ABSTRACT
The mechanisms underlying stabilization of soil organic matter (SOM) in vegetated coastal ecosystems, including mangrove forests, are poorly understood, limiting our ability to predict the consequences of disturbances. Here, we introduce density fractionation to mangrove soils to identify the distribution and properties of the functional components of SOM regarding degradation state, stability, and origin, namely, the free low-density fraction (f-LF), mineral-associated LF (m-LF), and high-density fraction (HF). Three soil cores (1 m) were collected in a mangrove forest on Ishigaki Island, Japan, and cut into 10 cm intervals and analyzed. Although HF was the most abundant, the massive production of mangrove fine roots resulted in a high abundance of LFs throughout the cores, which markedly differed from terrestrial soils. Relative abundance of LFs together accounted for 38–66% of total soil C. The m-LF was as abundant as f-LF and 1.6 times higher in relative abundance than the global average of terrestrial soils. The C/N ratios and δ13C values clearly increased with depth in all fractions, which was attributed to the increased contribution from roots. We found a consistent pattern in Δ14C values of density fractions. HF was the oldest with Δ14C between −149‰ and −97‰ followed by m-LF (between −130‰ and −87‰) and then f-LF (between −89‰ and 78‰), suggesting that mineral association may be pivotal in long-term carbon storage in the mangrove mineral soil. Our analysis successfully identified meaningful functional components of mangrove SOM, yet several questions remained unanswered, including a large variability in Δ14C in different cores. Future studies would benefit from a coupled analysis of the quantity and quality of density fractions and geochemical factors in mangrove soils.
1. Introduction
Mangrove forests exhibit a high capacity for soil organic matter (SOM) storage (Bouillon et al. Citation2008; Kristensen et al. Citation2008), with SOM accounting for about 75% of total carbon stocks in mangrove forests (Alongi Citation2014). The global average carbon stock in the top 1 m of mangrove forest soils is estimated to be 283 ± 193 Mg C ha−1 (Atwood et al. Citation2017). Furthermore, regardless of the soil type (i.e., peaty or mineral), the average carbon stock including deeper soils (~3 m) exceeds 900 Mg C ha−1, making mangrove forests among the most carbon-rich ecosystems in the tropics (Donato et al. Citation2011; Kida et al. Citation2021). Vegetated coastal ecosystems, known as ‘Blue Carbon’ ecosystems, including mangrove forests, are known to accumulate SOM at rates tens of times faster than terrestrial ecosystems (McLeod et al. Citation2011Alongi Citation2014)Ongoing climate change and anthropogenic disturbances such as deforestation, land reclamation, urbanization, and land use change pose significant threats to these ecosystems (Adame et al. Citation2021; Richards and Friess Citation2016). These disturbances can significantly impact carbon sequestration in coastal ecosystems and existing soil carbon pools. However, the mechanisms of SOM stabilization in these ecosystems are poorly understood, limiting our ability to predict the consequences of disturbances (Kida and Fujitake Citation2020).
Some stabilization mechanisms must be present for mangrove SOM to remain stable over the long-term. However, research on this topic is still scarce for mangrove soils and Blue Carbon ecosystems in general. While Blue Carbon studies have gathered significant information on the global C stocks and their regional variations in the last decade, a mechanistic understanding of SOM stabilization in these systems has been comparatively much less developed (Kida and Fujitake Citation2020). Anoxia has been considered a dominant factor in SOM stabilization in these vegetated coastal ecosystems. Certain organic compounds, particularly lignin, are degraded less efficiently in the absence of oxygen, and it is most likely the primary reason behind the millennial-scale accumulation of mangrove peat on oceanic islands (McKee, Cahoon, and Feller Citation2007). However, evidence is accumulating that SOM in mineral soils of vegetated coastal ecosystems consists of a myriad of different organic compounds (Dodla, Wang, and Cook Citation2012; Kida, Kondo, et al. Citation2019; Santín et al. Citation2008; Zhang et al. Citation2016). Previous studies have shown that labile organic components including many small organic compounds, tissues of micro autotrophs such as algae and phytoplankton, and fresh plant litter degrade at similar rates regardless of the presence or absence of oxygen (Lee Citation1992). Therefore, factors contributing to the stabilization of these otherwise labile compounds in mangrove mineral soils are of particular interest.
Several common mechanisms underlying SOM stabilization have been identified in terrestrial soils and marine sediments. Broadly, these include: (1) recalcitrance of organic matter due to its chemical structural properties, (2) physical protection (inaccessibility) of organic matter from microbial degradation within aggregates and pore spaces, and (3) chemical interactions with soil minerals and metals (Sollins, Homann, and Caldwell Citation1996). The physical and chemical stabilization of SOM reduces its availability to microorganisms and enzymes (Lützow et al. Citation2006; Marschner et al. Citation2008). Vegetated coastal soils, located in the transitional zone between terrestrial and marine environments, may also experience the same mechanisms of SOM stabilization. However, contrasting evidence has been found regarding the potential mechanisms that contribute to SOM stabilization in these ecosystems (). It is important to investigate whether physical and chemical stabilization of potentially labile organic matter is also present in mangrove mineral soils and coastal sediments, but few related studies have been conducted to date (Dicen et al. Citation2019; Shields et al. Citation2016; Zhao et al. Citation2018).
Table 1. Mechanisms that contribute to stabilization of soil organic matter (SOM) in coastal vegetated soils and evidence for and against each mechanism.
Density fractionation, which has been used by soil scientists for nearly 50 years, physically fractionates SOM into functional fractions of varying characteristics using a heavy liquid based on particle density (Crow et al. Citation2007). Chemical and molecular analysis of organic matter after density fractionation allows for the acquisition of higher-resolution data about the spatiotemporal distribution and properties of the functional components of SOM regarding stability and origin. The particle density of freshly incorporated, plant-derived particulate organic matter is less than 1.6 g cm−3, considerably smaller than that of soil minerals (2–4 g cm−3). As a result, density fractionation yields two fractions based on density: the low-density fraction (LF, also known as particulate organic matter) and high-density fraction (HF, also known as mineral-associated organic matter). The interaction between organic matter and minerals increases as the microbial degradation of plant-derived LF progresses. LF can be further divided into free LF (f-LF), a roughly mineral-free fraction consisting mainly of fresh, coarse organic materials such as plant residues, and mineral-associated LF (m-LF) which is LF attached to or embedded in soil minerals or aggregates, by disruption of soil aggregates and mineral association through mechanical shaking with glass beads or ultrasonication (Wagai, Mayer, and Kitayama Citation2009). Conceptually, f-LF is labile and fast-cycling due to the lack of protection by minerals, while HF is the most persistent, cycled at centuries to millennium time-scale, due to physico-chemical protection by soil mineral matrix, and m-LF often exhibits properties intermediate between the two (Wagai, Mayer, and Kitayama Citation2009). Density fractionation thus physically divides bulk SOC into pools directly associated with specific mechanisms and processes that affect its decomposition rate (Heckman et al. Citation2022). While density fractionation has been used in other coastal ecosystems such as seagrass meadows (Miyajima et al. Citation2017), it has not yet been applied to mangrove soils. Considering the massive production and turnover of fine roots in mangroves soils and their major contribution to SOM accumulation (Arnaud et al. Citation2021; Liu, Xiong, and Liao Citation2017; Muhammad-Nor et al. Citation2019) and recently proposed possible roles of soil physico-chemical factors in stabilizing SOC in vegetated coastal ecosystems (Dicen et al. Citation2019; Kida and Fujitake Citation2020; Shields et al. Citation2016; Zhao et al. Citation2018), density fractionation appears particularly useful in studying SOM stabilization mechanisms in mangrove soils.
The aim of this study was to identify the quantitatively important fractions for carbon storage in mangrove soils using density fractionation, to examine the differences in organic matter characteristics by fraction and depth, and to estimate the origin of organic matter in each density fraction. Previous research has shown that approximately 80% of fine root biomass is found within the top 30 cm of terrestrial soils (Hashimoto and Hyakumachi Citation1998), while in mangrove forests, fine root production is likely much greater and fine roots are widely distributed to deeper depth (Arnaud et al. Citation2021; Tabuchi Citation1983). Therefore, we hypothesized that f-LF is more abundant in mangrove soils compared to terrestrial soils. We also hypothesized that HF exhibits more non-mangrove (microbes or marine origin) signatures compared to other LFs because HF can capture other sources in water through organo-mineral interactions (Kida and Fujitake Citation2020).
