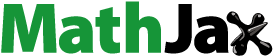
Abstract
Mineralized, bio-inspired nanofibrous scaffolds with controllable architecture and capable of mimicking essential characteristics of bone extracellular matrix at the micro- and nanoscale offer a promising strategy to restore functions or elicit favorable responses for bone tissue regeneration and repair. In this study, a simple approach to fabricate a hybrid scaffold with porous fibers for bone tissue engineering is presented. Non-woven multifunctional nano- and microfibers were fabricated using a block copolymer of poly(ε-caprolactone) (PCL) and poly(lactic acid) (PLA) (PL-b-CL) as a matrix and hydroxyapatite (HA) as a functional agent, dissolved in a binary solvent mixture. Physicochemical and thermal characterization as well as biocompatibility analyses were carried out using SaOS-2 cells. The results showed fibers with highly porous surfaces whose pore diameters range in the nanometer scale and all scaffolds exhibited hydrophobicity. HA-modified scaffolds significantly improved cell metabolic activity and proliferation as compared to pristine scaffolds. The biodegradable and biocompatible scaffolds proposed in this study carry great potential for various biomedical applications and in the future, it is expected that they can be used for controlled drug delivery by incorporating growth factors, proteins, or drugs to reduce the inflammatory response and/or to promote bone repair.
1. Introduction
Whether caused by trauma, congenital abnormalities, cancer, or infection, bone injuries, and defects exhibit a very high incidence in both active and elder groups. Bone diseases are responsible for about 1.5 million fractures each year, and this burden is thought to grow with the increasing aging of the population.[Citation1] Such numbers have raised concerns in terms of costs and risk of complications.[Citation2,Citation3] Insufficient blood supply, bone infection and systemic diseases are common complications that can affect bone repair, causing a delayed union or even nonunion bone fracture. Therefore, the need for implanting a biocompatible healing facilitator structure capable of promoting osteogenesis, osteoinduction and osteoconduction – the so-called bone grafts – emerged.[Citation4,Citation5] These bone substitutes can be harvested directly from the patient’s own body on a different anatomical site (autograft), from decellularized bone of non-self human body (allograft), from decellularized bone of a different species (xenograft) or can be artificially produced using biocompatible materials (synthetic grafts). The use of allografts and xenografts substitutes is limited due to the possible transmission of viruses and diseases, while the employment of autografts is often constrained by the lack of availability.[Citation6] Synthetic grafts do not carry a risk of disease transfer and provide the possibility of utilizing a vast range of materials for tailored fabrication with different morphologies. Nevertheless, a perfect scaffold material has yet to be encountered, and thus autografts remain the gold standard for bone repair.[Citation7,Citation8] Various efforts have been conducted to produce synthetic scaffolds with physiologically-relevant properties.[Citation9,Citation10] An ideal bone tissue engineering scaffold should primarily promote cell growth, which is dependent on the material, mechanical and biological cues, and extracellular matrix (ECM). In particular, the latter is involved in regulating cell adhesion, proliferation, differentiation, and functional characteristics of the mature bone.[Citation11] To replicate the fibrous and porous bone ECM, the versatile, simple, low cost and highly reproducible electrospinning technique has been employed.[Citation12,Citation13] This method allows to obtain ultrafine fibers through the application of a high-voltage electric field to an electrically charged solution.[Citation14] The performance of the electrospinning technique as well as the morphology of the fibers are influenced by several parameters, including environmental (relative humidity and temperature), process (flow rate, distance to the collector, collector rotation speed, voltage, and capillary nozzle diameter), and solution parameters (polymer concentration, polymer molecular weight, solvents, and solution conductivity). The fibrous scaffolds designed with the aforementioned method show enhanced tissue integration capacity due to the high surface-to-volume ratio of these meshes, provide a good environment for cell attachment and proliferation and exhibit high porosity with an interconnected pore structure.[Citation15,Citation16] Over the past 25 years, electrospinning has been extensively studied and the number of published articles has been growing.[Citation17] Even so, there is still a plethora of opportunities to explore, namely the introduction of new materials and encapsulation of proteins/growth factors into the fibers.
Hydroxyapatite (HA, Ca5(OH)(PO4)3) is an osteoconductive, biocompatible and bioactive ceramic with a close resemblance to the mineral portion of natural bones and teeth, and with ability to form direct chemical bonds with the living tissue; nonetheless, it has limited use due to its brittleness.[Citation18] To overcome this and provide a physical support for bone tissue engineering, this ceramic has been combined with other materials, including polymers.[Citation19–23] Poly(ε-caprolactone) (PCL) is a synthetic, semi-crystalline, biodegradable, biocompatible and aliphatic polymer widely used in medical applications.[Citation17] Furthermore, PCL nanofiber scaffolds have shown to increase the osteogenic differentiation of human mesenchymal stem cells (hMSCs), suggesting great potential for bone tissue repair.[Citation24,Citation25] In spite of that, the hydrophobicity and slow degradation of PCL limits its application as a scaffold material.[Citation15] Poly(lactic acid) (PLA), in turn, has a suitable biodegradability, its main degradation product is metabolically non-harmful, it increases the protein adsorption capacity to the material, increases the osteo-inducibility of the material, and is Food and Drug Administration (FDA)-approved for surgical implant material and for drug delivery systems.[Citation26–28] Nevertheless, PLA has low toughness, low ductility and low degradation rate, which are restraining factors for some biomedical areas.[Citation29] Mixing PLA and PCL together can retain the advantages of both polymers, alleviating their disadvantages. However, some immiscibility problems may arise when trying to blend these polymers, which can result in poorer biocompatibility of the final product. For instance, Herrero-Herrero et al. showed that fibrous scaffolds composed of blends of PCL and PLA led to poor cell adhesion and proliferation when using adipose tissue-derived mesenchymal stem cells.[Citation30] However, when using the block copolymer of PCL and PLA as an alternative route to avoid macro-phase separation, better biological results were obtained.[Citation29] Moreover, Su et al. produced drug-loaded electrospun nanofibers using a combination of the same copolymer and collagen to act as ECM for human mesenchymal cells growth and supply them with crucial differentiation factors.[Citation31] This system was able to provide a controlled release of different drugs at different stages and showed good biocompatibility with hMSCs.
With that in mind, a block copolymer of PLA and PCL was blended with HA to produce fibrous electrospun scaffolds with porous fibers for application in non-load bearing bone defects. Nano- and micro-fibrous scaffolds were generated via electrospinning, and characterized according to its morphology, fiber diameter, wettability, chemical composition, thermal properties and biological response. Biocompatibility assays were carried out using the human osteosarcoma cell line (SAOS-2) to evaluate the scaffolds’ potential for bone tissue engineering. To the best of our knowledge, this is the first study applying scaffolds with porous fibers composed by a mix of hydroxyapatite and a block copolymer of PLA and PCL for use in bone tissue engineering.
2. Materials and methods
2.1. Materials
Poly(L-lactide-co-caprolactone) (70:30) (PURASORB® PLC 7015) (PL-b-CL) was supplied from Corbion N.V. with an average molecular weight of 2.0 × 105 g·mol−1. Chloroform was purchased from Carl Roth, while acetone (99.8%) and HA (nanoparticle size = 200 nm) were acquired from Sigma Aldrich. Human osteosarcoma cells (SaOS-2 cell line) were obtained from American Type Culture Collection (ATCC). McCoy’s 5 A medium, penicillin/streptomycin (Pen/Strep, 100 IU·mL−1 and 100 µg·mL−1, respectively), and propidium iodide (PI) were purchased from Sigma Aldrich and fetal bovine serum (FBS) from Gibco™ Life Technologies (Thermo Fisher Scientific). CellTiter96® Aqueous One Solution Cell Proliferation Assay was acquired from Promega. Quant-iT™ dsDNA High-Sensitivity Assay and 3,3′- dihexyloxacarbocyanine iodide (DiOC6(3)) were purchased from Invitrogen (Thermo Fisher Scientific).
2.2. Electrospinning solutions preparation
PL-b-CL was dissolved in 3.5 mL of chloroform to prepare a final solution with 17% (w/v). HA nanoparticles (HA NPs) were sonicated for 1.5 hours in 1.5 mL of acetone to obtain a nanoparticle suspension. Then, the polymeric solution and the HA suspensions with 0, 3 and 7% (w/v) were mixed and stirred overnight to obtain the solutions for electrospinning. The solutions were fed into a 5 mL syringe fitted with a capillary tip of 0.25 mm in diameter, pushed at a flow rate of 0.5 mL·h−1, and drawn into fibers under the application of 15 kV of voltage. Fibers were collected at a distance of 20 cm from the needle tip by a rotating cylindrical drum at a rotating speed of 2500 rpm. The temperature and relative humidity were set at 16 °C, and 80%, respectively.
