ABSTRACT
As the redox catalytic activity of iron may be detrimental for waterlogged archaeological wood, it is of importance to know what ligands bind the iron, their distribution in the wood, and what the reactivity of these compounds are. We have identified iron-tannin precipitates in archaeological oak timber from the shipwreck of the seventeenth-century warship The Sword with confocal Raman spectroscopy in combination with light- and scanning electron microscopy. Iron tannin precipitates are present as larger aggregates (10–50 µm) in the lumen and vessels, but also as contamination within the wood cell wall. As the stability constants of iron-tannin and iron-diethylenetriamine-pentaacetic acid (DTPA) – a chelating agent commonly employed by conservators to extract iron – are in the same range, extraction experiments were carried out. These demonstrate that iron-tannin precipitations are difficult to extract. Tannin chemistry in waterlogged oak needs to be further explored to understand the mechanism of iron accumulation in wood as well as to correctly treat and preserve cultural heritage.
Introduction
During the last three decades, the problem of iron accumulation in waterlogged archaeological wood and its potential for accelerating abiotic wood degradation by catalytic oxidation have been discussed. The accumulation of iron has been reported in both terrestrial and marine environments and values over 1 wt% are not uncommon, even though such high values appear to require the presence of nearby corroding iron objects (MacLeod and Richards Citation1996; Wetherall et al. Citation2008; Fors et al. Citation2012; Welling, Schwarz, and Bauch Citation2018). However, even without iron objects present, the accumulation is substantial: an increase by a factor of c.200 (0.2 wt%) and 10 (0.03 wt%) was found when oak was submerged at different sites in the Baltic sea for two and five years respectively (Fojutowski et al. Citation2014; Komorowicz et al. Citation2018).
In the Vasa shipwreck, some correlation between abiotic holocellulose degradation and iron content was found (Bjurhager et al. Citation2012). Likewise, model studies on fresh oak have shown that the presence of Fe2+ facilitates oxidation and reduces the mechanical state of the wood as well as depolymerises holocellulose (Norbakhsh, Bjurhager, and Almkvist Citation2013, Citation2014). Iron likely enables degradation in archaeological wood by generating oxidative radicals that attack the wooden components directly (Almkvist and Persson Citation2008; Lindfors, Lindström, and Iversen Citation2008) as well as by producing an acidic environment through the oxidation of sulphur compounds present in waterlogged wood (Fors Citation2008).
The mechanism of accumulation of iron in buried or submerged wood is not completely understood. X-ray absorption near-edge structure (XANES) studies of iron speciation in archaeological wood found that Fe3+ was prevalent in the surface of the wood, whereas a mixture of Fe2+ and Fe3+ was present below the surface (Wetherall et al. Citation2008; Almkvist and Persson Citation2011). Almkvist and Persson (Citation2011) proposed the hypothesis that the more mobile Fe2+ ion diffuses into the wood and that oxidation occurs at a later stage – e.g. upon excavation. This conclusion was also given in a study on subfossil oak with Mössbauer spectroscopy: Fe2+ present in the sample material quickly oxidised upon contact with the atmosphere (van Bürck, Wagner, and Lerf Citation2012). Almkvist and Persson (Citation2011) also found that fresh oak impregnated with FeCl2 had a preferential absorption of iron compared to chloride, which would indicate that iron has been bound by organic ligands in the wood.
To better understand the accumulation and activity of iron in archaeological wood, its speciation and distribution must be further studied. The speciation – in terms of oxidation state and molecular/complex structure – will to a large degree determine its reactivity and its distribution in the wood matrix – due to the short lifetime of radicals – and determine what constituents are oxidised.
Many studies have focused on the molecular structure of inorganic iron in waterlogged archaeological wood (Sandström et al. Citation2002; Wetherall et al. Citation2008; Rémazeilles et al. Citation2019), but less attention has been given to the organic ligands. Both fresh and deteriorated wood contain a number of possible iron chelators: oxalic acid, low molecular lignins, polyphenols etc. Many of these compounds are present at high concentrations and form stable complexes with iron, which suggests that they could play an important role in iron accumulation in wood as well as influence the reactivity of the accumulated iron. To our knowledge, no attempts at quantifying the different iron containing compounds in waterlogged archaeological wood have been conducted, but given the presence of strong iron chelators, it appears unlikely that only inorganic species would prevail.
One such group of powerful iron chelators, that has the potential to aid in both the accumulation and redox activity of iron, is the tannins. Tannins are branched polyphenols of gallic acid, ellagic acid, or flavonoids (see ) and are present in most plants, but with an especially high abundance in oak wood (Scalbert, Monties, and Favre Citation1988). Ellagitannins are the main constituent of oak wood tannins (Du Penhoat et al. Citation1991). As flavonoid tannins are a very minor part of oak wood, they are not further mentioned. The formation of a black–blue complex on mixing tannins with iron is ancient technology and one of the oldest chemical reactions recorded (Lillie Citation1972). The presence of iron-tannin complexes in subfossil oak is well known (Krutul and Kocon Citation1982; Kalicki and Krąpiec Citation1995; van Bürck, Wagner, and Lerf Citation2012) and the presence of similar complexes in archaeological wood, especially in tannin-rich oak, can be assumed. For instance, precipitated dark polyphenols were recently detected with UV micro spectrophotometry (UMSP) in both archaeological (Koch et al. Citation2018) and subfossil oak (Baar et al. Citation2019), which were presumed to be iron-tannin precipitates.