2. Materials and methods
2.1. Study area and sampling
Samples were collected in a mangrove forest located along the Fukido River on Ishigaki Island, Okinawa Prefecture, Japan (24°29’N, 124°13’ E, ). This forest covers an area of approximately 19 ha around the mouth of the Fukido River (Kida, Tanabe, et al. Citation2019), and are dominated by two mangrove species, Bruguiera gymnorrhiza and Rhizophora stylosa. The study area has a subtropical monsoon climate, with an average annual precipitation of 2107 mm and an average annual temperature of 24.3°C from 1981 to 2010 (Ishigaki Island Local Meteorological Observatory, Japan Meteorological Agency). The watershed (approximately 2.4 km2) receives little human activity, and broadleaf forests occupy about 95% of the area, with the rest of land use being sparse sugar cane and paddy fields. The soil in the catchment is red-yellow soil (Ultisols) with a thin A horizon and low SOM content (Kida, Tanabe, et al. Citation2019). A previous study has detailed the species composition, biomass, and aboveground net primary productivity of this mangrove forest (Ohtsuka et al. Citation2019). The Fukido mangrove exhibits a clear semidiurnal tide, with maximum tidal height reaching over 1 m at spring tide (Ohtsuka et al. Citation2019). At high tide, the area is inundated with seawater, whereas at low tide, the soil surface is exposed to the air. The soil is mineral and tentatively classified as gley soil. The mineral composition within the mangrove forest is spatially relatively constant, with only a minimal contribution from calcite, indicating that the minerals were mainly derived from the catchment (Kinjo et al. Citation2005).
Figure 1. Map of the Fukido River mangrove forest site. The white square in the left panel indicates the 80 m × 80 m permanent quadrat, while the right panel shows the quadrat with sampling points denoted with numbers. DBH = diameter (in cm) at breast height.
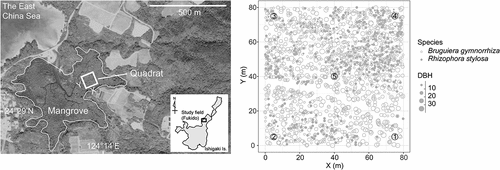
Soil samples were collected in August 2015 at five points within a permanent quadrat (80 m × 80 m) established at the site in 2014 (Ohtsuka et al. Citation2019) using an open-face stainless core sampler with minimum compaction (1 m long, 27 cm2 cross-sectional area; Handy GeoSlicer, Fukken Research and Design Co.) (). The cores were cut into 10 cm intervals at the field using a metal spatula and transported to the laboratory under cool, dark conditions. Upon arrival, the cores were immediately air-dried at 60°C until a constant weight was obtained, and bulk density was determined (Donato et al. Citation2011; Kida, Kondo, et al. Citation2019). The air-dried soil was then passed through a 2 mm mesh size sieve. No gravel was present in any of the samples. Soil texture is sandy clay loam with relative sand content of 60–70% (Kida, Kondo, et al. Citation2019). A leaf and fine root of Bruguiera gymnorrhiza, which was the dominant species in the quadrat (Ohtsuka et al. Citation2019), were also collected from each of four different standing trees. These samples were dried at 60°C and finely ground using a white porcelain mortar and pestle.
In this study, soil samples from the cores 2, 3, and 5, the deepest of the five cores, were analyzed (). Approximately 5.5 g of the air-dried samples were carefully subsampled using the conical quadrant method to ensure representative subsamples. The samples from a depth of 70–94 cm at the point 3 were treated with 2 M HCl overnight to remove inorganic carbon because some shell fragments were visually observed. The treated sample was carefully collected using a metal spoon and analyzed for density fractionation.
2.2. Density fractionation
Density fractionation was performed using an aqueous solution of sodium polytungstate (SPT-0, TC-Tungsten Compounds; SPT) with a density of 1.6 g cm−3 in accordance with previous studies (Golchin et al. Citation1994; Wagai et al. Citation2008). Despite the assumption that SPT readily produces insoluble precipitates in the presence of calcium ion and thus cannot be used in Ca-rich soils without prior washing, little literature evidence was found to support this argument. In fact, the original support for this notion appeared to be pers. comm. reported in Six (Citation1999) (Six Citation1999). We thus first examined whether marine-derived Ca2+ in mangrove soil samples interferes with the density fractionation experiment using two types of solutions: (1) a filtrate by 0.45 µm PTFE membrane filter (Omnipore, Merck) of one of the Fukido mangrove soil samples mixed with deionized water with approximately twice the solid-to-liquid ratio as the density fractionation experiment, and (2) a 0.6 M CaCl2 solution. These solutions were each mixed with the same volume of a 3.2 g cm−3 SPT solution, making a 1.6 g cm−3 SPT solution with Ca2+ concentrations representative of the Fukido samples and unlikely high 0.3 M, respectively. In both cases, no precipitates were found after 24 h. With 0.3 M CaCl2, a small amount of white precipitates (presumably Ca-PT) was observed only after 72h. The density of the supernatants remained unchanged during these experiments when there were no precipitates. We thus concluded that a prior desalinating washing step was not necessary for mangrove soils and coastal sediments in general. Omitting the washing step can alleviate the risk of material loss and saves time.
In this study, m-LF was collected through mechanical shaking with glass beads (φ6 mm) at 120 rpm, which facilitated the breakdown of aggregates and detachment of minerals attached to plant residues. We optimized the duration of shaking by comparing m-LF recovery with that obtained through sonication (). Approximately 90% recovery was achieved through 24 h of shaking compared to sonication with a total energy of 120 J mL−1 in ice water, which itself showed maximal recovery of m-LF (). The m-LF recovery through shaking also reached a plateau after 24 h, thus this duration was selected for the shaking process.
Figure 2. Comparison of mineral-associated low-density fraction (m-LF) recovery by sonication (a) and mechanical shaking (b). Surface (triangle), medium (square), bottom (circle) samples of the core 3 from the fukido mangrove soils used in the study were tested, where the corresponding line types in (a) and (b) represent the identical samples.
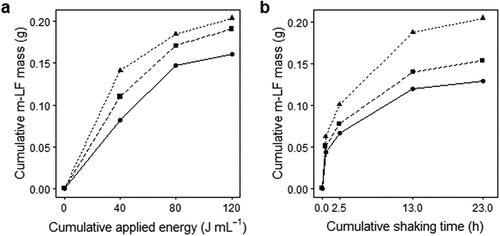
In density fractionation, 5 g of the soil sample was weighed in a 50 mL conical tube and 20 mL of a SPT solution was added, followed by gentle turning over 20 times. The sample was then centrifuged at 700 G for 5 min, and the suspended materials were collected as f-LF using a poly dropper and metal spoon onto a suction filtration device with a 0.45 µm PTFE membrane filter (Omnipore, Merck). This procedure was performed three times in total. In order to compensate for the SPT solution lost during the f-LF collection, the SPT solution was replenished every procedure. The collected f-LF was washed three times with 5 mL of 1M KCl to prevent possible inorganic nitrogen contamination from the SPT solution (Rota Wagai, pers. comm.), rinsed with deionized water until the electrical conductivity (EC) was less than 50 μS cm−1, and dried at 80°C for 48 hours. Subsequently, glass beads and the SPT solution were added to the remaining soil, and the sample was shaken reciprocally at 120 rpm for 24 hours. The sample was then centrifuged at 8700 G for 10 min, and the material floating (m-LF) was recovered using the same procedure as the f-LF recovery. Finally, the sample residue in the conical tube (HF) was transferred to a 250 mL centrifuge tube with deionized water, centrifuged at 13,000 G for 20 min, and the supernatant was carefully discarded as much as possible using a Komagome pipette. Glass beads were removed with a care not to lose any sample soil after centrifugation. The soil was then treated with 100 mL of 1M KCl (shaken reciprocally for 10 min and centrifuged at 13,000 G for 25 min), washed several times with 100 mL of deionized water until the EC reached less than 50 μS cm−1, and freeze-dried. The weight of each fraction was measured and mass recovery was calculated. Using a stereomicroscopy (×20), we observed the morphology of each density fraction. Photographs of representative samples were taken using density fractionation samples from another nearby mangrove forest on Ishigaki Island (m-LF collected by sonication) and provided in because we couldn’t take photographs of Fukido samples. The morphological features of the density fractions from these mangroves were almost identical.