2.3. Scanning electron microscopy (SEM)
The fiber morphology of the electrospun samples was characterized by SEM (JEOL JSM-IT100; Japan/Zeiss, Germany). Samples were attached to the aluminum stubs with carbon tape and sputtered with a thin film of gold for 60 seconds using a sputter coater device (Crossington Sputter Coater 108 Auto). Fiber diameter was determined by manual measurements using Fiji/ImageJ v1.51 software (National Institutes of Health (NIH), Bethesda, MD, USA).
2.4. Wettability measurements
Contact angles were measured immediately after depositing a 5 μL water droplet from a needle precision tip with an outer diameter of 310 µm, on the composite’s surface using a contact angle measurement setup (Dataphysics OCA 35 equipped with SCA 20 Version 4.3.19 software) based on the static sessile drop mode at room temperature (RT). The contact angle of each sample was determined by averaging the values of ten continuous measurements calculated through the Young-Laplace equation.
2.5. Fourier transform infrared spectroscopy (FTIR)
To identify chemical interactions between the PL-b-CL block copolymer and HA NPs, FTIR analysis was performed using the PerkinElmer Frontier™ FTIR Spectrometer. The FTIR spectra of scaffolds were recorded between 500 cm−1 and 4000 cm−1. Spectroscopic and thermal analysis data were processed and graphed using OriginPro (v8.5.1) software.
2.6. Thermogravimetric analysis (TGA)
Thermogravimetric analysis was performed to evaluate the scaffolds’ thermal stability on a thermogravimetric analyzer (TGA 4000, PerkinElmer). The samples were placed into ceramic crucibles and heated at a rate of 5 °C·min−1, from 30 °C to 800 °C. All thermal analyses were carried out in a nitrogen (N2) atmosphere flowing at 20 mL·min−1.
2.7. Differential Scanning Calorimetry (DSC)
To analyze various phase transitions and effects of functionalizing with HA NPs, a DSC analysis was performed. For this, 10 mg of electrospun mesh was placed into a DSC pan, and subsequently heated at a rate of 5 °C·min−1 from −80 °C to 180 °C in a PerkinElmer double-furnace DSC 8000. All thermal analyses were carried out in a N2 atmosphere flowing at 20 mL· min−1.
2.8. Biocompatibility studies
The obtained fibrous scaffolds were evaluated according to their biocompatibility toward SaOS-2 osteosarcoma-derived cells using distinct biological studies.
2.8.1. Cell culture
SaOS-2 cells (passage 41) were grown in McCoy’s 5 A medium supplemented with 15% (v/v) FBS and 1% (v/v) Pen/Strep. Cells were cultured on tissue culture polystyrene (TCP) flasks and incubated at 37 °C under a 5% CO2 humidified atmosphere. Culture medium was refreshed every 2 days.
2.8.2. Cell seeding on the fibrous surface
The electrospun scaffolds were cut into circular disks with 6 mm diameter to use in standard 96-well cell culture plates. Scaffold sterilization was performed inside a laminar air flow cabin by immersing the scaffolds in 70% (v/v) ethanol for 30 min and subsequently washing them thrice with PBS. Freshly confluent SaOS-2 cells were first rinsed with PBS, followed by incubation in trypsin/EDTA for 3 min at 37 °C and re-suspension in culture medium. SaOS-2 cells were seeded at a density of 89 300 cells/cm2 onto the electrospun meshes. Cells were incubated at 37 °C and 5% CO2 and culture medium was exchanged once every 2 days.
2.8.3. Cell metabolic activity
After 1, 7 and 14 days of cell culture, the metabolic activity of the cells seeded in the fibrous scaffolds was assessed using CellTiter 96® AQueous One Solution Reagent (MTS assay) according to the manufacturer’s directives. Briefly, a mixture of MTS reagent and culture media at a volume ratio of 1:5 was added to each cell-seeded scaffold and incubated for 2h. Absorbance values recorded at 690 nm were subtracted to the absorbance values recorded at 490 nm. For MTS and DNA evaluation, 4 replicates were used per experimental group.
2.8.4. Cell proliferation
DNA extraction was performed by transferring cell-seeded meshes to Eppendorf tubes containing cell lysis buffer (0.2% (v/v) Triton™ X-100, 10 mM Tris, 1 mM EDTA) and subjecting them to three freeze-thaw-vortex cycles. Cell proliferation was determined from the dsDNA amount estimated by the Quant-iT™ dsDNA high sensitivity assay on days 1, 7 and 14 of cell culture, according to the manufacturer’s instructions.
2.8.5. Cell adhesion and morphology
SaOS-2 cells adhesion and morphology were assessed by staining cell internal membranes and nuclei with DiOC6(3) and PI reagents, respectively, on days 7 and 14 of the experiment. Ice-cold methyl-alcohol (–20 °C) was added to each well and incubated at RT for 10 min. After fixation, the cell-seeded meshes were washed with PBS and the DiOC6(3) probe was added at a concentration of 1 µg·mL−1 and incubated for 45 min at RT, protected from light. Next, scaffolds were rinsed with PBS and incubated with PI (5 μg·mL−1) for 10 min at RT in the dark. Lastly, cells were washed with PBS and transferred onto glass microscope slides. Cells were visualized using a confocal laser scanning microscope (Zeiss 880 LSM Airyscan) at λex = 488 and 560 nm and λem = 520 and 580 nm for DiOC6(3) and PI detection, respectively. Three replicates per timepoint were used from each experiment. Images were obtained using Zen Black software and prepared with Adobe Photoshop 2020 software.
2.9. Statistical analysis
GraphPad Prism 9.1.1 software (San Diego, CA, USA) was used to perform statistical analysis. Normally distributed data are represented by mean ± standard deviation and the comparations were carried out using one-way analysis of variance (ANOVA) with Tukey’s post hoc analysis. Data following a non-Gaussian distribution are represented by median ± interquartile range. Results were found statistically significant for p-values < 0.05.
3. Results and discussion
Here, co-electrospinning of PL-b-CL has been performed with HA NPs to obtain functionalized nanofibers for supporting osteogenic SaOS-2 cell growth and differentiation. HA NPs suspended in PL-b-CL solution were stable for a considerable amount of time, which is ideal in electrospinning. However, with the increase in the concentration of HA NPs, its behavior started deviating from the optimized state. This impacted the electrospinning yields and required longer time to obtain similar scale of materials. Further, electrospinning was impacted due to rapid evaporation of solvents which hampered the continuity of the process due to the set up used in this study. After multiple runs to optimize the concentration of the polymer and HA NPs along with the electrospinning stability process, obtained pristine and functionalized nanofibers were collected and analyzed with various physicochemical methods.
3.1. SEM
The morphology and fiber size distribution of PL-b-CL pristine (PL-b-CL), PL-b-CL with 3% (w/v) of HA NPs (PL-b-CL:HA3), and PL-b-CL with 7% (w/v) of HA NPs (PL-b-CL:HA7) scaffolds were analyzed using SEM, and are displayed in . PL-b-CL concentration optimizations were performed to achieve semi-oriented fiber mats with beadless and uniform fibers, resulting in an ideal concentration set at 17% (w/v). Indeed, PL-b-CL pristine fibers exhibited smooth fibers in a semi-aligned oriented mat with heterogenous fiber size distribution. On the contrary, PL-b-CL:HA3 and PL-b-CL:HA7 showed porous and rough fibers in a non-woven mat with heterogeneous fiber size distribution and negligible number of beads. The pores on the surface of PL-b-CL:HA3 and PL-b-CL:HA7 samples can provide a higher surface area for the cells to attach, and offer the possibility for drug incorporation, which shows their potential use as drug delivery or release systems.[Citation32,Citation33] The formation of these pores may have occurred due to the delayed evaporation of volatile solvents, such as chloroform and acetone, which led to a significant decrease in the temperature on the fiber surface and consequent formation of water condensation droplets caused by the high humidity environment. These water droplets acted as a cast, occupying space on the surface of the fiber, and once the water and solvent have evaporated, pores were formed.[Citation34,Citation35] This process of pore formation on the fiber surface is already described in the literature and known as the breath figure mechanism. In fact, chloroform has been reported as an inducer of pore formation in PLA.[Citation36,Citation37] Since the block copolymer used in the present study is composed by 70% of PLA, and electrospun under high humidity environment, it is likely that pores were formed according to that mechanism. The rough surface of the fibers, on the other hand, can be attributed to the encapsulation of HA within the PL-b-CL matrix.