Figure 1. The molecular structure of gallica acid, ellagic acid, tannic acid, and castalagin. Tannic acid is a gallotannin, a tannin that has a central carbohydrate partly or fully esterified by gallic acid, whereas castalagin is an ellagitannin with ellagic acid ester bonded to the central carbohydrate.
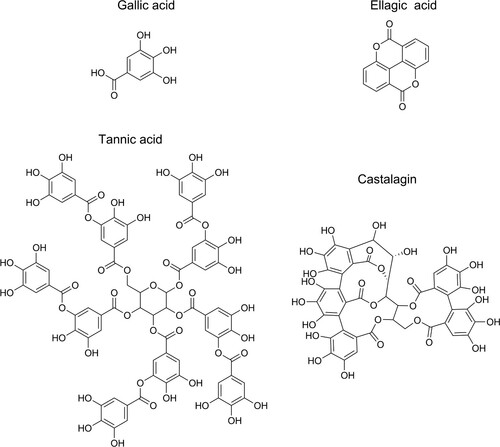
Tannins, and smaller polyphenols, such as gallic acid, can react with iron in a number of ways: complex formation, precipitation, facilitation of autoxidation of Fe2+ to Fe3+, and act as a reducing agent for Fe3+ (see ) (Perron and Brumaghim Citation2009). Thus, in iron-containing systems, polyphenols can act as either an antioxidant or a pro-oxidant, depending on the environment (Pulido, Bravo, and Saura-Calixto Citation2000; Chvátalová et al. Citation2008; Nkhili et al. Citation2014). A better understanding of the iron-tannin chemistry in waterlogged archaeological oak is therefore needed to correctly preserve and treat cultural heritage.
Figure 2. Mechanisms of polyphenolic reactions with iron (with pyrogallol as model compound) adapted from Perron and Brumaghim (Citation2009). Autooxidation (A) by complex formation and subsequent electron transfer in the presence of oxygen leading to a Fe(III)-polyphenol complex. Pro-oxidant reaction (B) by complex formation, iron reduction and semiquinone formation followed by a second iron reduction and formation of a quinone species.
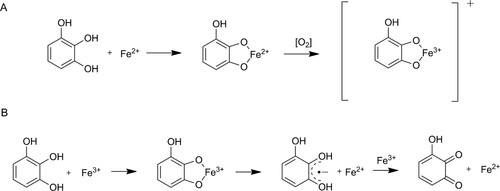
Here, we use confocal Raman spectroscopy to identify iron-tannin precipitates and study their micro-distribution in archaeological waterlogged oak (Quercus sp.). The study is divided into a series of investigations on both synthesised compounds and on thin wood sections. In the first step we synthesise iron-tannin precipitates from commercial products and from oak extracts. Secondly, we examine the wood structure by light microscopy and localise and analyse the precipitations with confocal Raman spectroscopy. These are compared to the synthesised compounds. Finally, as the stability constants of iron-tannin and iron-diethylenetriamine-pentaacetic acid (DTPA) – a chelating agent typically employed by conservators to extract iron – are in the same range (Ahrland, Dahlgren, and Persson Citation1990; Perron and Brumaghim Citation2009), extraction with DPTA and its effect on removal of iron-tannins are investigated.
Materials and methods
Archaeological and reference wood
The wood material used in this study came from the shipwreck The Sword (Swedish: Svärdet) that sank into the seabed in 1676 off the island of Gotland in the Baltic Sea. The wreck, the wreck site, and the timber have previously been described in connection with investigations on its state of microbial degradation and the content of iron and sulphur (Fors et al. Citation2015; Björdal and Fors Citation2019). From the same timber, close to samples investigated earlier, one sample with intact original surfaces was available for our analysis.
This waterlogged oak sample (Quercus sp), representing heartwood, had been kept wet by storage in air-free plastic bags at +8 C° during the last two years prior to examination. Exposure to the atmosphere did occasionally occur. From the first centimetre of the crosscut end, one subsample of approximately 15 × 15 × 10 mm3 was cut out by knife for further investigations. Subsequently, thin transverse and longitudinal sections from the surface layer were taken by hand with a double-edged razor blade. The parallel sections were subsequently prepared for light microscopy, confocal Raman spectroscopy, and DTPA extraction studies. For scanning electron microscopy (SEM) with energy-dispersive X-ray spectroscopy (EDX), cubes of approximately 4 × 3 × 2 mm3 were prepared. Recent non-contaminated dry heartwood oak (Swedish origin) was used as a reference in SEM-EDX analysis as well as for extraction of tannins.
Synthesis of iron-tannin precipitates
In order to correctly identify iron-tannin complexes in the sample material using confocal Raman spectroscopy, iron-tannins were synthesised from tannic acid (Sigma Aldrich) and from extracts of both recent and archeological oak that was synthesised.
Tannic acid and iron(II)sulphate (FeSO4·7H2O, reagent quality, Honeywell) were each dissolved in deionised water to a molarity of 20 mM. The two solutions were then mixed and left to react for a week with occasional stirring. The precipitate was collected and washed repeatedly with deionised water.
Both recent and archaeological oak wood were cut into shavings with a knife. One gram (1 g) was extracted in deionised water (5 ml) and stirred for 48 h. Afterwards, the two yellow coloured solutions were mixed with a 20 mM solution of iron (II) sulphate and left to react for a week with occasional stirring. The precipitates were collected and washed repeatedly with deionised water.
Raman analysis of iron-tannin precipitates
To locate and identify iron-tannin complexes in the wood, thin sections were transferred to a CaF2 microscopy slide (Raman grade, Crystran Ltd) equipped with small-lidded wells (ibidi GbmH). Here the sections were kept moist during analyses. The advantage of this set up is that the morphology and distribution of the compounds can be studied in situ.