Figure 3. Contribution of each density fraction to total soil mass (partitioned from 5 g) (a) and C and N concentrations of each fraction on fraction basis (b, c) with depth. The stereomicroscopic photographs (×40) of representative samples of each fraction are also provided in (d). Note the different x-scale between fractions. In (a), the x-axis of f-LF and m-LF was magnified by a factor of five compared to that of HF, while in (b) and (c), the x-axis of HF was magnified by a factor of five. f-LF: free low-density fraction, m-LF: mineral-associated low-density fraction, and HF: high-density fraction.
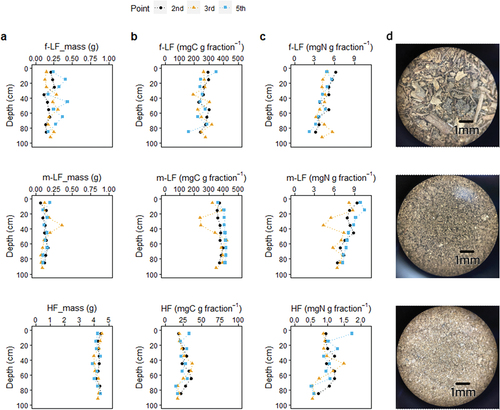
2.3. Elemental analysis
The elemental composition of bulk soil, each density fraction, leaves, and fine roots was determined using an elemental analyzer (PE2400 series II; PerkinElmer). Following grinding and drying at 80°C for 12 h, the soil samples were sealed in tin capsules (Tin Capsule Foil, 8 × 5 mm, Exeter Analytical Inc.). Bulk soil and HF fractions were encapsulated in approximately 15 mg, whereas the organic materials including LF fractions were encapsulated in approximately 2–4 mg. Measurements were performed in duplicate and the average value was used for the results. If the coefficient of variation (CV) between two measurements exceeded 10%, a third measurement was performed and the most outlier was removed. The overall analytical precision for C and N was 1.2% and 2.5% by CV, respectively. From the obtained C and N content, the mass recovery of C and N by density fractionation, the contribution of each density fraction to the bulk C and N content, and the C/N ratio were calculated.
2.4. Stable carbon isotope analysis
In mangrove soils, stable carbon isotopes have been used to identify major carbon sources of SOM, to investigate patterns of organic carbon utilization by microbial and animal communities, and to track organic matter exchange between adjacent ecosystems (Bouillon, Connolly, and ShingY Citation2008), but never for density fractions. In this study, carbon stable isotope ratios were measured for each density fraction using a continuous flow elemental analyzer/isotope ratio mass spectrometer (EA/IRMS; FLASH EA 1112 series + Thermo Finngan DELTA plus, Thermo Scientific, U.S.A.). The carbon stable isotope ratios were expressed in the common delta (δ) notation as the per mil (‰) difference of the13C/12C ratio in a sample relative to the Vienna Pee Dee Belemnite standard. Peach Leaves (δ13C: −26.06‰ ± 0.05‰, NIST1547, Sigma-Aldrich) and Glycine (δ13C: −32.3‰ ± 0.2‰, Aminostandard, Shoko Science) were used as calibration standards. To check the scale-dependent variation of the δ13C values, the amounts of the standards were varied from 0.03 to 0.07 mg. The calibration curve using the Peach Leaves and Glycine standards was prepared at approximately 0.07 mg and 0.03 mg, respectively, to correct for the deviation of the measured values from the true values of the standard samples. A 5-point calibration with the standards was used to calibrate and normalize the measured isotopic ratios to the international scale. Two standards were run for every 20 samples, and 2 blanks and conditioning and calibration standards were included at the beginning and end of each run. Measurement precision and trueness were both within ± 0.14‰ for δ13C of the laboratory standards.
2.5. Radiocarbon analysis
Radiocarbon analysis of density fractions at the deepest part of each core were performed at Yokoyama Lab, AORI, Japan (Yokoyama et al. Citation2019). We provided approximately 3 mg C for radiocarbon analysis. All samples were sealed in Ag capsules, combusted into CO2 gas in an elemental analyzer (Vario Micro Cube, Elementar), and converted to graphite by a custom-built graphitization vacuum line (Yokoyama et al. Citation2022). After graphitization, the radiocarbon content was measured with a single-stage accelerator mass spectrometer (NEC, U.S.A.). Radiocarbon data are expressed as14C ages, percent modern carbon (pMC), and Δ14C which is the fractional deviation, in parts per thousand (‰), of the sample14C/12C ratio relative to that of the oxalic acid international standard (National Institute of Standards and Technology) (Stuiver and Polach Citation1977). Analytical precision for the Δ14C analysis was better than 4‰.
3. Results and discussion
3.1. General characteristics and microscopic observations of soil density fractions
The evaluation of material recovery is a crucial initial step in reporting the results of density fractionation. Our density fractionation yielded an average soil mass recovery as high as 92.2% (). The recoveries based on C and N content were also high, albeit slightly lower than those of the mass recovery (), which are commonly observed in density fractionation analysis of soils. The slight loss could be due to the loss of dissolved matter and fine colloids during the washing step (Wagai et al. Citation2008). Additionally, a higher loss of N might have resulted from the loss of inorganic N. Overall, these high material recovery rates assured the validity of our approach.
Table 2. Mass recovery rate and weight recovery rate of C and N relative to the mass.
HF was by far the most dominant fraction in the mangrove soils in terms of mass (). The average mass proportions of f-LF, m-LF, and HF in the bulk soils were 4.9%, 2.9%, and 92.2%, respectively. Yet, the average percentage of the f-LF and m-LF combined (~8%) was higher than that of forest and agricultural soils (Cerli et al. Citation2012; Crow et al. Citation2007; Kölbl and Kögel‐Knabner Citation2004; Parker et al. Citation2002; Swanston et al. Citation2005; Wagai et al. Citation2008). The amounts of f-LF did not show a clear change with depth and varied largely (). The m-LF content showed much less variation with depth but had a notably high value at 30–40 cm in core 3, accompanied by reductions in the C and N concentrations (. The HF content was almost constant with depth and between cores. According to stereomicroscopic observation, materials recovered as f-LF were mainly coarse plant residues, with relatively intact, undecomposed mangrove fine roots found in shallower (<50 cm) sections while more fragmented fine roots and bark of coarser roots in deeper sections (still, live fine roots were present in the deepest sections) (). Much of the plant residues in f-LF was covered with patches of fine mineral particles. In contrast, m-LF was almost free of mineral particles and consisted of much smaller plant fragments. The use of glass beads and mechanical shaking may have largely broken plant tissues and obscured the morphology. However, m-LF recovered by disruption of aggregates by sonication consisted of similarly small fragments (), thus this fragmented morphology may be a property of m-LF in mangrove soils. As expected, HF contained no recognizable plant tissues and was instead dominated by mineral particles ().
The C and N concentrations of the density fractions () were consistent with the microscopic observations. C concentrations were one-order of magnitude higher in LF than in HF, while N concentrations were several times higher. Among LF, m-LF had almost always higher C and N concentrations compared to f-LF (). Nitrogen was relatively more enriched (by ~80%) than C (by ~50%) in m-LF than in f-LF. Higher C and N concentrations in m-LF compared to f-LF have been found in forest and agricultural soils (Wagai et al. Citation2008 and references there in). Interestingly, only N concentrations on fraction basis showed a clear decline with depth in all fractions (). This decoupling between C and N dynamics suggests selective consumption or leaching of N-rich compounds and/or selective preservation of C-rich compounds.
When evaluating on a bulk soil basis, the C concentrations (mgC g soil−1) in all fractions varied widely and did not show a clear depth trend, while the N concentrations decreased with depth, particularly in the m-LF and HF fractions (). The depth distributions of LFs were markedly different from those typically observed in terrestrial soils which tend to decrease rapidly with depth (Luo et al. Citation2020; Parker et al. Citation2002; Swanston et al. Citation2005). This is presumably due to the massive production of mangrove fine roots, even in deep soils. Although data on in-situ fine root production in deep mangrove soils (>30 cm) is scarce, Arnaud et al. (Citation2021) recently showed that a major fraction of fine root production occurs deeper than 30 cm. Other recent papers have also reported fine root production down to a depth of 50–60 cm below ground in mangrove forests (Fujimoto et al. Citation2021; Ono et al. Citation2022). Our visual inspection also revealed abundant live and dead fine roots in deep (> 50cm) soils. These results collectively support our first hypothesis.