Figure 1. SEM images of the different electrospun samples and their fiber diameter distribution histograms. All images were acquired at a magnification of 4500×; scale bars: 5 µm.
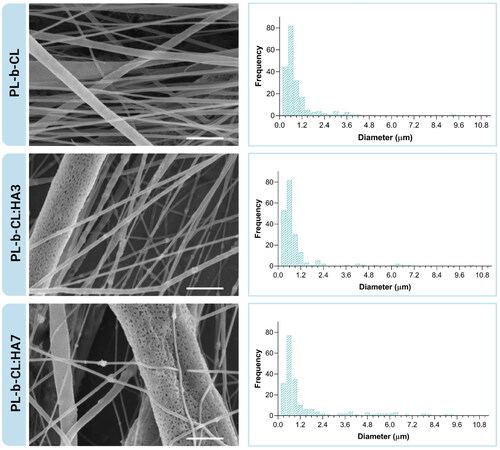
Fiber diameter histograms of PL-b-CL, PL-b-CL:HA3 and PL-b-CL:HA7 displayed a multimodal frequency distribution ranging between 0.3 and 9.3 µm, 0.3 and 10.2 µm and 0.3 and 9.3 µm, respectively. In addition, 76% of the PL-b-CL fibers, 81% of the PL-b-CL:HA3 fibers and 69% of the PL-b-CL:HA7 fibers were found to have a diameter inferior to 1 µm. According to these results, all samples were composed by both nano- and microfibers, with higher amount of nanofibers.
Previous studies in electrospun scaffolds for bone tissue engineering have shown that nano- and micro-fibrous combined scaffolds promote higher cell viability, proliferation and enhanced differentiation compared to microfiber scaffolds.[Citation38,Citation39] Additionally, it is important to note that no significant differences can be found in terms of diameter between PL-b-CL and PL-b-CL:HA3 fibers. However, with the increase of the HA concentration, a larger number of fibers with greater diameters appear (). This is due to the incorporation of HA into the polymer solution which contributed to an increase in the solution viscosity, augmenting the diameter of the randomly oriented fibers, and making the electrospinning process of the PL-b-CL:HA7 solution more challenging.
Table 1. Fiber diameter of the different types of scaffolds, measured manually using Fiji/ImageJ software.
3.2. Wettability
Water droplet contact angle method was performed to evaluate the wettability of the meshes. The mean contact angles of PL-b-CL pristine, PL-b-CL:HA3 and PL-b-CL:HA7 are depicted in . All composites exhibited poor wettability with the contact angle increasing slightly with the addition of HA (PL-b-CL (θ) = 142.62 ± 5.44°; PL-b-CL:HA3 (θ) = 145.39 ± 5.31°; PL-b-CL:HA7 (θ) = 148.40 ± 5.53°). These results were not in accordance with the literature, which reports that as HA is a hydrophilic component, its addition should decrease the contact angle, making the matrices with HA more hydrophilic than the pristine PL-b-CL sample.[Citation40–42] This slight increase in the hydrophobicity of samples with the addition of HA may be explained by the increase in porosity or roughness of the matrix.[Citation37,Citation43] By observing the SEM images, it was possible to identify rough and porous fibers, which may have resulted in a slight increment in the hydrophobicity of membranes whose contact angle was already above 90°.[Citation44–46] In fact, in , the embedding of HA NPs into the polymeric matrix is evident; therefore, the expected decrease in hydrophobicity of nanofibers did not occur.
3.3. FTIR
FTIR spectra of electrospun PL-b-CL pristine and PL-b-CL with 3 and 7% (w/v) of HA scaffolds was performed to confirm the presence of the block copolymer and HA NPs in the samples, as presented in . FTIR spectrum of PL-b-CL pristine exhibited several peaks corresponding to PCL namely, a -CH2 symmetric stretch at 2947 cm−1, a -CH2 asymmetric stretch at 2856 cm−1, C-O stretch at around 1186 cm−1 and 1044 cm−1, and a band at 1746 cm−1 associated with the overlapped carbonyl stretch of PCL and PLA.[Citation47] Additionally, PLA peaks can be observed at 1452, 1361 and 1089 cm−1, which are associated with the asymmetric and symmetric bending vibrations of -CH3 and stretching of C-O, respectively.[Citation48] A peak at 753 cm−1 can also be identified in all scaffolds which is attributed to chloroform residues.[Citation49] These PL-b-CL characteristic peaks were found in all FTIR spectra (PL-b-CL, PL-b-CL:HA3 and PL-b-CL:HA7), therefore indicating its presence in those samples. Besides, the FTIR profile of PL-b-CL:HA3 and PL-b-CL:HA7 showed peaks at 566 and 605 cm−1 corresponding to v4 vibrational band of HA, which confirms the successful incorporation of HA NPs into the PL-b-CL scaffolds.[Citation50,Citation51] Furthermore, the minor changes in peak positions noticeable in the -CH2 symmetric stretch and in the C-O stretch can be attributed to chemical interactions between the block copolymer and HA.
3.4. TGA
Thermogravimetric analysis was used to investigate the effect of immobilization of HA NPs at varying concentrations on the thermostability of nanofibers scaffolds, and thermograms are displayed in . The characteristic temperatures, including the onset, offset, and inflection temperature along with total weight loss for all studied samples are tabulated in . All formulations exhibited a similar profile, with a two-step decomposition involving the decomposition of amorphous PLA (first step, temperature interval (ΔT) = 200 – 365 °C) and low-crystallinity PCL (second step, ΔT = 365–420 °C).[Citation52] The PL-b-CL:HA3 and PL-b-CL:HA7 profiles were shifted toward lower temperatures compared to those of pristine PL-b-CL, thus suggesting reduced thermostability by the addition of HA NPs; nevertheless, the PL-b-CL:HA7 profile slightly shifted toward higher temperatures compared to PL-b-CL:HA3, indicating enhanced thermostability by high HA NPs concentration. Specifically, compared with PL-b-CL, the inflection temperature of the PL-b-CL:HA3 sample, decreased by 6.8 °C in the first step and 23.19 °C in the second step, as did the offset temperature which shifted downwards by 19.56 °C. Similarly, the PL-b-CL:HA7 sample showed a decrease of 1.24 °C and 11.84 °C in the first and second inflection point, respectively, and 7.65 °C in the offset temperature, compared to PL-b-CL pristine, but when compared with PL-b-CL:HA3 samples, the inflection point increased by 5.56 °C and the offset temperature by 11.91 °C. This slight improvement in thermal stability of PL-b-CL:HA7 in relation to PL-b-CL:HA3 can be explained by the formation of hydrogen bonds and van der Waals’ forces between the inorganic nanoparticles and the polymer chains.[Citation53] Indeed, PL-b-CL:HA3 followed a faster degradative kinetics (Tonset = 303.02 °C, Toffset = 348.20 °C), whereas PL-b-CL:HA7 revealed a broader weight loss profile, emphasizing a better molecular interaction between the block copolymer and the HA NPs in this formulation (Tonset = 306.21 °C, Toffset = 360.11 °C).[Citation54] Although these results are in agreement with the literature where the addition of HA to the polymer has been shown to decrease its thermal stability, analysis of the data revealed a slight deviation in case of nanofibers with highest concentration of HA NPs.[Citation55] As expected, the residual weights increased linearly with increasing concentration of HA NPs in electrospinning solutions (), confirming the nominal content of the added nanofillers in the fibrous scaffold.[Citation56]
Figure 4. (A) Thermogravimetric analysis (TGA) and (B) derivative gravimetric (DTG) curves of PL-b-CL microfibers containing different amounts of HA.
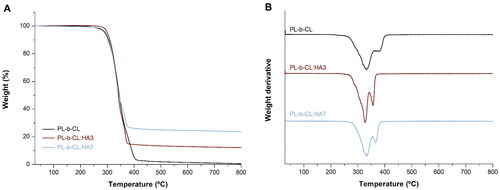
Table 2. Onset, inflection point and offset temperatures and total weight loss of the block copolymer pristine with different concentrations of HA.
3.5. DSC
Main phase transitions of PL-b-CL, PL-b-CL:HA3 and PL-b-CL:HA7 scaffolds were identified by DSC as can be observed in . The DSC thermogram of PL-b-CL pristine showed a glass transition temperature (Tg) at 46.3 °C, a crystallization temperature (Tc) at 81.9 °C whose associated enthalpy (ΔHc) was 0.43 J·g−1 and a melting peak (melting temperature –Tm) was noticed at 160.6 °C with a corresponding enthalpy (ΔHm) of 2.35 J·g−1. Tg of PL-b-CL revealed to be lower than that of PLA (Tg = 55 °C), which was due to the presence of PCL whose Tg is rather low (Tg = − 60 °C).[Citation57]
Figure 5. DSC curves of PL-b-CL, PL-b-CL:HA3 and PL-b-CL:HA7 electrospun samples, with the identified characteristic temperatures. Tg – Glass transition temperature; Tc –crystallization temperature; Tm – melting temperature.