A Raman spectrometer (Dilor Labram IV) coupled with an inverted confocal microscope (Olympus IX70) was used for analysis. The system detector was a charged coupled device (CCD) and a 632.8 nm (He/Ne) laser was used as excitation source. The acquisitions were triplicates of 30 s that were averaged into one observation. Microscope objectives of 10x and 100x were used, with a theoretical focal spot of 5 and 1 µm respectively. The laser intensity at the sample was c.2 mW. The spectrum resolution was 2.2 cm−1 and the system was continuously frequency-calibrated against a silica standard.
Spectra were acquired on loose particles, filled lumens, and vessels and on discoloured rays and cell walls. Most spectra were acquired with the 10x objective, but when small features (<20 µm) were to be analysed or when the photoluminescence level was high, the 100x objective was used. The imaging capacity of the microscope connected to the Raman instrument was not fully satisfactory, and thus complementing images on the same sections were acquired using another microscope. Literature sources and synthesised reference compounds (see above) were used to correctly identify iron-tannin complexes.
Band fitting of synthesised compounds (the compounds in ) were performed in LabSpec 5 (Horiba Ltd) using a Levenberg-Marquardt algorithm for Lorentzian–Gaussian functions. Compounds in the sample material were individually compared to these bands.
Figure 3. Spatially offset normalised Raman spectra of iron-tannins precipitates synthesised from tannic acid (Fe-TA), extract from archaeological oak (Fe-AOE), and extract from recent oak (Fe-ROE) and of iron-tannin precipitates in The Sword. The band at 1594 cm−1 in the spectrum for The Sword is likely lignin.
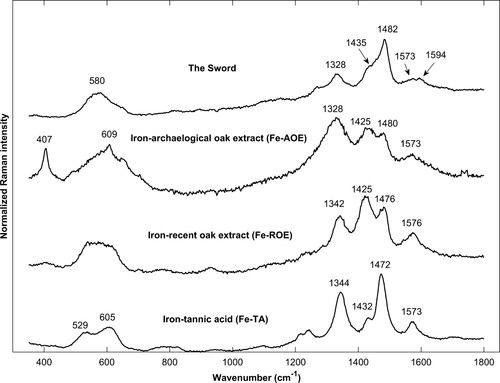
Elemental analysis by SEM-EDX
The wet small cubes were dried by critical point drying (Leica CPD300) to reduce shrinkage, and gold-sputtered (Agar Sputter Coater B7340) to enhance conductivity. The samples were analysed with a FEI Quanta200 equipped with Oxford Inca X-max 80. The instrument was operated at high vacuum, 20 kV voltage, 11 mm working distance and with both secondary and backscatter electron imaging. Areas of interest were localised and elemental maps and particle compositions were acquired.
Extraction experiment
To investigate whether iron-tannin precipitates were extracted by DTPA, micro sections were treated with the chelating agent. Ten transversal and six longitudinal micro sections were cut in succession. Half of them were extracted in vials containing 2 ml of 10 mM DTPA buffered to pH 7 with HCl and the other half were stored in vials of 2 ml of distilled water. The extraction treatment was carried out for 21 days. Following extraction, the micro sections were analysed in the Raman set up. Another set of 12 transversal sections were also studied by light microscopy where the same site was photographed before and after extraction – six in DTPA and six in water – to observe any visual changes, such as change in colour or removal of particles.
Results and discussion
Synthesis of iron-tannin precipitates
In order to correctly identify iron-tannin precipitates in archaeological wood, iron-tannins were synthesised from extracts originating from recent oak wood, archeological oak, and from commercial tannic acid (see methods above). Tannic acid (decagalloyl glucose) differentiates from most tannins in oak as it is made up by gallic acid (gallotannin), whereas most tannins in oak consist of ellagic acid (ellagitannins) (Scalbert, Monties, and Favre Citation1988). As there are many Raman studies on iron-gallotannin complexes these were included as a reference (Lee, Mahon, and Creagh Citation2006; Ponce et al. Citation2016; Díaz Hidalgo et al. Citation2018).
The polyphenol content of fresh oak wood consists mainly of the ellagitannins of vescalagin and castalagin (stereoisomers with the same formula) and roburins (Du Penhoat et al. Citation1991). These tannins make up as much as about 1 and 10% of the dry weight of sapwood and heartwood respectively (Scalbert, Monties, and Favre Citation1988). A recent study on subfossil oak found that vescalagin and castalagin were reduced by a factor of 5–15 and gallic and ellagic acid by a factor 1–5 as compared to recent oak (Baar et al. Citation2019). The ellagitannin content of waterlogged archaeological oak has also been analysed and was found to be substantially lower than recent oak (Dedic et al. Citation2014).
Based on the examples mentioned above and previous studies which found that ellagitannins are quite readily extracted from oak with water at room temperature (Moutounet et al. Citation1989; Jordão, Ricardo-da-Silva, and Laureano Citation2005),the tannin content of the extracts are expected to be in the 0.1–10 mM range, with the archaeological and recent wood extracts at the lower and higher end respectively. The main iron binding constituents are likely vescalagin, castalagin, roburins, and gallic acid with the polyfunctional tannins dominating in the extract from recent oak.