Figure 4. Contribution of each density fraction to total soil C (a, c) and N (b, d) content with depth. Results are presented in C and N concentrations of each fraction on bulk soil basis (a, b) and relative abundance of each fraction in bulk C and N abundance (c, d). The vertical lines in (C) represent means (solid line) and one standard errors (dashed line) of the relative abundance of density fractions in 1222 terrestrial soils reported by Heckman et al. (Citation2022). f-LF: free low-density fraction, m-LF: mineral-associated low-density fraction, and HF: high-density fraction.
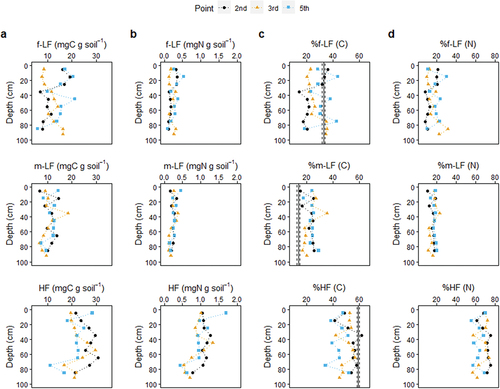
When normalized to the total, f-LF accounted for 14–44% of total soil C and 8–32% of total N, m-LF for 15–36% and 11–23%, and HF for 34–62% and 56–75%, respectively (). HF was therefore the most abundant form of SOM in the mangrove soils, and its contribution was more prominent as N. All fractions did not exhibit a clear depth trend, while the variations of %m-LF (CV of 18% and 17% for C and N, respectively) were relatively smaller than that of %f-LF (CV of 28% and 39% for C and N, respectively). A recent comprehensive meta-analysis of data obtained by density fractionation of terrestrial soils (n=1222) (Heckman et al. Citation2022) reported that the mean contribution of f-LF, m-LF, and HF to the total soil C was 33%, 14%, and 59%, respectively, indicating m-LF is the minimal functional component of SOM in terrestrial soils. Contrarily, our analysis revealed that m-LF was as abundant as f-LF (particularly as N) in the mangrove soils throughout the 1-m cores (). The average relative contribution of m-LF carbon in the mangrove soils was 1.6 times higher than that in terrestrial soils (Heckman et al. Citation2022). In mangrove soils, the longer residence time of plant residues (f-LF, mainly as fine roots) under suboxic conditions may have resulted in greater associations with soil minerals, providing physico-chemical protection for such plant residues from microbial degradation.
3.2. Organic matter early diagenesis inferred from C/N ratios and δ13C values
The C/N ratios of the density fractions showed clear differences between fractions (). The C/N ratios of the f-LF, m-LF, and HF ranged from 43.2 to 78.5, 32.0 to 66.8, and 16.8 to 33.8, respectively (). Those of leaves and fine roots of Bruguiera gymnorrhiza ranged from 31.6 to 42.6 (mean ± standard deviation of 36.0 ± 4.9, n = 4) and 47.9 to 57.6 (52.3 ± 4.3, n = 4), respectively. The lower C/N ratio of HF compared to the other low-density fractions is similar to that observed in terrestrial soils, suggesting that microbial degradation of HF was more progressed and that plant-derived components contributed more to the LFs (Liao, Boutton, and Jastrow Citation2006; Tan et al. Citation2007). There was no obvious difference in δ13C values between fractions (overall −29.7 to −27.3‰) (), indicating that the major origin of organic matter differed only little among the fractions. Previous studies have shown that mangrove tissues, microalgae, macroalgae, and seagrasses are important sources of SOM in mangroves (Bouillon, Connolly, and ShingY Citation2008). Their average δ13C values are −28.1‰, −20.2‰, −18.9‰, and −12.1‰, respectively (Bouillon, Connolly, and ShingY Citation2008), suggesting all fractions were primarily derived from mangroves in the Fukido mangrove forest. The little difference in δ13C values between density fractions was against commonly observed patterns in terrestrial soils, where organic matter strongly associated with minerals (i.e., HF) is typically enriched in13C (Sollins et al. Citation2009), and rejected our second hypothesis.
Figure 5. C/N ratio and δ13C stable isotope ratio of each fraction with depth. f-LF: free low-density fraction, m-LF: mineral-associated low-density fraction, and HF: high-density fraction. The vertical dashed lines in the δ13C results represent the average δ13C values for leaf and root of mangroves in the fukido mangrove forest reported by Iimura et al., (Citation2019).
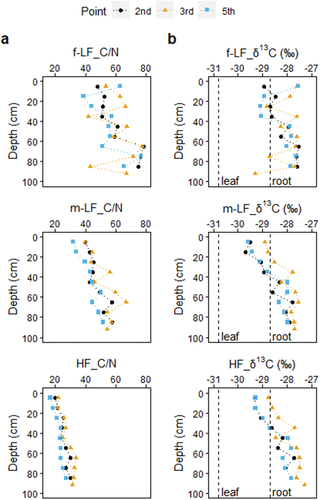
The C/N ratio clearly increased with depth in all density fractions (). At the same time, the δ13C values also showed clear increases with depth (). The simultaneous increases in the C/N ratios and δ13C values with depth were against commonly observed patterns in terrestrial soils and not straightforward to interpret. In terrestrial soils under C3 plants, C/N ratios generally decrease while δ13C values increase with depth, resulting in a negative correlation between them (Lorenz et al. Citation2020; Paul, Balesdent, and Hatté Citation2020; Sollins et al. Citation2009; Werth and Kuzyakov Citation2010). Although inorganic carbon can theoretically raise C/N ratios and δ13C values, we could rule out a contribution from inorganic carbon because the three samples that were subjected to a HCl treatment (70–94 cm at station 3) showed similar C and N concentrations, C/N ratios, and δ13C values compared to the rest of untreated samples (). A HCl test on randomly selected samples with no visible inorganic carbon fragments (such as shells or coral fragments) was indeed negative.
The reasons for the enrichment in13C with depth are not yet fully understood, but the13C enrichment can be the result of temporal changes in the initial composition of C or isotopic fractionation associated with post-photosynthesis processes in either plants or soils. We summarized possible processes that can affect C/N ratios and δ13C values of SOM (). A range of processes is known to influence both parameters in either direction. Among these, only the increased relative dominance of roots compared to leaves could explain the simultaneous increases in the C/N ratios and δ13C values with depth, although all processes are not mutually exclusive (). The proportion of root-derived C inputs is expected to be higher at depth, and roots generally have higher C/N ratios (by ~5–50) and δ13C values (by ~1–5‰) compared to leaves because of differences in chemical composition and post-photosynthetic allocation mechanisms (Cernusak et al. Citation2009; Lorenz et al. Citation2020; Werth and Kuzyakov Citation2010; Zeh et al. Citation2020). Our data for Bruguiera gymnorrhiza were consistent with this general trend; the C/N ratio of fine roots was higher by approximately 16 than leaves. Furthermore, decomposed mangrove roots can exhibit a high C/N ratio. For instance, after one-year of decomposition, mangrove leaves decreased in C/N ratio (from 32 to 18) while roots considerably increased it (from 36 to 66) in a mangrove forest on Pohnpei Island (Ono et al. Citation2015). In this regard, the decomposition of roots alone might explain the simultaneous increases in the C/N ratios and δ13C values with depth () if13C kinetic discrimination during microbial utilization favors the enrichment of13C in residual roots (Ågren, Bosatta, and Balesdent Citation1996; Torn et al. Citation2002). Previous research has shown that the δ13C values of mangrove leaves and fine roots in the Fukido mangrove were on average −30.9‰ and −28.7‰, respectively (), indicating that roots had a heavier carbon isotope signature than leaves (Iimura et al. Citation2019). However, a slight (~1.5‰) but consistent deviation from the simple leaf-to-root mixing model () suggests an input of13C-enriched materials, such as soil microbial-derived organic matter and marine organic matter, regardless of depth or fractions () (Boström, Comstedt, and Ekblad Citation2007; Marchand et al. Citation2005; Werth and Kuzyakov Citation2010). Nonetheless, the marked difference in the C/N ratios between fractions () indicates that different density fractions have undergone distinct degradation state and potentially have different ages.