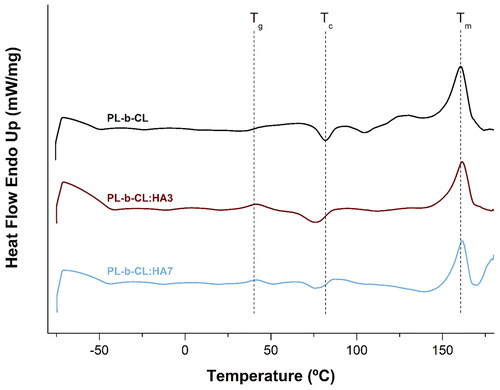
As the ratio of PLA to PCL is 70:30, it was already predictable that the Tg would be closer to PLA than to PCL. DSC thermograms of PL-b-CL:HA3 and PL-b-CL:HA7 demonstrated shifts in the Tg value and in the crystallization and melting peaks, in comparison to PL-b-CL pristine membrane (). In both samples with HA, the Tg value decreased to lower temperatures, which can be explained by the different distribution of HA particles. In other words, the interaction between the polymer and the HA modifies the mobility of the block copolymer chains, since at Tg the molecular chains become more flexible.[Citation58,Citation59] It is also possible to observe a decrease in the crystallization temperature of the samples with HA compared with that of the PL-b-CL pristine. This indicates a faster crystallization enhanced by the addition of HA, which facilitates the packing of PL-b-CL chains into crystalline structures. Compared with PL-b-CL, the crystallization enthalpy of PL-b-CL:HA3 increases, but that of PL-b-CL:HA7 decreases as well as the melting enthalpy of both HA matrices. These results suggest that the nucleation and crystallization process of PL-b-CL may be hampered by the higher concentration of HA NPs.[Citation60,Citation61] In addition, this process as well as the presence of more imperfect crystals within the polymer-HA matrix were responsible for the slight increase in the melting temperature.[Citation59,Citation61] This increase remains unaltered by the increase of HA concentration in the samples.
Table 3. DSC analysis of the block copolymer pristine and with different concentrations of HA.
3.6. Biocompatibility studies
The generated scaffolds are aimed at the repair of non-load bearing bone defects, which still represent a significant clinical problem.[Citation62] The ideal scaffold should be biodegradable, biocompatible and provide structural support for cells to adhere and proliferate.[Citation63] Biodegradability is ensured by the presence of biodegradable aliphatic polyesters (PCL and PLA)[Citation64] and biocompatibility was evaluated through cell metabolic activity, proliferation and adhesion assays.
SaOS-2 is a osteosarcoma cell line isolated from the bone of a 11 year old white female, which displays several osteoblastic features, namely the capacity of depositing a mineralization-competent extracellular matrix.[Citation65] Because of this similarity to osteoblasts, SaOS-2 cells were herein used as an in vitro model of mature osteoblasts.
SaOS-2 cells’ metabolic activity was assessed using an MTS assay on days 1, 7 and 14 of the experiment and is represented in . On day 1 and 7, higher absorbance was observed on TCP compared to all scaffolds (p < 0.001 for all scaffolds in both timepoints). Cells seeded on TCP reached confluence before day 14 and detached, thus no cell viability and proliferation data are presented on this timepoint. The MTS profile of SaOS-2 cells seeded on all electrospun scaffolds showed a continuous increase in cell metabolic activity throughout the culture period, which demonstrated the scaffold biocompatibility. Cells grown on scaffolds with HA (PL-b-CL:HA3 and PL-b-CL:HA7) demonstrated significantly higher metabolic activity than PL-b-CL pristine on day 14 (p = 0.0178 for PL-b-CL:HA3 and p = 0.0015 for PL-b-CL:HA7 compared with PL-b-CL pristine). No significant differences were found between PL-b-CL:HA3 and PL-b-CL:HA7 meshes during the experimental period.
Figure 6. (A) Metabolic activity and (B) dsDNA amount of SaOS-2 cells seeded on TCP and PL-b-CL pristine, PL-b-CL:HA3 and PL-b-CL:HA7 electrospun scaffolds on days 1, 7 and 14 of culture. Data represent mean ± SD (n ≤ 4). One-way ANOVA with Tukey’s post hoc analysis, *p ˂ 0.05, **p ˂ 0.01, ****p ˂ 0.0001.
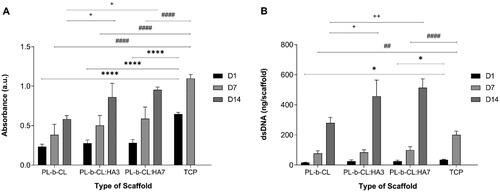
Cell proliferation on PL-b-CL, PL-b-CL:HA3 and PL-b-CL:HA7 matrices was evaluated by dsDNA quantification on days 1, 7 and 14 of the experiment and is depicted in . Cell proliferation data revealed a similar trend to the results obtained from the metabolic activity of cells. The dsDNA amount in all substrates rose considerably from day 1 to day 14, indicating that they were able to promote cell proliferation. TCP showed higher dsDNA amount compared to PL-b-CL and PL-b-CL:HA7 on day 1 and 7 (Day 1: p = 0.0240 for PL-b-CL, p = 0.0381 for PL-b-CL:HA7; Day 7: p = 0.0077 for PL-b-CL, p < 0.001 for PL-b-CL:HA7). On the second week of cell culture, the dsDNA amounts of SaOS-2 cells cultured on scaffolds containing HA NPs were significantly superior to those on PL-b-CL pristine meshes (p = 0.0137 for PL-b-CL:HA3 and p = 0.0002 for PL-b-CL:HA7 compared with PL-b-CL pristine), suggesting that the addition of HA NPs promotes the recovery and proliferation of SaOS-2 cells. The cell metabolic activity and proliferation of cells cultured on fibrous scaffolds are lower than those of TCP, which can be related to its inherent chemically treated positive charge that promotes cell attachment by means of negative charge protein binding. However, given that in all scaffolds there was an increase of both absorbance and dsDNA amount throughout the experimental period, it is therefore inferred that the meshes prepared are not cytotoxic toward SaOS-2 cells.
Cell adhesion to a substrate is essential to regulate a wide variety of cellular processes, such as survival, migration and differentiation,[Citation66] and thus it is important to observe whether the matrix promotes cell attachment. DiOC6(3)/PI staining of SaOS-2 cells grown on PL-b-CL, PL-b-CL:HA3 and PL-b-CL:HA7 meshes demonstrated that cells were able to adhere and proliferate in all substrates and no substantial cell loss was observed during the experimental period. In fact, these can be confirmed by the high cell density detected as early as day 7 and supported by the metabolic activity and cell proliferation results. Cell attachment is influenced by the scaffold physical and chemical properties, such as certain functional groups, solubility, crystallinity, electric charge, surface energy, surface roughness, topography, pore size and stiffness. Moreover, cell attachment is mediated by the proteins adsorbed on the material’s surface.[Citation67] Hydrophilic surfaces have been recognized as the most favorable for SaOS-2 cell adhesion, since it is mediated by the a priori adhesion of proteins to the surface, and these proteins, when exposed to a hydrophobic surface, undergo a conformational change leading to a decrease in accessible focal adhesion sites.[Citation68,Citation69] Although the scaffolds produced in our study were hydrophobic in nature, it is possible that the successful cell adhesion was a result from their rough surface and presence of HA.[Citation70] In fact, various studies have demonstrated that fiber’s roughness enhance cellular responses.[Citation71–73] For example, studies from Zamani et al. and Zhou et al. revealed that rough fiber surfaces and nanopores instigate the adhesion, growth and proliferation of nerve cells and vascular smooth muscle cells, respectively.[Citation71,Citation72] Furthermore, on day 7 and 14, cells cultivated on the scaffolds with HA NPs were homogeneously distributed (). Unfortunately, cell morphology is not easily discernible due to the high adsorption of DiOC6(3) dye by the fibers. This stronger green color may be explained by the binding of the positive charges of the DiOC6(3) dye[Citation74] to the negative charges of the carbonyl groups of PCL and PLA, resulting in a green color stronger than the internal membranes of the cell and thus making the latter imperceptible. However, the morphology of cells tends to be oval or round as previously described in a study by Markazy et al.[Citation75]
Figure 7. Confocal microscope microphotographs of SaOS-2 cells seeded on PL-b-CL pristine, PL-b-CL:HA3 and PL-b-CL:HA7 electrospun scaffolds after 7 and 14 days of culture. Cells’ internal membranes were stained with DiOC6(3) (green) and cell nuclei counterstained with PI (red). Objective 20 ×; scale bars: 50 µm.