All three reaction mixtures produced a black precipitate. For the reaction mixture containing tannic acid, the precipitation was black–blue and aggregated into larger (>100 µm) particles, henceforth termed Fe-TA. The precipitates synthesised from extracts were off-black with a warmer hue and formed smaller particles (<100 µm). The total amount of precipitates was also less, especially for the extracts from archaeological wood, obviously due to the smaller amount of tannins in the extracts. The precipitates of recent oak extract are henceforth termed Fe-ROE whereas precipitates of archaeological oak extracts are termed Fe-AOE.
Raman analysis of iron-tannin precipitates
Raman spectroscopic analysis of iron-tannin complexes have mainly been conducted in studies on iron gall ink (IGI). IGI is a historical ink produced by mixing iron salts with tannin-rich (typically gallotannin-rich) extracts from plants. Raman spectroscopy has been applied to classify both different compositions and state of degradation (Lee, Mahon, and Creagh Citation2006; Bicchieri et al. Citation2013; Piantanida et al. Citation2013) as well as to clarify its molecular structure (Ponce et al. Citation2016; Díaz Hidalgo et al. Citation2018). The publications generally show a great resemblance of Raman spectra acquired, but with some variations due to recipe and state of degradation. There is no consensus on the assignment of Raman bands of iron-gallic acid and IGI (Díaz Hidalgo et al. Citation2018) but the bands at 1584–1570 medium, 1494–68 strong, 1346–15 shoulder, and a broad feature at 600–500 appear in all studies and can be considered signature bands. A medium to weak band at 400 cm−1 has also been proposed as a signature band (Lee, Mahon, and Creagh Citation2006), but in studies of aged IGI this band is often obscured by noise or is missing.
The iron-tannic acid precipitate, Fe-TA, synthesised in this study has an overall resemblance with the literature cited above (see and ); however, many bands have a blue shift compared to IGI synthesised from tannic acid from the same supplier (Sigma Aldrich). These shifts are possibly due to the inconsistency of the tannic acid product – Díaz Hidalgo et al. (Citation2018) found that it contains polygalloyl glucose of different sizes as well as gallic acid – or the different synthesis conditions.
Table 1. Raman band positions (cm−1) for iron-tannin compounds.
The iron-tannins that was synthesised with extractives from recent and archaeological oak exhibited spectra that in many features are similar to Fe-TA and IGI from the literature: the signature bands were present as well as a few of the smaller features (1240, 937–933, and a broad band at 800 cm−1). However, the relative magnitude of 1425 and 1480–1476 cm−1 were interchanged, with the former being more intense. Similar patterns are observed for aging IGI (Bicchieri et al. Citation2013; Ponce et al. Citation2016) and are likely related to the ordering and the chemical integrity of the compounds. The two spectra also exhibit a lower signal to noise ratio (S/N) and fewer bands are present in the middle region (700–1200 cm−1) compared to Fe-TA. As aqueous extracts of oak, apart from tannins, also contain low-molecular carbohydrates (Ajuong and Redington Citation2004), the precipitations are likely less pure than Fe-TA.
Raman microanalysis of micro sections taken from The Sword showed that many dark precipitates exhibited a spectrum that resemble the iron-tannin precipitates described above. The signature bands are present at 1573, 1482, 1328, and 580 cm−1. The spectral profile, i.e. the relative magnitudes of the bands and their shape, are similar to Fe-TA and to IGI. However, less distinct spectra were also acquired (see ) where the spectral profile is more similar to Fe-AOE and Fe-ROE. As suggested above, this could be the result of differences in ordering, purity, and state of degradation.
Figure 4. Untreated Raman spectra of iron-tannin precipitates identified in different structures in The Sword: filled vessel (top), ray (middle), and cell wall (bottom) in cross section. Signature bands (580, 1328, 1482, and 1573 cm−1) are marked. See micrographs (Supplementary Figures 2–4) showing site of analysis. The top and bottom spectra represent extremes, whereas the middle was most commonly acquired. All spectra exhibit photoluminescence, likely from lignin and degraded wood material.
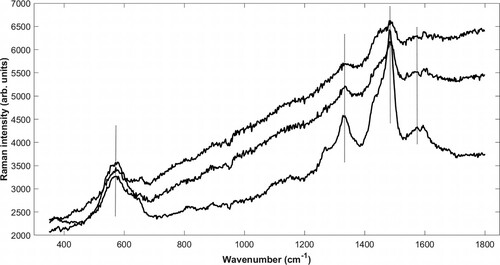
Bicchieri et al. (Citation2013) showed that the type of tannin involved in complexation will affect the position of most bands of the iron-tannin complex. This would indicate that the tannins involved in iron precipitating reactions in archaeological wood could be identified using Raman spectroscopy. However, as the tannins used for synthesis in this study were all mixtures, no such distinctions could be made.
SEM-EDX analysis of wood from The Sword
As a complement to the Raman investigation, SEM-EDX analysis was carried out on transversal and longitude sections. Elemental analysis found a background presence of iron throughout the samples. Similarly, sulphur, calcium, potassium, sodium, and zinc were also present at most sites. High iron content was found in many structural components: secondary cell wall, rays, vessels, and lumen filled with residual material and tyloses. Some iron containing particles (1–100 µm) were found, but more commonly the iron was dispersed into the cell wall structures where no particles were evident (). Due to the gold coating, only a semi-quantitative estimation of the elemental composition was possible. In some instances, iron and sulphur appeared to be present at similar concentrations, but more often iron was paired with only oxygen. Such sites could indicate iron oxides and hydroxides, but also the presence of organic ligands (-OH and -COOH).
Figure 5. SEM backscattered electron image (A) of a longitudinal section from The Sword showing fibres with partly fractured cell walls that opens up the fibres. EDX mapping of (B) iron and (C) sulphur at the same site. The cell wall has a high iron content but relatively little sulphur.