Table 3. Processes that can change C/N ratios and δ13C values of soil organic matter under C3 plants. The direction of change is expressed as downward change in a soil column.
Selective preservation of phenolic compounds reported in this mangrove forest (Kida, Kondo, et al. Citation2019) could explain the increase in C/N ratios (), but that would in turn lead to depleted δ13C values because lignin typically is depleted in13C compared to other plant constituents such as proteins and cellulose (Bowling, Pataki, and Randerson Citation2008). Isotope analysis of plant organic compounds, as well as end-members such as terrestrial, mangrove, and marine sources, could provide further insight into the simultaneous increases in the C/N ratios and δ13C values with depth (). Finally, fractionation of dissolved organic carbon (DOC) can also partly account for the depth gradients in C/N ratios and δ13C (Bowling, Pataki, and Randerson Citation2008; Kaiser et al., Citation2001). In terrestrial soils, material flow is typically ‘top-down’ due to major organic matter inputs in surface soils and subsequent translocation down to subsurface soils through water flow (Heckman et al. Citation2022). During passage through the mineral soil, DOC interacts with the mineral matrix through preferential sorption/desorption of compounds with specific molecular characteristics as in chromatography. These chromatographic behaviors and the decay of labile compounds can alter the molecular signatures of DOM, and in extension, associated bulk SOM because of differences in C/N ratios and δ13C values among compounds (Bowling, Pataki, and Randerson Citation2008). However, the influence of DOC on the molecular signature of bulk SOM in mangrove soils is unknown, as material flow in these soils is not only vertical but also horizontal due to organic matter inputs throughout the soil column (~1 m) through massive fine root production (Arnaud et al. Citation2021; Tabuchi Citation1983) and advective translocation of DOC in soils by tidal water movement (Maher et al. Citation2013; Ohtsuka et al. Citation2020).
3.3. Mineral association as a key factor in long-term carbon storage in mangrove mineral soils
The major contribution of m-LF in mangrove soils () compared to terrestrial soils was a novel finding, but its long-term stability needs verification for m-LF, as well as HF, to be an important fraction for carbon storage in mangrove soils. We therefore conducted a radiocarbon analysis of density fractions in the deepest samples. We found a consistent pattern in Δ14C values of density fractions in all the measured cores (). HF was the oldest with Δ14C between −149‰ and −97‰ followed by m-LF (between −130‰ and −87‰) and then f-LF (between −89‰ and 78‰). These differences in Δ14C among density fractions were consistent with the findings from terrestrial soils (Heckman et al. Citation2022) and suggest that mineral association may be pivotal in long-term carbon storage in mangrove mineral soils. In the LFs, m-LF was always older than the corresponding f-LF, although f-LF showed a considerable variability in their Δ14C (). The lower Δ14C values of m-LF indicate that on average, m-LF is more persistent than f-LF. Together with the relative enrichment of m-LF, our findings suggest that m-LF may play a more important role in carbon storage in mangrove soils than in terrestrial soils. It is likely that slower decomposition under reducing conditions due to flooding resulted in longer residence time of f-LF and greater associations with soil minerals. The positive Δ14C (modern age) observed in f-LF of the core 3 was due to recent inputs of live fine roots, while negative Δ14C values of f-LF in the other cores suggest limited inputs from live roots (). Questions remained, however, why there were a large variability in Δ14C between the same fractions of different cores (). Molecular-level analyses of each fraction and more detailed source partitioning by measuring end-members (river, mangrove, and marine) and linking the result to geochemical factors such as specific surface area and reactive metal phases (Fe, Al), may shed light on organic carbon stabilization mechanisms in mangrove soils. A variability in environmental factors such as oxidation-reduction potential, pH, or salinity should simultaneously be considered.
Table 4. Radiocarbon (± 1σ) of the density fractions of fukido mangrove soil cores. The numbers after ‘FUK’ represent the soil core numbers and depths of the sections analyzed.
4. Conclusion
We introduced density fractionation to mangrove soils in this study. The method could successfully separate meaningful functional components of SOM in mangrove soils which differed in abundance, degradation state, and age. The massive production of mangrove fine roots resulted in a high abundance of plant debris (low-density fractions) throughout the 1-m cores, which markedly differed from terrestrial soils. By analyzing elemental and isotopic signatures of density fractions, we revealed shifts in sources and degradation states within and between fractions. Although we were able to show that mineral associated fractions were the most important for carbon storage in mangrove soils both in terms of quantity and residence time, the processes generating organic matter in each density fraction and their influencing factors remain to be studied. Future studies would benefit from a coupled analysis of quantity (C/N concentrations and relative abundance) and quality (stable and radio isotopes and molecular composition) of density fractions and geochemical factors in mangrove soils. It is also needed to elucidate how natural environmental variations such as redox conditions and pH influence the association between mineral particles and reactive metal phases and SOM of different nature, on different time scales of hourly, daily, and seasonally over semi-diurnal and spring – neap tidal cycles.
Author contributions
MK designed the experiment and collected the samples with the help of NF. KH conducted the density fractionation experiments with an initial guidance from MK. MK and KH conducted stable isotope analysis with the help of TO. MK, YM, and YY conducted radiocarbon analysis. KH wrote an initial draft with a significant contribution from MK, and all authors have reviewed and approved the final article.
Acknowledgments
We thank Rota Wagai (NIAES/NARO) for his initial guidance in density fractionation.
Disclosure statement
No potential conflict of interest was reported by the author(s).
Additional information
Funding
References
- Adame, M. F., R. M. Connolly, M. P. Turschwell, C. E. Lovelock, T. Fatoyinbo, D. Lagomasino, and L. A. Goldberg. 2021. “Future carbon emissions from global mangrove forest loss.” Global Change Biology 27 (12): 2856–2866. https://doi.org/10.1111/gcb.15571.
- Ågren, G. I., E. Bosatta, and J. Balesdent. 1996. “Isotope Discrimination During Decomposition of Organic Matter: A Theoretical Analysis.” Soil Science Society of America Journal 60 (4): 1121–1126. https://doi.org/10.2136/sssaj1996.03615995006000040023x.
- Alongi, D. M. 2014. “Carbon Cycling and Storage in Mangrove Forests.” Annual Review of Marine Science 6 (1): 195–219. https://doi.org/10.1146/annurev-marine-010213-135020.
- Arnaud, M., P. J. Morris, A. J. Baird, H. Dang, and T. T. Nguyen. 2021. “Fine Root Production in a Chronosequence of Mature Reforested Mangroves.” New Phytologist 232 (4): 1591–1602. https://doi.org/10.1111/nph.17480.
- Atwood, T. B., R. M. Connolly, H. Almahasheer, P. E. Carnell, C. M. Duarte, C. J. Ewers Lewis, X. Irigoien, et al. 2017. “Global Patterns in Mangrove Soil Carbon Stocks and Losses.” Nature Climate Change 7 (7): 523–528. https://doi.org/10.1038/nclimate3326.
- Boström, B., D. Comstedt, and A. Ekblad. 2007. “Ekblad a 2007: Isotope Fractionation and 13C Enrichment in Soil Profiles During the Decomposition of Soil Organic Matter.” Oecologia 153 (1): 89–98. https://doi.org/10.1007/s00442-007-0700-8.
- Bouillon, S., A. V. Borges, E. Castañeda-Moya, K. Diele, T. Dittmar, N. C. Duke, E. Kristensen, et al. 2008. “Mangrove Production and Carbon Sinks: A Revision of Global Budget Estimates.“ Global Biogeochemical Cycles 22. https://doi.org/10.1029/2007GB003052.
- Bouillon, S., R. M. Connolly, and L. ShingY. 2008. “Organic Matter Exchange and Cycling in Mangrove Ecosystems: Recent Insights from Stable Isotope Studies.” Journal of Sea Research 59 (1–2): 44–58. https://doi.org/10.1016/j.seares.2007.05.001.
- Bowling, D. R., D. E. Pataki, and J. T. Randerson. 2008. “Carbon Isotopes in Terrestrial Ecosystem Pools and CO 2 Fluxes.” New Phytologist 178 (1): 24–40. https://doi.org/10.1111/j.1469-8137.2007.02342.x.