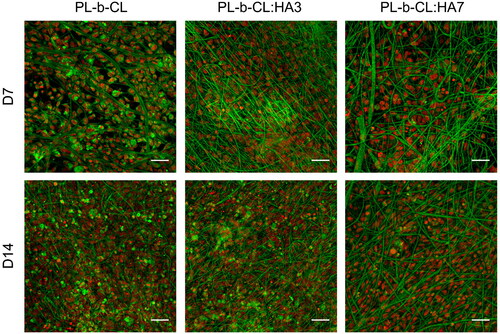
PCL and PLA are among the more widely studied biopolymers to generate fibrous scaffolds for tissue engineering, including bone.[Citation28,Citation76–80] As the produced scaffolds are based on the copolymerization of these two polymers, good biocompatibility results were already expected. In fact, studies with normal human dermal fibroblasts (NHDF), human bladder cancer cells (HBCC), stromal, embryonic and bone cells have already demonstrated that these cells were able to adhere, grow and proliferate on PL-b-CL scaffolds, thus showing the biocompatibility of this material.[Citation81–83] HA, being a bioactive and osteoconductive material, was incorporated into the block copolymer matrix to promote cell-matrix interaction and to mimic the native bone ECM microenvironment.[Citation84] Numerous studies have reported that the addition of HA to different materials has improved the scaffolds’ biocompatibility, osteoconductivity and biomechanical properties.[Citation19,Citation85–90] HA interactions with bone proteoglycans, bone peptides and calcium are critical for bone formation.[Citation91–93] For instance, Lü et al. investigated the effect of the incorporation of HA on nanofibrous scaffolds of poly(3-hydroxybutyrate-co-3-hydroxyvalerate) (PHBV) on mesenchymal stem cells (MSCs) osteogenic differentiation.[Citation85] Higher ALP expression level was obtained on PHBV-HA scaffolds in normal growth medium than on PHBV scaffolds in osteogenic medium, suggesting that HA has a higher stimulating potential than the osteogenic medium. Likewise, Fu et al. demonstrated that the HA present on poly(lactic-co-glycolic acid) (PLGA) nanofibrous matrices promoted greater MC3T3-E1 cell adhesion and proliferation than PLGA pristine matrices.[Citation94] HA doped on the surface of coaxial PCL-polyvinylalcohol (PVA) nanofibers, forming a layer of a depth 800 nm, showed increased osteogenic differentiation of hMSC compared to 200 or 400 nm of HA layer.[Citation95] Similarly, in our study, the nanoparticles of HA appear to have a positive influence on cell adhesion, viability, and proliferation. Indeed, SaOS-2 cells were able to proliferate better on scaffolds with HA NPs than without, indicating that PL-b-CL:HA3 and PL-b-CL:HA7 have better biocompatibility than the PL-b-CL meshes. Moreover, the incorporation of HA into the polymer matrix also prevents fibrous structure of the scaffolds while subsequent application of HA was shown to lose fibrous character of nanofibrous scaffold.[Citation95]
HA particle size has been reported to influence the cytotoxicity of this material. HA NPs have been used in various studies as their crystal size mimics the nanostructure of bone’s inorganic phase, and have been shown to improve the initial attachment, adhesion and spreading as well as the long-term cell proliferation and differentiation.[Citation96,Citation97] However, the literature still shows discrepancies regarding the optimal particle size for improved biocompatibility. For instance, a study by Filová et al. demonstrated that, on day 3 post-seeding, HA NPs impregnated in a matrix composed of polyamide and PDMS promoted significantly the attachment and growth of human osteoblast-like MG-63 cells compared to microparticles.[Citation98] However, by day 7, cell proliferation was more pronounced on micro-HA scaffolds. Moreover, they demonstrated that high concentrations of micro-HA (i.e., above 20 vol%) were cytotoxic. In our studies, HA NPs were chosen as a filler, but further in vitro experiments should be performed to evaluate the size-effects of HA on SaOS-2 cells.
In addition to chemical composition, wettability and surface roughness, the distribution of HA on both the core and surface of the fibers, facilitated by the electrospinning process, may also affect cell adhesion, proliferation, and differentiation. However, we did not see any differences in metabolic activity and amount of dsDNA between PL-b-CL:HA3 and PL-b-CL:HA7, although significant differences were found when compared to pristine PL-b-CL, which showed lower metabolic activity as well as lower amount of dsDNA in both HA-modified scaffolds at day 14. These results are promising, but the scaffolds need to be evaluated for long-term toxicological and differentiation studies and for further in vivo testing in relevant non-load bearing bone defect models such as, the skull.
All in all, the formulations generated in the present study, especially those containing HA, showed potential as scaffolds for application in bone defect repair and regeneration.
4. Conclusion
Scaffolds of PL-b-CL with different concentrations of HA NPs were successfully produced using a versatile electrospinning method. All the formulations resulted in beadless mats with a combined size of nano- and microfibers. The contact angles obtained for each blend revealed to be above 140°, thus quite hydrophobic, with this value increasing with the HA concentration. PL-b-CL pristine scaffolds were found to be more thermally stable than those with HA, of which samples with higher HA concentration exhibited higher thermal stability than those with lower concentration. Unfortunately, as the concentration of HA NPs increased, the electrospinning process became more challenging, due to the rapid evaporation of solvent, leading to solidification of the polymer at the capillary opening. In order to overcome this limitation, a higher flow rate was considered. Additionally, a needleless or co-axial electrospinning approach could be used, mitigating the problem of rapid evaporation at high concentrations of HA NPs. Biological analysis demonstrated that all scaffolds supported SaOS-2 cell proliferation and adhesion, however the scaffolds immobilized with HA NPs exhibited superior performance. Overall, promising results were obtained with scaffolds generated from a block copolymer of PCL and PLA with embedded HA NPs, reinforcing the potential of these scaffolds for bone tissue engineering. Further studies on cell differentiation, and mechanical, osteoinductive and osteoconductive properties of the matrices are recommended to assess these materials as suitable bone substitutes.
Acknowledgments
This project has received funding from the European Union’s HORIZON 2020 MSCA-RISE Marie Skłodowska-Curie Research and Innovation Staff Exchange Research Program 2018 under grant agreement no. 824007 – iP-OSTEO.
Conflicts of interest
The authors report no conflicts of interest in this work.
Additional information
Funding
References
- U.S. Department of Health and Human Services. Bone Health and Osteoporosis: A Report of the Surgeon General; U.S. Department of Health and Human Services, Office of the Surgeon General, Eds.; Office of the Surgeon General (US): Rockville MD, 2004.
- de Villiers, T. J.; Goldstein, S. R. Bone Health 2022: An Update. Climacteric 2022, 25, 1–3. DOI: 10.1080/13697137.2021.1965408.
- Wiese, A.; Pape, H. C. Bone Defects Caused by High-Energy Injuries, Bone Loss, Infected Nonunions, and Nonunions. Orthop Clin North Am 2010, 41, 1–4. DOI: 10.1016/j.ocl.2009.07.003.
- Van Gaalen, S.; Kruyt, M.; Meijer, G.; Mistry, A.; Mikos, A.; Beucken, J. van Den; Jansen, J.; De Groot, K.; Cancedda, R.; Olivo, C.; et al. Tissue Engineering of Bone. In Tissue Engineering; Blitterswijk, C. van, Thomsen, P., Lindahl, A., Hubbell, J., Williams, D. F., Cancedda, R., de Bruijn, J. D., Sohier, J., Eds.; Elsevier Inc.: Amsterdam, 2008; pp 559–610. DOI: 10.1016/B978-0-12-370869-4.00019-7.
- Oryan, A.; Alidadi, S.; Moshiri, A.; Maffulli, N. Bone Regenerative Medicine: Classic Options, Novel Strategies, and Future Directions. J. Orthop. Surg. Res. 2014, 9, 18. DOI: 10.1186/1749-799X-9-18.
- Campana, V.; Milano, G.; Pagano, E.; Barba, M.; Cicione, C.; Salonna, G.; Lattanzi, W.; Logroscino, G. Bone Substitutes in Orthopaedic Surgery: From Basic Science to Clinical Practice. J. Mater. Sci. 2014, 25, 2445–2461. DOI: 10.1007/S10856-014-5240-2/FIGURES/8.