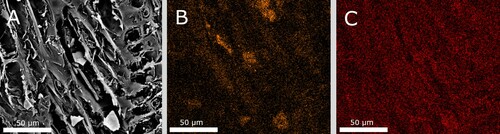
As samples for SEM and Raman analysis were cut with stainless-steel razor blades, a reference of recent oak was also analysed. The whole surface of the sample was mapped for iron and chromium (the main constituents of stainless steel). Only one occurrence of iron contamination was found: a 20 µm chipping of stainless steel (see Supplementary Figure 1). Apart from the chipping, no other iron particle or iron background was detected in the reference. Likewise, no chromium was detected in the archaeological material.
Distribution of iron-tannin precipitates in The Sword
To study the distribution of iron-tannin precipitates in the archaeological material, loose material/particles and dark/contaminated cell walls, rays, and vessels were analysed with the confocal Raman spectroscope. Spectra of varying quality were generated, as can be seen in , where signal-to-noise ratio and degree of photoluminescence vary. The top and bottom spectra represent the extremes, whereas the middle spectrum was the most commonly acquired. Compounds were only regarded as iron-tannins if they had the signature-bands mentioned in the previous section, with the exception of the band at 1573 cm−1, as it often was obscured in situ by the presence of lignin with its strong band at 1594–1598 cm−1 (Zeise et al. Citation2018). As the microscope connected to the Raman instrument provided images of poor quality (see Supplementary Figures 2–4), complementary micrographs of similar dark precipitates and contaminated wood structure from other sections were provided (see ). These do more clearly show the typical areas containing iron-tannin precipitates.
Figure 6. (A) micrograph (100x) of transverse section from The Sword. The wood tissue has turned dark and blackish. Both rays (red arrow), cell walls, and tyloses appears contaminated. In the lower left corner, a small vessel is filled with extensive amount of contaminated material (blue arrow); (B) micrograph (200x) of transverse section from The Sword, showing dark filled lumens of small vessels; and (C), micrograph (630x) of transverse section from The Sword, showing a dark filled vessel and dark contaminated rays.
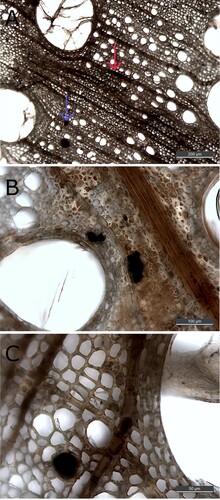
Iron-tannin precipitates were detected in filled lumen and vessels ((A, B)). In these voids, they appear to aggregate into larger particles (10–50 µm), but it could also be residual material interspersed with iron-tannins. Iron-tannin precipitates exhibit strong Raman scattering compared to holocellulose and lignin, which could mask the presence of these biopolymers. Thus, whether these particles are solely iron-tannins or if they also contain lignocellulosic compounds cannot be determined under our conditions. Iron tannin-precipitates were also detected in dark-coloured rays and cell walls ((A,B)). Here it appears as if precipitation has occurred within the existing structure. Not all dark precipitates and stained structures contained iron-tannins; consequently, iron-tannins cannot be identified solely visually.
The distribution of iron-tannin precipitates in The Sword is similar to what Koch et al. (Citation2018) and Baar et al. (Citation2019) found in subfossil and archaeological oak respectively. However, they did not find precipitated polyphenols in cell walls, which occurred several times in this study. The laser spot size (1 µm) and optics of the instrument did not allow for a further detailed study of the cell wall and middle lamella; thus, it could not be discerned if the compound was present in the lignin or cellulose rich part of the cell wall.
Our method cannot determine the absolute amount of iron-tannins, but as iron-tannin precipitates are present in all sections of the examined piece of wood, it appears likely that the polyphenols play an important role in the iron accumulation of waterlogged oak. When pine (Pinus sp.) and oak samples from the same marine site are analysed, oak appears to accumulate more iron (Fors et al. Citation2012; Björdal and Fors Citation2019). This could be a consequence of the much higher tannin content in oak wood compared to softwoods (Nisula Citation2018).
Three mechanisms of iron accumulation by reaction with tannins are plausible. First, chelation which forms large complexes that would be slow to diffuse out of the wood. Second, autooxidation of Fe2+, with what little oxygen is present, which would accumulate the less mobile Fe3+. Third, the complex precipitates and remains insoluble within the wood. In this study, precipitation had obviously occurred, but as our sample material was relatively acclimatised to the atmosphere, we cannot be certain that precipitation had not occurred already at the seabed. As Fe2+-tannins are unstable in the presence of oxygen, they are difficult to study. If, and under what conditions, they precipitate is unknown. Thus, to test the hypothesis of tannin-aided iron accumulation, iron-tannin reactions under seabed/burial conditions have to be conducted in the future.
Extraction experiment
A common method for extracting iron from archaeological wood is to use the chelating agent DTPA, due to its strong iron complexing abilities: over 80 wt% of the iron has been successfully extracted from waterlogged archaeological oak (Almkvist and Persson Citation2006). However, the Fe3+-gallic acid complex has stability constants in the same range as that of Fe3+-DTPA: logKFeLigand = 27–30 (Ahrland, Dahlgren, and Persson Citation1990; Perron and Brumaghim Citation2009). Also, larger polyfunctional tannins have been shown to exhibit even stronger metal-binding capacity than that of gallic acid (Mila, Scalbert, and Expert Citation1996). Thus, it may be that large portions of iron are unaffected by the extraction treatment due to the high stability of the iron-tannin complex.