- Cerli, C., L. Celi, K. Kalbitz, G. Guggenberger, and K. Kaiser. 2012. “Separation of Light and Heavy Organic Matter Fractions in Soil — Testing for Proper Density Cut-Off and Dispersion Level.” Geoderma 170:403–416. https://doi.org/10.1016/j.geoderma.2011.10.009.
- Cernusak, L. A., G. Tcherkez, C. Keitel, W. K. Cornwell, L. S. Santiago, A. Knohl, M. M. Barbour, et al. 2009. “Why are Non-Photosynthetic Tissues Generally 13C Enriched Compared with Leaves in C3 Plants? Review and Synthesis of Current Hypotheses.” Functional Plant Biology 36 (3): 199. https://doi.org/10.1071/FP08216.
- Chen, C., S. J. Hall, E. Coward, and A. Thompson. 2020. “Iron-Mediated Organic Matter Decomposition in Humid Soils Can Counteract Protection.” Nature Communications 11 (1): 2255. https://doi.org/10.1038/s41467-020-16071-5.
- Crow, S. E., C. W. Swanston, K. Lajtha, J. R. Brooks, and H. Keirstead. 2007. “Density Fractionation of Forest Soils: Methodological Questions and Interpretation of Incubation Results and Turnover Time in an Ecosystem Context.” Biogeochemistry 85 (1): 69–90. https://doi.org/10.1007/s10533-007-9100-8.
- Dicen, G. P., I. A. Navarrete, R. V. Rallos, S. G. Salmo, and M. C. A. Garcia. 2019. “The Role of Reactive Iron in Long-Term Carbon Sequestration in Mangrove Sediments.” Journal of Soils & Sediments 19 (1): 501–510. https://doi.org/10.1007/s11368-018-2051-y.
- Dodla, S. K., J. J. Wang, and R. L. Cook. 2012. “Molecular Composition of Humic Acids from Coastal Wetland Soils Along a Salinity Gradient.” Soil Science Society of America Journal 76 (5): 1592–1605. https://doi.org/10.2136/sssaj2011.0346.
- Donato, D. C., J. B. Kauffman, D. Murdiyarso, S. Kurnianto, M. Stidham, and M. Kanninen. 2011. “Mangroves Among the Most Carbon-Rich Forests in the Tropics.” Nature Geoscience 4 (5): 293–297. https://doi.org/10.1038/ngeo1123.
- Francey, R. J., C. E. Allison, D. M. Etheridge, C. M. Trudinger, I. G. Enting, M. Leuenberger, R. L. Langenfelds, E. Michel, and L. P. Steele. 1999. “A 1000-Year High Precision Record of δ13C in Atmospheric CO2.” Tellus B: Chemical & Physical Meteorology 51 (2): 170–193. https://doi.org/10.3402/tellusb.v51i2.16269.
- Fujimoto, K., K. Ono, S. Watanabe, S. Taniguchi, T. Inoue, K. Kanayama, and T. Ogawa. 2021. “Estimation of Probable Annual Fine-Root Production and Missing Dead Roots Associated with the Ingrowth Core Method: Attempt with Major Mangrove Species on Iriomote Island, Southwestern Japan, Located in the Subtropics.” Mangrove Science 12:11–24. http://www.mangrove.or.jp/gakkai/subpage/gakkaishi/vol_12.html.
- Golchin, A., J. Oades, J. Skjemstad, and P. Clarke. 1994. “Soil structure and carbon cycling.” Soil Research 32 (5): 1043. https://doi.org/10.1071/SR9941043.
- Hashimoto, Y., and M. Hyakumachi. 1998. “Effects of Vegetation Change and Soil Disturbance on Ectomycorrhizas of Betula Platyphylla Var. Japonica: A Test Using Seedlings Planted in Soils Taken from Various Sites.” Mycoscience 39 (4): 433–439. https://doi.org/10.1007/BF02460904.
- Heckman, K., C. E. Hicks Pries, C. R. Lawrence, C. Rasmussen, S. E. Crow, A. M. Hoyt, S. F. von Fromm, et al. 2022. “Beyond Bulk: Density Fractions Explain Heterogeneity in Global Soil Carbon Abundance and Persistence.” Global Change Biology 28 (3): 1178–1196. https://doi.org/10.1111/gcb.16023.
- Huxham, M., J. Langat, F. Tamooh, H. Kennedy, M. Mencuccini, M. W. Skov, and J. Kairo. 2010. “Decomposition of Mangrove Roots: Effects of Location, Nutrients, Species Identity and Mix in a Kenyan Forest.” Estuarine, Coastal and Shelf Science 88 (1): 135–142. https://doi.org/10.1016/j.ecss.2010.03.021.
- Iimura, Y., K. Kinjo, M. Kondo, and T. Ohtsuka. 2019. “Soil Carbon Stocks and Their Primary Origin at Mature Mangrove Ecosystems in the Estuary of Fukido River, Ishigaki Island, Southwestern Japan.” Soil Science & Plant Nutrition 65 (5): 435–443. https://doi.org/10.1080/00380768.2019.1660589.
- Jia, B., Z. Niu, Y. Wu, Y. Kuzyakov, and X. G. Li. 2020. “Waterlogging Increases Organic Carbon Decomposition in Grassland Soils.” Soil Biology & Biochemistry 148:107927. https://doi.org/10.1016/j.soilbio.2020.107927.
- Kaiser, K., G. Guggenberger, and W. Zech. 2001. “Isotopic Fractionation of Dissolved Organic Carbon in Shallow Forest Soils as Affected by Sorption.” European Journal of Soil Science 52: 585–597. https://doi.org/10.1046/j.1365-2389.2001.00407.x.
- Keeling, R. F., H. D. Graven, L. R. Welp, L. Resplandy, J. Bi, S. C. Piper, Y. Sun, A. Bollenbacher, and H. A. J. Meijer. 2017. “Atmospheric Evidence for a Global Secular Increase in Carbon Isotopic Discrimination of Land Photosynthesis.” Proceedings of the National Academy of Sciences 114 (39): 10361–10366. https://doi.org/10.1073/pnas.1619240114.
- Keuskamp, J. A., H. Schmitt, H. J. Laanbroek, J. T. A. Verhoeven, and M. M. Hefting. 2013. “Nutrient Amendment Does Not Increase Mineralisation of Sequestered Carbon During Incubation of a Nitrogen Limited Mangrove Soil.” Soil Biology & Biochemistry 57:822–829. https://doi.org/10.1016/j.soilbio.2012.08.007.
- Kida, M., and N. Fujitake. 2020. “Organic carbon stabilization mechanisms in mangrove soils: A review.” Forests 11 (9): 981. https://doi.org/10.3390/f11090981.
- Kida, M., M. Kondo, M. Tomotsune, K. Kinjo, T. Ohtsuka, and N. Fujitake. 2019. “Molecular Composition and Decomposition Stages of Organic Matter in a Mangrove Mineral Soil with Time.” Estuarine, Coastal and Shelf Science 231:106478. https://doi.org/10.1016/j.ecss.2019.106478.
- Kida, M., M. Tanabe, M. Tomotsune, S. Yoshitake, K. Kinjo, T. Ohtsuka, and N. Fujitake. 2019. “Changes in Dissolved Organic Matter Composition and Dynamics in a Subtropical Mangrove River Driven by Rainfall.” Estuarine, Coastal and Shelf Science 223:6–17. https://doi.org/10.1016/j.ecss.2019.04.029.
- Kida, M., M. Tomotsune, Y. Iimura, K. Kinjo, T. Ohtsuka, and N. Fujitake. 2017. “High Salinity Leads to Accumulation of Soil Organic Carbon in Mangrove Soil.” Chemosphere 177:51–55. https://doi.org/10.1016/j.chemosphere.2017.02.074.
- Kida, M., I. Watanabe, K. Kinjo, M. Kondo, S. Yoshitake, M. Tomotsune, Y. Iimura, et al. 2021. “Organic Carbon Stock and Composition in 3.5-M Core Mangrove Soils (Trat, Thailand).” Science of the Total Environment 801:149682. https://doi.org/10.1016/j.scitotenv.2021.149682.
- Kinjo, K., Y. Tokashiki, K. Sato, M. Kitou, and M. Shimo. 2005. “Characteristics of Surface Sediments Along a Creek in a Mangrove Forest.” Soil Science & Plant Nutrition 51 (6): 809–817. https://doi.org/10.1111/j.1747-0765.2005.tb00115.x.