- Valtanen, R. S.; Yang, Y. P.; Gurtner, G. C.; Maloney, W. J.; Lowenberg, D. W. Synthetic and Bone Tissue Engineering Graft Substitutes: What is the Future? Injury. 2021, 52 Suppl 2, S72–S77. DOI: 10.1016/J.INJURY.2020.07.040.
- Turnbull, G.; Clarke, J.; Picard, F.; Riches, P.; Jia, L.; Han, F.; Li, B.; Shu, W. 3D Bioactive Composite Scaffolds for Bone Tissue Engineering. Bioact. Mater. 2018, 3, 278–314. DOI: 10.1016/j.bioactmat.2017.10.001.
- Wu, D. T.; Munguia-Lopez, J. G.; Cho, Y. W.; Ma, X.; Song, V.; Zhu, Z.; Tran, S. D. Polymeric Scaffolds for Dental, Oral, and Craniofacial Regenerative Medicine. Molecules. 2021, 26, 7043. DOI: 10.3390/MOLECULES26227043.
- García, A.; Cabañas, M. V.; Peña, J.; Sánchez-Salcedo, S. Design of 3d Scaffolds for Hard Tissue Engineering: From Apatites to Silicon Mesoporous Materials. Pharmaceutics. 2021, 13, 1981. DOI: 10.3390/PHARMACEUTICS13111981.
- Lin, X.; Patil, S.; Gao, Y. G.; Qian, A. The Bone Extracellular Matrix in Bone Formation and Regeneration. Front. Pharmacol. 2020, 11, 757. DOI: 10.3389/FPHAR.2020.00757.
- Shi, X.; Zhou, W.; Ma, D.; Ma, Q.; Bridges, D.; Ma, Y.; Hu, A. Electrospinning of Nanofibers and Their Applications for Energy Devices. J. Nanomater. 2015, 2015, 1–20. DOI: 10.1155/2015/140716.
- Rivero, P. J.; Redin, D. M.; Rodríguez, R. J. Electrospinning: A Powerful Tool to Improve the Corrosion Resistance of Metallic Surfaces Using Nanofibrous Coatings. Metals. 2020, 10, 350. DOI: 10.3390/met10030350.
- Zhong, W. Nanofibres for Medical Textiles. In Advances in Smart Medical Textiles: Treatments and Health Monitoring; L. van Langenhove, Ed.; Elsevier Inc.: Canada, 2016; Vol. 1, pp 57–70. DOI: 10.1016/B978-1-78242-379-9.00003-7.
- Cho, S. J.; Jung, S. M.; Kang, M.; Shin, H. S.; Youk, J. H. Preparation of hydrophilic PCL nanofiber scaffolds via electrospinning of PCL/PVP-b-PCL block copolymers for enhanced cell biocompatibility. Polymer 2015, 69, 95–102. DOI: 10.1016/j.polymer.2015.05.037.
- Amler, E.; Filová, E.; Buzgo, M.; Prosecká, E.; Rampichová, M.; Nečas, A.; Nooeaid, P.; Boccaccini, A. R. Functionalized Nanofibers as Drug-Delivery Systems for Osteochondral Regeneration. Nanomedicine 2014, 9, 1083–1094. DOI: 10.2217/NNM.14.57.
- Mochane, M. J.; Motsoeneng, T. S.; Sadiku, E. R.; Mokhena, T. C.; Sefadi, J. S. Morphology and Properties of Electrospun PCL and Its Composites for Medical Applications: A Mini Review. Appl. Sci. 2019, 9, 2205. DOI: 10.3390/app9112205.
- Chen, J.; Yu, Q.; Zhang, G.; Yang, S.; Wu, J.; Zhang, Q. Preparation and Biocompatibility of Nanohybrid Scaffolds by in Situ Homogeneous Formation of Nano Hydroxyapatite from Biopolymer Polyelectrolyte Complex for Bone Repair Applications. Colloids. Surf. B. Biointerfaces. 2012, 93, 100–107. DOI: 10.1016/j.colsurfb.2011.12.022.
- Prosecká, E.; Rampichová, M.; Vojtová, L.; Tvrdík, D.; Melčáková, Š.; Juhasová, J.; Plencner, M.; Jakubová, R.; Jančář, J.; Nečas, A.; et al. Optimized Conditions for Mesenchymal Stem Cells to Differentiate into Osteoblasts on a Collagen/Hydroxyapatite Matrix. J. Biomed. Mater. Res. A. 2011, 99, 307–315. DOI: 10.1002/JBM.A.33189.
- Prosecká, E.; Rampichová, M.; Litvinec, A.; Tonar, Z.; Králíčková, M.; Vojtová, L.; Kochová, P.; Plencner, M.; Buzgo, M.; Míčková, A.; et al. Collagen/Hydroxyapatite Scaffold Enriched with Polycaprolactone Nanofibers, Thrombocyte-Rich Solution and Mesenchymal Stem Cells Promotes Regeneration in Large Bone Defect In Vivo. J. Biomed. Mater. Res. A. 2015, 103, 671–682. DOI: 10.1002/JBM.A.35216.
- Zhang, H.; Mao, X.; Du, Z.; Jiang, W.; Han, X.; Zhao, D.; Han, D.; Li, Q. Three Dimensional Printed Macroporous Polylactic Acid/Hydroxyapatite Composite Scaffolds for Promoting Bone Formation in a Critical-Size Rat Calvarial Defect Model. Sci. Technol. Adv. Mater. 2016, 17, 136–148. DOI: 10.1080/14686996.2016.1145532.
- Blahnová, V. H.; Vojtová, L.; Pavliňáková, V.; Muchová, J.; Filová, E. Calcined Hydroxyapatite with Collagen I Foam Promotes Human MSC Osteogenic Differentiation. Int. J. Mol. Sci. 2022, 23, 4236. DOI: 10.3390/IJMS23084236.
- Xing, F.; Chi, Z.; Yang, R.; Xu, D.; Cui, J.; Huang, Y.; Zhou, C.; Liu, C. Chitin-Hydroxyapatite-Collagen Composite Scaffolds for Bone Regeneration. Int. J. Biol. Macromol. 2021, 184, 170–180. DOI: 10.1016/J.IJBIOMAC.2021.05.019.
- Xue, R.; Qian, Y.; Li, L.; Yao, G.; Yang, L.; Sun, Y. Polycaprolactone Nanofiber Scaffold Enhances the Osteogenic Differentiation Potency of Various Human Tissue-Derived Mesenchymal Stem Cells. Stem. Cell. Res. Ther. 2017, 8, 1–9. DOI: 10.1186/S13287-017-0588-0/FIGURES/6.
- Rampichová, M.; Chvojka, J.; Buzgo, M.; Prosecká, E.; Mikeš, P.; Vysloužilová, L.; Tvrdík, D.; Kochová, P.; Gregor, T.; Lukáš, D.; et al. Elastic Three-Dimensional Poly (ε-Caprolactone) Nanofibre Scaffold Enhances Migration, Proliferation and Osteogenic Differentiation of Mese. Cell. Prolif. 2013, 46, 23–37. DOI: 10.1111/CPR.12001.
- Wei, S.; Ma, J. X.; Xu, L.; Gu, X. S.; Ma, X. L. Biodegradable Materials for Bone Defect Repair. Mil. Med. Res. 2020, 7, 54. DOI: 10.1186/s40779-020-00280-6.
- Dorati, R.; Pisani, S.; Maffeis, G.; Conti, B.; Modena, T.; Chiesa, E.; Bruni, G.; Musazzi, U. M.; Genta, I. Study on Hydrophilicity and Degradability of Chitosan/Polylactide-Co-Polycaprolactone Nanofibre Blend Electrospun Membrane. Carbohydr. Polym. 2018, 199, 150–160. DOI: 10.1016/j.carbpol.2018.06.050.
- Yao, Q.; Cosme, J. G. L.; Xu, T.; Miszuk, J. M.; Picciani, P. H. S.; Fong, H.; Sun, H. Three Dimensional Electrospun PCL/PLA Blend Nanofibrous Scaffolds with Significantly Improved Stem Cells Osteogenic Differentiation and Cranial Bone Formation. Biomaterials. 2017, 115, 115–127. DOI: 10.1016/j.biomaterials.2016.11.018.
- Carvalho, J. R. G.; Conde, G.; Antonioli, M. L.; Dias, P. P.; Vasconcelos, R. O.; Taboga, S. R.; Canola, P. A.; Chinelatto, M. A.; Pereira, G. T.; Ferraz, G. C. Biocompatibility and Biodegradation of Poly(Lactic Acid) (PLA) and an Immiscible PLA/Poly(ε-Caprolactone) (PCL) Blend Compatibilized by Poly(ε-Caprolactone-b-Tetrahydrofuran) Implanted in Horses. Polym. J. 2020, 52, 629–643. DOI: 10.1038/s41428-020-0308-y.
