of Organic N and Its Contribution to Nitrous Oxide Emissions in Soils.” Soil Biology & Biochemistry 84: 199–209. doi:10.1016/j.soilbio.2015.02.028.
- Zhu, X., M. Burger, T. A. Doane, and W. R. Horwath. 2013. “Ammonia Oxidation Pathways and Nitrifier Denitrification are Significant Sources of N2O and NO under Low Oxygen Availability.” Proceedings of the National Academy of Sciences of the United States of America, April 16, USA. 110: 6328–6333.
- Zhu, Y., L. Merbold, S. Leitner, L. Xia, D. E. Pelster, E. Diaz-pines, S. Abwanda, P. M. Mutuo, and K. Butterbach-Bahl. 2020. “Influence of Soil Properties on N2O and CO2 Emissions from Excreta Deposited on Tropical Pastures in Kenya.” Soil Biology & Biochemistry 140 (107636). doi:10.1016/j.soilbio.2019.107636.