- Kölbl, A., and I. Kögel‐Knabner. 2004. “Content and Composition of Free and Occluded Particulate Organic Matter in a Differently Textured Arable Cambisol as Revealed by Solid‐State 13 C NMR Spectroscopy.” Journal of Plant Nutrition and Soil Science 167 (1): 45–53. https://doi.org/10.1002/jpln.200321185.
- Kooner, Z. S., P. M. Jardine, and S. Feldman. 1995. “Competitive Surface Complexation Reactions of Sulfate and Natural Organic Carbon on Soil.” Journal of Environmental Quality 24 (4): 656–662. https://doi.org/10.2134/jeq1995.00472425002400040016x.
- Kristensen, E., S. Bouillon, T. Dittmar, and C. Marchand. 2008. “Organic Carbon Dynamics in Mangrove Ecosystems: A Review.” Aquatic Botany 89 (2): 201–219. https://doi.org/10.1016/j.aquabot.2007.12.005.
- Lalonde, K., A. Mucci, A. Ouellet, and Y. Gélinas. 2012. “Preservation of Organic Matter in Sediments Promoted by Iron.” Nature 483 (7388): 198–200. https://doi.org/10.1038/nature10855.
- Lee, C. 1992. “Controls on Organic Carbon Preservation: The Use of Stratified Water Bodies to Compare Intrinsic Rates of Decomposition in Oxic and Anoxic Systems.” Geochimica et cosmochimica acta 56 (8): 3323–3335. https://doi.org/10.1016/0016-7037(92)90308-6.
- Liao, J. D., T. W. Boutton, and J. D. Jastrow. 2006. “Organic Matter Turnover in Soil Physical Fractions Following Woody Plant Invasion of Grassland: Evidence from Natural 13C and 15N.” Soil Biology & Biochemistry 38 (11): 3197–3210. https://doi.org/10.1016/j.soilbio.2006.04.004.
- Liu, X., Y. Xiong, and B. Liao. 2017. “Relative Contributions of Leaf Litter and Fine Roots to Soil Organic Matter Accumulation in Mangrove Forests.” Plant and Soil 421 (1–2): 493–503. https://doi.org/10.1007/s11104-017-3477-5.
- Lorenz, M., D. Derrien, B. Zeller, T. Udelhoven, W. Werner, and S. Thiele-Bruhn. 2020. “The Linkage of 13C and 15N Soil Depth Gradients with C: N and O: C Stoichiometry Reveals Tree Species Effects on Organic Matter Turnover in Soil.” Biogeochemistry 151 (2–3): 203–220. https://doi.org/10.1007/s10533-020-00721-3.
- Lovelock, C. E., I. C. Feller, R. Reef, and R. W. Ruess. 2014. “Variable effects of nutrient enrichment on soil respiration in mangrove forests.” Plant and Soil 379 (1–2): 135–148. https://doi.org/10.1007/s11104-014-2036-6.
- Luo, X., E. Hou, L. Zhang, and D. Wen. 2020. “Soil Carbon Dynamics in Different Types of Subtropical Forests as Determined by Density Fractionation and Stable Isotope Analysis.” Forest Ecology and Management 475:118401. https://doi.org/10.1016/j.foreco.2020.118401.
- Lützow, M. V., I. Kögel-Knabner, K. Ekschmitt, E. Matzner, G. Guggenberger, B. Marschner, and H. Flessa. 2006. “Stabilization of Organic Matter in Temperate Soils: Mechanisms and Their Relevance Under Different Soil Conditions – a Review.” European Journal of Soil Science 57 (4): 426–445. https://doi.org/10.1111/j.1365-2389.2006.00809.x.
- Maher, D. T., I. R. Santos, L. Golsby-Smith, J. Gleeson, and B. D. Eyre. 2013. “Groundwater-Derived Dissolved Inorganic and Organic Carbon Exports from a Mangrove Tidal Creek: The Missing Mangrove Carbon Sink?” Limnology & Oceanography 58 (2): 475–488. https://doi.org/10.4319/lo.2013.58.2.0475.
- Marchand, C., J. R. Disnar, E. Lallier-Vergès, and N. Lottier. 2005. “Early Diagenesis of Carbohydrates and Lignin in Mangrove Sediments Subject to Variable Redox Conditions (French Guiana).” Geochimica et cosmochimica acta 69 (1): 131–142. https://doi.org/10.1016/j.gca.2004.06.016.
- Marschner, B., S. Brodowski, A. Dreves, G. Gleixner, A. Gude, P. M. Grootes, U. Hamer, et al. 2008. “How Relevant is Recalcitrance for the Stabilization of Organic Matter in Soils?” Journal of Plant Nutrition and Soil Science 171 (1): 91–110. https://doi.org/10.1002/jpln.200700049.
- McKee, K. L., D. R. Cahoon, and I. C. Feller. 2007. “Caribbean Mangroves Adjust to Rising Sea Level Through Biotic Controls on Change in Soil Elevation.” Global Ecology and Biogeography 16 (5): 545–556. https://doi.org/10.1111/j.1466-8238.2007.00317.x.
- McLeod, E., G. L. Chmura, S. Bouillon, R. Salm, M. Björk, C. M. Duarte, C. E. Lovelock, W. H. Schlesinger, and B. R. Silliman. 2011. “A Blueprint for Blue Carbon: Toward an Improved Understanding of the Role of Vegetated Coastal Habitats in Sequestering CO2.” Frontiers in Ecology and the Environment 9 (10): 552–560. https://doi.org/10.1890/110004.
- Middleton, B. A., and K. L. McKee. 2001. “Degradation of Mangrove Tissues and Implications for Peat Formation in Belizean Island Forests.” Journal of Ecology 89 (5): 818–828. https://doi.org/10.1046/j.0022-0477.2001.00602.x.
- Miyajima, T., M. Hori, M. Hamaguchi, H. Shimabukuro, and G. Yoshida. 2017. “Geophysical Constraints for Organic Carbon Sequestration Capacity of Zostera Marina Seagrass Meadows and Surrounding Habitats.” Limnology & Oceanography 62 (3): 954–972. https://doi.org/10.1002/lno.10478.
- Muhammad-Nor, S. M., M. Huxham, Y. Salmon, S. J. Duddy, A. Mazars-Simon, M. Mencuccini, P. Meir, and G. Jackson. 2019. “Exceptionally High Mangrove Root Production Rates in the Kelantan Delta, Malaysia; an Experimental and Comparative Study.” Forest Ecology and Management 444:214–224. https://doi.org/10.1016/j.foreco.2019.04.026.
- Ohtsuka, T., T. Onishi, S. Yoshitake, M. Tomotsune, M. Kida, Y. Iimura, M. Kondo, et al. 2020. “Lateral Export of Dissolved Inorganic and Organic Carbon from a Small Mangrove Estuary with Tidal Fluctuation.” Forests 11 (10): 1041. https://doi.org/10.3390/f11101041.
- Ohtsuka, T., M. Tomotsune, V. Suchewaboripont, Y. Iimura, M. Kida, S. Yoshitake, M. Kondo, and K. Kinjo. 2019. “Stand Dynamics and Aboveground Net Primary Productivity of a Mature Subtropical Mangrove Forest on Ishigaki Island, South-Western Japan.” Regional Studies in Marine Science 27:100516. https://doi.org/10.1016/j.rsma.2019.100516.
- Ono, K., K. Fujimoto, Y. Hirata, R. Tabuchi, S. Taniguchi, K. Furukawa, S. Watanabe, R. Suwa, and S. Lihpai. 2022. “Estimation of Total Fine Root Production Using Continuous Inflow Methods in Tropical Mangrove Forest on Pohnpei Island, Micronesia: Fine Root Necromass Accumulation is a Substantial Contributor to Blue Carbon Stocks.” Ecological Research 37 (1): 33–52. https://doi.org/10.1111/1440-1703.12280.
- Ono, K., S. Hiradate, S. Morita, M. Hiraide, Y. Hirata, K. Fujimoto, R. Tabuchi, and S. Lihpai. 2015. “Assessing the Carbon Compositions and Sources of Mangrove Peat in a Tropical Mangrove Forest on Pohnpei Island, Federated States of Micronesia.” Geoderma 245–246:11–20. https://doi.org/10.1016/j.geoderma.2015.01.008.