To evaluate the effect of DTPA on the iron-tannin compounds, an extraction experiment was conducted where transverse micro sections of the sample material were treated with the chelating agent. Micro sections were used in order to solely study the effect of DTPA on the complexes: with a volume of ca 1 µL for the micro sections, an equilibrium with the extraction solution was expected to be reached quickly.
The experiment showed that thin sections treated with DTPA appeared generally much lighter in colour after extraction (), which indicates that discolouring matter, such as iron compounds, are extracted. In the sections stored in water, some particles had come loose, but in general the appearance was as dark and contaminated as before (image not shown). The phenomena of filled/dark lumen of rays and vessels were much reduced in the sections treated with DTPA. However, in all sections discolorations and precipitates were still present after extraction, and Raman analysis confirmed that most of them were iron-tannin products (see for example spectra, micrograph of site of analysis Supplementary Figures 4–5). It is possible that some fraction of the iron-tannins had dissolved, but nonetheless, large deposits of iron-tannins were found in all sections, leading us to conclude that a substantial amount remains unaffected.
Figure 7. Micrograph (200x) of one cross section from The Sword before (left) and after extraction (right). It is evident that the extraction has led to the removal of depositions and to a lighter appearance; however, Raman analysis still detects iron-tannin precipitates in the remaining dark areas.
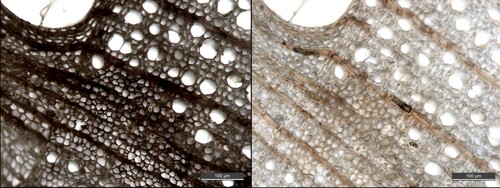
Figure 8. Spatially offset normalised Raman spectra. Example spectra of sample extracted in water (top) and DTPA (bottom). The site of analysis is a contaminated ray and a cell wall respectively. Signature bands of iron-tannin (580, 1328, and 1482 cm−1) are marked. As previously described, the band at 1573 cm−1 is often obscured by the presence of lignin, as can be seen in the top spectrum.
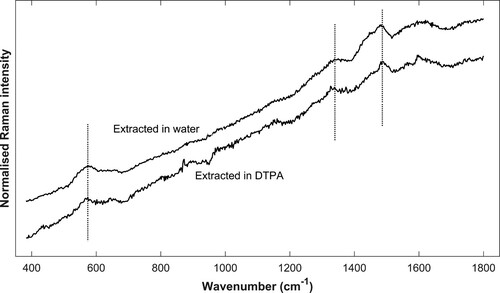
What long-term effect these remaining iron-tannins will have on the treated archaeological wood (shipwreck timbers on display), are difficult to predict. The pro-oxidant mechanism of gallic acid, reduction of Fe3+ to the redox active Fe2+, is dominant in a Fenton-type system when the stoichiometric balance is shifted towards iron (Strlič et al. Citation2002). If larger polyphenols react in the same way, the extraction of other iron species likely reduces the pro-oxidant potential of iron-tannins.
Raman and light microscopic studies on very thin wood sections allows for extraction monitoring of in situ iron compounds without the effects of diffusion or dilution. Diffusion and dilution are circumvented as the volume of the sample is small compared to that of the treatment solution. These effects can otherwise obfuscate extraction experiments on larger objects, e.g. is extraction inefficient because of diffusion, dilution, or dissolution. The transversal sections had a thickness around 50 µm, which allowed the concentrated solution to react almost directly with the wood matrix and the iron-compounds. We believe that this technique is useful when testing the treatment potential of chelating agents in archaeological wood conservation. But it could also be applied to study other reactions, e.g. at what conditions Fe3+-tannins decay into Fe2+ and quinone species (see ).
Conclusion
Archaeological oak often contains elevated concentrations of iron as a consequence of long-term exposure in nature. We found that tannins in oak react with iron and form stable iron-tannin compounds that are difficult to extract. With the help of confocal Raman spectroscopy, iron-tannin precipitates were identified in vessels, lumen, rays, and cell walls of archaeological shipwreck timber. However, dark precipitations were not always related to iron compounds and we conclude that iron precipitation in general cannot be identified solely visually, for example by use of light microscopy. The widespread presence of iron-tannin precipitates suggests that the natural high content of tannins in oak plays an important role for accumulation of iron.
In this study we introduced a novel method for studying the reactions of precipitated compounds on a cellular level in archaeological wood. Extraction experiments on thin transversal wood sections showed that that iron-tannin precipitates are not efficiently removed by treatment with DTPA, a chelating agent often used in conservation treatment. As iron-tannins could decompose into redox active Fe2+, their presence in the wood are of concern. We conclude that further studies of the reactivity of the iron-tannin precipitates in treated museum objects are needed, as well as more detailed studies on the chemical process of iron extraction, especially of the remaining non-soluble compounds.
Supplemental Material
Download Zip (5 MB)Acknowledgements
We thank Chalmers Materials Analysis Laboratory for access to and advice on SEM-EDX analysis.
Disclosure statement
No potential conflict of interest was reported by the author(s).
References
- Ahrland, S., Å Dahlgren, and I. Persson. 1990. “Stabilities and Hydrolysis of Some Iron(III) and Manganese(III) Complexes with Chelating Ligands.” Acta Agriculturae Scandinavica 40: 101–111.
- Ajuong, E. M. A., and M. Redington. 2004. “Fourier Transform Infrared Analyses of Bog and Modern Oak Wood (Quercus petraea) Extractives.” Wood Science and Technology 38: 181–190.