- Herrero-Herrero, M.; Alberdi-Torres, S.; González-Fernández, M. L.; Vilariño-Feltrer, G.; Rodríguez-Hernández, J. C.; Vallés-Lluch, A.; Villar-Suárez, V. Influence of Chemistry and Fiber Diameter of Electrospun PLA, PCL and Their Blend Membranes, Intended as Cell Supports, on Their Biological Behavior. Polym. Test. 2021, 103, 107364. DOI: 10.1016/j.polymertesting.2021.107364.
- Su, Y.; Su, Q.; Liu, W.; Lim, M.; Venugopal, J. R.; Mo, X.; Ramakrishna, S.; Al-Deyab, S. S.; El-Newehy, M. Controlled Release of Bone Morphogenetic Protein 2 and Dexamethasone Loaded in Core-Shell PLLACL-Collagen Fibers for Use in Bone Tissue Engineering. Acta. Biomater. 2012, 8, 763–771. DOI: 10.1016/J.ACTBIO.2011.11.002.
- Khajavi, R.; Abbasipour, M. Electrospinning as a Versatile Method for Fabricating Coreshell, Hollow and Porous Nanofibers. Sci. Iran. 2012, 19, 2029–2034. DOI: 10.1016/j.scient.2012.10.037.
- Liu, Q.; Yang, Q.; Zhou, Y.; Zhao, M.; Shen, Y.; Zhou, F.; Gong, R. H.; Deng, B. A Facile Method of Preparing Highly Porous Polylactide Microfibers. J. Appl. Polym. Sci. 2018, 135, 45860. DOI: 10.1002/app.45860.
- Huang, C.; Thomas, N. L. Fabrication of Porous Fibers via Electrospinning: Strategies and Applications. Polym. Rev. 2020, 60, 595–647. DOI: 10.1080/15583724.2019.1688830.
- Şimşek, M. Tuning Surface Texture of Electrospun Polycaprolactone Fibers: Effects of Solvent Systems and Relative Humidity. J. Mater. Res. 2020, 35, 332–342. DOI: 10.1557/jmr.2020.20.
- Casasola, R.; Thomas, N. L.; Trybala, A.; Georgiadou, S. Electrospun Poly Lactic Acid (PLA) Fibres: Effect of Different Solvent Systems on Fibre Morphology and Diameter. Polymer. 2014, 55, 4728–4737. DOI: 10.1016/j.polymer.2014.06.032.
- Huang, C.; Thomas, N. L. Fabricating Porous Poly(Lactic Acid) Fibres via Electrospinning. Eur. Polym. J. 2018, 99, 464–476. DOI: 10.1016/j.eurpolymj.2017.12.025.
- Tuzlakoglu, K.; Bolgen, N.; Salgado, A. J.; Gomes, M. E.; Piskin, E.; Reis, R. L. Nano- and Micro-Fiber Combined Scaffolds: A New Architecture for Bone Tissue Engineering. J. Mater. Sci. Mater. Med. 2005, 16, 1099–1104. DOI: 10.1007/S10856-005-4713-8.
- Cui, L.; Zhang, N.; Cui, W.; Zhang, P.; Chen, X. A Novel Nano/Micro-Fibrous Scaffold by Melt-Spinning Method for Bone Tissue Engineering. J. Bionic. Eng. 2015, 12, 117–128. DOI: 10.1016/S1672-6529(14)60106-2.
- Izzat Hassan, M.; Sultana, N.; Hamdan, S. Bioactivity Assessment of Poly(Caprolactone)/Hydroxyapatite Electrospun Fibers for Bone Tissue Engineering Application. J. Nanomater. 2014, 2014, 1–6. DOI: 10.1155/2014/573238.
- Bayrak, E.; Ozcan, B.; Erisken, C. Processing of Polycaprolactone and Hydroxyapatite to Fabricate Graded Electrospun Composites for Tendon-Bone Interface Regeneration. J. Polym. Eng. 2017, 37, 99–106. DOI: 10.1515/polyeng-2016-0017.
- Torres, E.; Dominguez-Candela, I.; Castello-Palacios, S.; Vallés-Lluch, A.; Fombuena, V. Development and Characterization of Polyester and Acrylate-Based Composites with Hydroxyapatite and Halloysite Nanotubes for Medical Applications. Polymers. 2020, 12, 1–13. DOI: 10.3390/POLYM12081703.
- Liu, L.; Lin, Z.; Niu, J.; Tian, D.; He, J. Electrospun Polysulfone/Poly(Lactic Acid) Nanoporous Fibrous Mats for Oil Removal from Water. Adsorpt. Sci. Technol 2019, 37, 438–450. DOI: 10.1177/0263617419828059.
- Zhou, X.; Lin, X.; White, K. L.; Lin, S.; Wu, H.; Cao, S.; Huang, L.; Chen, L. Effect of the Degree of Substitution on the Hydrophobicity of Acetylated Cellulose for Production of Liquid Marbles. Cellulose. 2016, 23, 811–821. DOI: 10.1007/S10570-015-0856-Z/FIGURES/7.
- Srikanth, M.; Asmatulu, R.; Cluff, K.; Yao, L. Material Characterization and Bioanalysis of Hybrid Scaffolds of Carbon Nanomaterial and Polymer Nanofibers. ACS. Omega. 2019, 4, 5044–5051. DOI: 10.1021/ACSOMEGA.9B00197/ASSET/IMAGES/ACSOMEGA.9B00197.SOCIAL.JPEG_V03.
- Shim, M. H.; Kim, J.; Park, C. H. The Effects of Surface Energy and Roughness on the Hydrophobicity of Woven Fabrics. Text. Res. J. 2014, 84, 1268–1278. DOI: 10.1177/0040517513495945.
- Chieng, B. W.; Ibrahim, N. A.; Yunus, W. M. Z. W.; Hussein, M. Z. Poly(Lactic Acid)/Poly(Ethylene Glycol) Polymer Nanocomposites: Effects of Graphene Nanoplatelets. Polymers. 2013, 6, 93–104. DOI: 10.3390/polym6010093.
- Hassanajili, S.; Karami-Pour, A.; Oryan, A.; Talaei-Khozani, T. Preparation and Characterization of PLA/PCL/HA Composite Scaffolds Using Indirect 3D Printing for Bone Tissue Engineering. Mater. Sci. Eng. C. Mater. Biol. Appl. 2019, 104, 109960. DOI: 10.1016/j.msec.2019.109960.
- D’Amato, A. R.; Schaub, N. J.; Cardenas, J. M.; Franz, E.; Rende, D.; Ziemba, A. M.; Gilbert, R. J. Evaluation of Procedures to Quantify Solvent Retention in Electrospun Fibers and Facilitate Solvent Removal. Fibers. Polym. 2017, 18, 483–492. DOI: 10.1007/s12221-017-1061-5.
- Ergul, N. M.; Unal, S.; Kartal, I.; Kalkandelen, C.; Ekren, N.; Kilic, O.; Chi-Chang, L.; Gunduz, O. 3D Printing of Chitosan/Poly(Vinyl Alcohol) Hydrogel Containing Synthesized Hydroxyapatite Scaffolds for Hard-Tissue Engineering. Polym. Test. 2019, 79, 106006. DOI: 10.1016/j.polymertesting.2019.106006.
- Mujahid, M.; Sarfraz, S.; Amin, S. On the Formation of Hydroxyapatite Nano Crystals Prepared Using Cationic Surfactant. Mat. Res. 2015, 18, 468–472. DOI: 10.1590/1516-1439.298014.
- Prajongtat, P.; Sriprachuabwong, C.; Wongkanya, R.; Dechtrirat, D.; Sudchanham, J.; Srisamran, N.; Sangthong, W.; Chuysinuan, P.; Tuantranont, A.; Hannongbua, S.; Chattham, N. Moisture-Resistant Electrospun Polymer Membranes for Efficient and Stable Fully Printable Perovskite Solar Cells Prepared in Humid Air. ACS Appl. Mater. Interfaces. 2019, 11, 27677–27685. DOI: 10.1021/ACSAMI.9B05032/SUPPL_FILE/AM9B05032_SI_001.PDF.
- Pandele, A. M.; Constantinescu, A.; Radu, I. C.; Miculescu, F.; Voicu, S. I.; Ciocan, L. T. Synthesis and Characterization of PLA-Micro-Structured Hydroxyapatite Composite Films. Materials. 2020, 13, 1–13. DOI: 10.3390/MA13020274.