- Parker, J. L., I. J. Fernandez, L. E. Rustad, and S. A. Norton. 2002. “Soil Organic Matter Fractions in Experimental Forested Watersheds.” Water, Air, and Soil Pollution 138:101–121. https://doi.org/10.1023/A:1015516607941.
- Paul, A., J. Balesdent, and C. Hatté. 2020. “13C-14C Relations Reveal That Soil 13C-Depth Gradient is Linked to Historical Changes in Vegetation 13C.” Plant and Soil 447 (1–2): 305–317. https://doi.org/10.1007/s11104-019-04384-4.
- Richards, D. R., and D. A. Friess. 2016. “Rates and Drivers of Mangrove Deforestation in Southeast Asia, 2000–2012.” Proceedings of the National Academy of Sciences 113:344–349. https://doi.org/10.1073/pnas.1510272113.
- Riedel, T., D. Zak, H. Biester, and T. Dittmar. 2013. “Iron Traps Terrestrially Derived Dissolved Organic Matter at Redox Interfaces.” Proceedings of the National Academy of Sciences 110 (25): 10101–10105. https://doi.org/10.1073/pnas.1221487110.
- Romero, L. M., T. J. Smith, and J. W. Fourqurean. 2005. “Changes in Mass and Nutrient Content of Wood During Decomposition in a South Florida Mangrove Forest.” Journal of Ecology 93 (3): 618–631. https://doi.org/10.1111/j.1365-2745.2005.00970.x.
- Santín, C., M. González-Pérez, X. L. Otero, P. Vidal-Torrado, F. Macías, and M. Á. Álvarez. 2008. “Characterization of Humic Substances in Salt Marsh Soils Under Sea Rush (Juncus Maritimus).” Estuarine, Coastal and Shelf Science 79 (3): 541–548. https://doi.org/10.1016/j.ecss.2008.05.007.
- Saraswati, S., C. Dunn, W. J. Mitsch, and C. Freeman. 2016. “Is Peat Accumulation in Mangrove Swamps Influenced by the “Enzymic latch” Mechanism?” Wetlands Ecology and Management 24 (6): 641–650. https://doi.org/10.1007/s11273-016-9493-z.
- Shields, M. R., T. S. Bianchi, Y. Gélinas, M. A. Allison, and R. R. Twilley. 2016. “Enhanced Terrestrial Carbon Preservation Promoted by Reactive Iron in Deltaic Sediments.” Geophysical Research Letters 43 (3): 1149–1157. https://doi.org/10.1002/2015GL067388.
- Sholkovitz, E. R. 1976. “Flocculation of Dissolved Organic and Inorganic Matter During the Mixing of River Water and Seawater.” Geochimica et cosmochimica acta 40 (7): 831–845. https://doi.org/10.1016/0016-7037(76)90035-1.
- Six, J. 1999. “Recycling of Sodium Polytungstate Used in Soil Organic Matter Studies.” Soil Biology & Biochemistry 31 (8): 1193–1196. https://doi.org/10.1016/S0038-0717(99)00023-1.
- Sollins, P., P. Homann, and B. A. Caldwell. 1996. “Stabilization and Destabilization of Soil Organic Matter: Mechanisms and Controls.” Geoderma 74 (1–2): 65–105. https://doi.org/10.1016/S0016-7061(96)00036-5.
- Sollins, P., M. G. Kramer, C. Swanston, K. Lajtha, T. Filley, A. K. Aufdenkampe, R. Wagai, and R. D. Bowden. 2009. “Sequential Density Fractionation Across Soils of Contrasting Mineralogy: Evidence for Both Microbial- and Mineral-Controlled Soil Organic Matter Stabilization.” Biogeochemistry 96 (1–3): 209–231. https://doi.org/10.1007/s10533-009-9359-z.
- Stuiver, M., and H. A. Polach. 1977. “Discussion Reporting of 14 C Data.” Radiocarbon 19 (3): 355–363. https://doi.org/10.1017/S0033822200003672.
- Swanston, C. W., M. S. Torn, P. J. Hanson, J. R. Southon, C. T. Garten, E. M. Hanlon, and L. Ganio. 2005. “Initial Characterization of Processes of Soil Carbon Stabilization Using Forest Stand-Level Radiocarbon Enrichment.” Geoderma 128 (1–2): 52–62. https://doi.org/10.1016/j.geoderma.2004.12.015.
- Tabuchi, R. 1983. “Fine Root Amount of Mangrove Forest: A Preliminary Survey.” Indian Journal of Plant Sciences 1:31–40.
- Tan, Z., R. Lal, L. Owens, and R. Izaurraralde. 2007. “Distribution of Light and Heavy Fractions of Soil Organic Carbon as Related to Land Use and Tillage Practice.” Soil and Tillage Research 92 (1–2): 53–59. https://doi.org/10.1016/j.still.2006.01.003.
- Torn, M. S., A. G. Lapenis, A. Timofeev, M. L. Fischer, B. V. Babikov, and J. W. Harden. 2002. “Organic Carbon and Carbon Isotopes in Modern and 100-Year-Old-Soil Archives of the Russian Steppe.” Global Change Biology 8 (10): 941–953. https://doi.org/10.1046/j.1365-2486.2002.00477.x.
- Wagai, R., L. M. Mayer, and K. Kitayama. 2009. “Nature of the “Occluded” Low-Density Fraction in Soil Organic Matter Studies: A Critical Review.” Soil Science & Plant Nutrition 55 (1): 13–25. https://doi.org/10.1111/j.1747-0765.2008.00356.x.
- Wagai, R., L. M. Mayer, K. Kitayama, and H. Knicker. 2008. “Climate and Parent Material Controls on Organic Matter Storage in Surface Soils: A Three-Pool, Density-Separation Approach.” Geoderma 147 (1–2): 23–33. https://doi.org/10.1016/j.geoderma.2008.07.010.
- Werth, M., and Y. Kuzyakov. 2010. “13C Fractionation at the Root–Microorganisms–Soil Interface: A Review and Outlook for Partitioning Studies.” Soil Biology & Biochemistry 42 (9): 1372–1384. https://doi.org/10.1016/j.soilbio.2010.04.009.
- Yarwood, S. A. 2018. “The Role of Wetland Microorganisms in Plant-Litter Decomposition and Soil Organic Matter Formation: A Critical Review.” FEMS Microbiology Ecology 94 (11). https://doi.org/10.1093/femsec/fiy175.
- Yokoyama, Y., Y. Miyairi, T. Aze, C. Sawada, Y. Ando, S. Izawa, Y. Ueno, et al. 2022. “Efficient Radiocarbon Measurements on Marine and Terrestrial Samples with Single Stage Accelerator Mass Spectrometry at the Atmosphere and Ocean Research Institute, University of Tokyo.” Nuclear Instruments & Methods in Physics Research Section B 532:62–67. https://doi.org/10.1016/j.nimb.2022.10.006.
- Yokoyama, Y., Y. Miyairi, T. Aze, M. Yamane, C. Sawada, Y. Ando, M. de Natris, et al. 2019. “A Single Stage Accelerator Mass Spectrometry at the Atmosphere and Ocean Research Institute, the University of Tokyo.” Nuclear Instruments & Methods in Physics Research Section B 455:311–316. https://doi.org/10.1016/j.nimb.2019.01.055.
- Zeh, L., M. T. Igel, J. Schellekens, J. Limpens, L. Bragazza, and K. Kalbitz. 2020. “Vascular Plants Affect Properties and Decomposition of Moss-Dominated Peat, Particularly at Elevated Temperatures.” Biogeosciences 17 (19): 4797–4813. https://doi.org/10.5194/bg-17-4797-2020.
- Zhang, Y., J. Du, X. Ding, and F. Zhang. 2016. “Comparison Study of Sedimentary Humic Substances Isolated from Contrasting Coastal Marine Environments by Chemical and Spectroscopic Analysis.” Environmental Earth Sciences 75 (5): 378. https://doi.org/10.1007/s12665-016-5263-8.
- Zhao, B., P. Yao, T. S. Bianchi, M. R. Shields, X. Q. Cui, X. W. Zhang, X. Y. Huang, C. Schröeder, J. Zhao, and Z. G. Yu. 2018. “The Role of Reactive Iron in the Preservation of Terrestrial Organic Carbon in Estuarine Sediments.” Journal of Geophysical Research: Biogeosciences 123 (12): 3556–3569. https://doi.org/10.1029/2018JG004649.