- Almkvist, G., and I. Persson. 2006. “Extraction of Iron Compounds from Wood from the Vasa.” Holzforschung 60: 678–684.
- Almkvist, G., and I. Persson. 2008. “Analysis of Acids and Degradation Products Related to Iron and Sulfur in the Swedish Warship Vasa.” Holzforschung 62: 694–703.
- Almkvist, G., and I. Persson. 2011. “Distribution of Iron and Sulfur and Their Speciation in Relation to Degradation Processes in Wood from the Swedish Warship Vasa.” New Journal of Chemistry 35: 1491–1502.
- Baar, J., Z. Paschová, T. Hofmann, T. Kolář, G. Koch, B. Saake, and P. Rademacher. 2019. “Natural Durability of Subfossil Oak: Wood Chemical Composition Changes Through the Ages.” Holzforschung 74: 47–59.
- Bicchieri, M., M. Monti, G. Piantanida, and A. Sodo. 2013. “Non-destructive Spectroscopic Investigation on Historic Yemenite Scriptorial Fragments: Evidence of Different Degradation and Recipes for Iron Tannic Inks.” Analytical and Bioanalytical Chemistry 405: 2713–2721.
- Björdal, C. G., and Y. Fors. 2019. “Correlation Between Sulfur Accumulation and Microbial Wood Degradation on Shipwreck Timbers.” International Biodeterioration & Biodegradation 140: 37–42.
- Bjurhager, I., H. Halonen, E.-L. Lindfors, T. Iversen, G. Almkvist, E. K. Gamstedt, and L. A. Berglund. 2012. “State of Degradation in Archeological Oak from the 17th Century Vasa Ship: Substantial Strength Loss Correlates with Reduction in (Holo)Cellulose Molecular Weight.” Biomacromolecules 13: 2521–2527.
- Chvátalová, K., I. Slaninová, L. Březinová, and J. Slanina. 2008. “Influence of Dietary Phenolic Acids on Redox Status of Iron: Ferrous Iron Autoxidation and Ferric Iron Reduction.” Food Chemistry 106: 650–660.
- Dedic, D., T. Sandberg, T. Iversen, T. Larsson, and M. Ek. 2014. “Analysis of Lignin and Extractives in the Oak Wood of the 17th Century Warship Vasa.” Holzforschung 68: 419–425.
- Díaz Hidalgo, R. J., R. Córdoba, P. Nabais, V. Silva, M. J. Melo, F. Pina, N. Teixeira, and V. Freitas. 2018. “New Insights into Iron-Gall Inks Through the Use of Historically Accurate Reconstructions.” Heritage Science 6: 63.
- Du Penhoat, C. L. M. H., V. M. F. Michon, S. Peng, C. Viriot, A. Scalbert, and D. Gage. 1991. “Structural Elucidation of new Dimeric Ellagitannins from Quercus Robur L. Roburins A–E.” Journal of the Chemical Society, Perkin Transactions 1: 1653–1660.
- Fojutowski, A., H. Wróblewska, M. Komorowicz, A. Kropacz, A. Noskowiak, and I. Pomian. 2014. “Changes in the Properties of English oak Wood (Quercus robur L.) as a Result of Remaining Submerged in Baltic Sea Waters for Two Years.” International Biodeterioration & Biodegradation 86: 122–128.
- Fors, Y. 2008. “Sulfur-related Conservation Concerns for Marine Archaeological Wood: The Origin, Speciation and Distribution of Accumulated Sulfur with Some Remedies for the Vasa.” diss., Stockholms universitet.
- Fors, Y., H. Grudd, A. Rindby, F. Jalilehvand, M. Sandström, I. Cato, and L. Bornmalm. 2015. “Sulfur and Iron Accumulation in Three Marine-Archaeological Shipwrecks in the Baltic Sea: The Ghost, the Crown and the Sword.” Scientific Reports 4: 4222.
- Fors, Y., F. Jalilehvand, E. Damian Risberg, C. Björdal, E. Phillips, and M. Sandström. 2012. “Sulfur and Iron Analyses of Marine Archaeological Wood in Shipwrecks from the Baltic Sea and Scandinavian Waters.” Journal of Archaeological Science 39: 2521–2532.
- Jordão, A. M., J. M. Ricardo-da-Silva, and O. Laureano. 2005. “Extraction of Some Ellagic Tannins and Ellagic Acid from oak Wood Chips (Quercus pyrenaica L.) in Model Wine Solutions: Effect of Time, pH, Temperature and Alcoholic Content.” South African Journal of Enology and Viticulture 26: 83–89.
- Kalicki, T., and M. Krąpiec. 1995. “Problems of Dating Alluvium Using Buried Subfossil Tree Trunks: Lessons from the ‘Black Oaks’ of the Vistula Valley, Central Europe.” The Holocene 5: 243–250.
- Koch, G., E. Melcher, M.-T. Lenz, and J. Bauch. 2018. “Biological and Topochemical Studies on the Resistance of Excavated oak Piles (Quercus sp.) from a Historical Bridge in Bavaria.” Holzforschung 72: 133–141.
- Komorowicz, M., H. Wróblewska, A. Fojutowski, A. Kropacz, A. Noskowiak, and I. Pomian. 2018. “The Impact of 5 Years’ Underwater Exposure in the Baltic Sea (Puck Bay) on Selected Properties of English Oak Wood Samples.” International Biodeterioration & Biodegradation 131: 40–50.
- Krutul, D., and J. Kocon. 1982. “Inorganic Constituents and Scanning Electron Microscopic Study of Fossil Oak Wood (Quercus sp.).” Holzforschung und Holzverwertung 34: 69–77.
- Lee, A. S., P. J. Mahon, and D. C. Creagh. 2006. “Raman Analysis of Iron Gall Inks on Parchment.” Vibrational Spectroscopy 41: 170–175.
- Lillie, R. D. 1972. “A Histochemical Reaction from 1807: Iron Tannin.” Journal of Histochemistry & Cytochemistry 20: 295–295.
- Lindfors, E.-L., M. Lindström, and T. Iversen. 2008. “Polysaccharide Degradation in Waterlogged Oak Wood from the Ancient Warship Vasa.” Holzforschung 62: 57–63.
- MacLeod, I. D., and V. L. Richards. 1996. “The Impact of Metal Corrosion Products on the Degradation of Waterlogged Wood Recovered from Historic Shipwreck Sites.” In Proceedings of the 6th ICOM Group on wet Organic Archaeological Materials Conference, edited by P. Hoffmann, T. Daley, and T. Grant, 331–349. York: ICOM CC.
- Mila, I., A. Scalbert, and D. Expert. 1996. “Iron Withholding by Plant Polyphenols and Resistance to Pathogens and Rots.” Phytochemistry 42: 1551–1555.
- Moutounet, M., P. Rabier, J. Puech, et al. 1989. “Analysis by HPLC of Extractable Substances in Oak Wood. Application to a Chardonnay Wine.” Sciences des Aliments 9: 35–51.
- Nisula, L. 2018. “Wood Extractives in Conifers: a Study of Stemwood and Knots of Industrially Important Species.” diss., Åbo Akademi University.
- Nkhili, E., M. Loonis, S. Mihai, H. El Hajji, and O. Dangles. 2014. “Reactivity of Food Phenols with Iron and Copper Ions: Binding, Dioxygen Activation and Oxidation Mechanisms.” Food & Function 5: 1186–1202.
- Norbakhsh, S., I. Bjurhager, and G. Almkvist. 2013. “Mimicking of the Strength Loss in the Vasa: Model Experiments with Iron-Impregnated Recent Oak.” Holzforschung 67: 707–714.
- Norbakhsh, S., I. Bjurhager, and G. Almkvist. 2014. “Impact of Iron(II) and Oxygen on Degradation of Oak – Modeling of the Vasa Wood.” Holzforschung 68: 649–655.
- Perron, N. R., and J. L. Brumaghim. 2009. “A Review of the Antioxidant Mechanisms of Polyphenol Compounds Related to Iron Binding.” Cell Biochemistry and Biophysics 53: 75–100.
- Piantanida, G., E. Menart, M. Bicchieri, and M. Strlič. 2013. “Classification of Iron-Based Inks by Means of Micro-Raman Spectroscopy and Multivariate Data Analysis.” Journal of Raman Spectroscopy 44: 1299–1305.
- Ponce, A., L. B. Brostoff, S. K. Gibbons, P. Zavalij, C. Viragh, J. Hooper, S. Alnemrat, K. J. Gaskell, and B. Eichhorn. 2016. “Elucidation of the Fe(III) Gallate Structure in Historical Iron Gall Ink.” Analytical Chemistry 88: 5152–5158.
- Pulido, R., L. Bravo, and F. Saura-Calixto. 2000. “Antioxidant Activity of Dietary Polyphenols As Determined by a Modified Ferric Reducing/Antioxidant Power Assay.” Journal of Agricultural and Food Chemistry 48: 3396–3402.
- Rémazeilles, C., F. Lévêque, E. Conforto, L. Meunier, and P. Refait. 2019. “Contribution of Magnetic Measurement Methods to the Analysis of Iron Sulfides in Archaeological Waterlogged Wood-Iron Assemblies.” Microchemical Journal 148: 10–20.
- Sandström, M., F. Jalilehvand, I. Persson, U. Gelius, P. Frank, and I. Hall-Roth. 2002. “Deterioration of the Seventeenth-century Warship Vasa by Internal Formation of Sulphuric Acid.” Nature 415: 893–897.
- Scalbert, A., B. Monties, and J.-M. Favre. 1988. “Polyphenols of Quercus Robur: Adult Tree and in Vitro Grown Calli and Shoots.” Phytochemistry 27: 3483–3488.
- Strlič, M., T. Radovič, J. Kolar, and B. Pihlar. 2002. “Anti- and Prooxidative Properties of Gallic Acid in Fenton-Type Systems.” Journal of Agricultural and Food Chemistry 50: 6313–6317.
- van Bürck, U., F. E. Wagner, and A. Lerf. 2012. “ Mössbauer Studies of Subfossil Oak.” Hyperfine Interactions 208: 105–110.
- Welling, J., T. Schwarz, and J. Bauch. 2018. “Biological, Chemical and Technological Characteristics of Waterlogged Archaeological Piles (Quercus Petraea (Matt.)Liebl.) of a Medieval Bridge Foundation in Bavaria.” European Journal of Wood and Wood Products 76: 1173–1186.
- Wetherall, K. M., R. M. Moss, A. M. Jones, A. D. Smith, T. Skinner, D. M. Pickup, S. W. Goatham, A. V. Chadwick, and R. J. Newport. 2008. “Sulfur and Iron Speciation in Recently Recovered Timbers of the Mary Rose Revealed via X-ray Absorption Spectroscopy.” Journal of Archaeological Science 35: 1317–1328.
- Zeise, I., Z. Heiner, S. Holz, M. Joester, C. Büttner, and J. Kneipp. 2018. “Raman Imaging of Plant Cell Walls in Sections of Cucumis Sativus.” Plants 7: 7.