- Turco, R.; Ortega-Toro, R.; Tesser, R.; Mallardo, S.; Collazo-Bigliardi, S.; Boix, A. C.; Malinconico, M.; Rippa, M.; Di Serio, M.; Santagata, G. Poly (Lactic Acid)/Thermoplastic Starch Films: Effect of Cardoon Seed Epoxidized Oil on Their Chemicophysical, Mechanical, and Barrier Properties. Coatings. 2019, 9, 574. DOI: 10.3390/coatings9090574.
- Motloung, M. P.; Mofokeng, T. G.; Ray, S. S. Viscoelastic, Thermal, and Mechanical Properties of Melt-Processed Poly (ε-Caprolactone) (PCL)/Hydroxyapatite (HAP) Composites. Materials. 2022, 15, 104. DOI: 10.3390/MA15010104.
- Mystiridou, E.; Patsidis, A. C.; Bouropoulos, N. Development and Characterization of 3D Printed Multifunctional Bioscaffolds Based on PLA/PCL/HAp/BaTiO3 Composites. Appl. Sci. 2021, 11, 4253. DOI: 10.3390/app11094253.
- Nguyen, H. T. H.; Qi, P.; Rostagno, M.; Feteha, A.; Miller, S. A. The Quest for High Glass Transition Temperature Bioplastics. J. Mater. Chem. A. 2018, 6, 9298–9331. DOI: 10.1039/C8TA00377G.
- de Moura, N. K.; Siqueira, I. A. W. B.; Machado, J. P. d B.; Kido, H. W.; Avanzi, I. R.; Rennó, A. C. M.; Trichês, E. d S.; Passador, F. R. Production and Characterization of Porous Polymeric Membranes of Pla/Pcl Blends with the Addition of Hydroxyapatite. J. Compos. Sci. 2019, 3, 45. DOI: 10.3390/jcs3020045.
- Akindoyo, J. O.; Beg, M. D. H.; Ghazali, S.; Heim, H. P.; Feldmann, M. Effects of Surface Modification on Dispersion, Mechanical, Thermal and Dynamic Mechanical Properties of Injection Molded PLA-Hydroxyapatite Composites. Compos. Part A Appl. Sci. Manuf. 2017, 103, 96–105. DOI: 10.1016/j.compositesa.2017.09.013.
- Zhao, L.; Cheng, S.; Liu, S.; Gao, X. Improvement of the Addition Amount and Dispersion of Hydroxyapatite in the Poly(Lactic Acid) Matrix by the Compatibilizer-Epoxidized Soybean Oil. J. Mater. Res. 2020, 35, 1523–1530. DOI: 10.1557/JMR.2020.116/TABLES/2.
- Athanasoulia, I. G. I.; Christoforidis, M. N.; Korres, D. M.; Tarantili, P. A. The Effect of Hydroxyapatite Nanoparticles on Crystallization and Thermomechanical Properties of PLLA Matrix. Pure. Appl. Chem. 2017, 89, 125–140. DOI: 10.1515/PAC-2016-0912/PDF.
- Dewey, M. J.; Harley, B. A. C. Biomaterial Design Strategies to Address Obstacles in Craniomaxillofacial Bone Repair. RSC Adv. 2021, 11, 17809–17827. DOI: 10.1039/D1RA02557K.
- Fu, S. Z.; Ni, P. Y.; Wang, B. Y.; Chu, B. Y.; Peng, J. R.; Zheng, L.; Zhao, X.; Luo, F.; Wei, Y. Q.; Qian, Z. Y. In Vivo Biocompatibility and Osteogenesis of Electrospun Poly(ε-Caprolactone)–Poly(Ethylene Glycol)–Poly(ε-Caprolactone)/Nano-Hydroxyapatite Composite Scaffold. Biomaterials. 2012, 33, 8363–8371. DOI: 10.1016/J.BIOMATERIALS.2012.08.023.
- Kim, T.; Lee, S.; Park, S. Y.; Chung, I. Biodegradable Pcl-b-Pla Microspheres with Nanopores Prepared via Raft Polymerization and Uv Photodegradation of Poly(Methyl Vinyl Ketone) Blocks. Polymers. 2021, 13, 3964. DOI: 10.3390/POLYM13223964/S1.
- Hausser, H. J.; Brenner, R. E. Phenotypic Instability of Saos-2 Cells in Long-Term Culture. Biochem. Biophys. Res. Commun. 2005, 333, 216–222. DOI: 10.1016/J.BBRC.2005.05.097.
- Berrier, A. L.; Yamada, K. M. Cell–Matrix Adhesion. J. Cell. Physiol. 2007, 213, 565–573. DOI: 10.1002/JCP.21237.
- Bacáková, L.; Filová, E.; Rypácek, F.; Svorcík, V.; Starý, V. Cell Adhesion on Artificial Materials for Tissue Engineering. Physiol. Res. 2004, 53, S35–S45.
- Paterlini, T. T.; Nogueira, L. F. B.; Tovani, C. B.; Cruz, M. A. E.; Derradi, R.; Ramos, A. P. The Role Played by Modified Bioinspired Surfaces in Interfacial Properties of Biomaterials. Biophys. Rev. 2017, 9, 683–698. DOI: 10.1007/s12551-017-0306-2.
- Menzies, K. L.; Jones, L. The Impact of Contact Angle on the Biocompatibility of Biomaterials. Optom. Vis. Sci. 2010, 87, 387–399. DOI: 10.1097/OPX.0B013E3181DA863E.
- Pivodova, V.; Frankova, J.; Dolezel, P.; Ulrichova, J. The Response of Osteoblast-like SaOS-2 Cells to Modified Titanium Surfaces. Int. J. Oral. Maxillofac. Implants. 2013, 28, 1386–1394. DOI: 10.11607/JOMI.3039.
- Zamani, F.; Amani-Tehran, M.; Latifi, M.; Shokrgozar, M. A. The Influence of Surface Nanoroughness of Electrospun PLGA Nanofibrous Scaffold on Nerve Cell Adhesion and Proliferation. J. Mater. Sci. Mater. Med. 2013, 24, 1551–1560. DOI: 10.1007/S10856-013-4905-6.
- Zhou, Q.; Xie, J.; Bao, M.; Yuan, H.; Ye, Z.; Lou, X.; Zhang, Y. Engineering Aligned Electrospun PLLA Microfibers with Nano-Porous Surface Nanotopography for Modulating the Responses of Vascular Smooth Muscle Cells. J. Mater. Chem. B. 2015, 3, 4439–4450. DOI: 10.1039/C5TB00051C.
- Yu, T.; Gleeson, S. E.; Li, C. Y.; Marcolongo, M. Electrospun Poly(ε-Caprolactone) Nanofiber Shish Kebabs Mimic Mineralized Bony Surface Features. J. Biomed. Mater. Res. B. Appl. Biomater. 2019, 107, 1141–1149. DOI: 10.1002/JBM.B.34207.
- Sabnis, R. W.; Deligeorgiev, T. G.; Jachak, M. N.; Dalvi, T. S. DiOC6(3): a Useful Dye for Staining the Endoplasmic Reticulum. Biotech. Histochem. 1997, 72, 253–258. DOI: 10.3109/10520299709082249.
- Ayobian-Markazi, N.; Fourootan, T.; Kharazifar, M. J. Comparison of Cell Viability and Morphology of a Human Osteoblast-like Cell Line (SaOS-2) Seeded on Various Bone Substitute Materials: An in Vitro Study. Dent. Res. J. 2012, 9, 86–92. DOI: 10.4103/1735-3327.92959.
- Yoshimoto, H.; Shin, Y. M.; Terai, H.; Vacanti, J. P. A Biodegradable Nanofiber Scaffold by Electrospinning and Its Potential for Bone Tissue Engineering. Biomaterials. 2003, 24, 2077–2082. DOI: 10.1016/S0142-9612(02)00635-X.
- Shin, M.; Yoshimoto, H.; Vacanti, J. P. In Vivo Bone Tissue Engineering Using Mesenchymal Stem Cells on a Novel Electrospun Nanofibrous Scaffold. Tissue. Eng. 2004, 10, 33–41. DOI: 10.1089/107632704322791673.
- Badami, A. S.; Kreke, M. R.; Thompson, M. S.; Riffle, J. S.; Goldstein, A. S. Effect of Fiber Diameter on Spreading, Proliferation, and Differentiation of Osteoblastic Cells on Electrospun Poly(Lactic Acid) Substrates. Biomaterials. 2006, 27, 596–606. DOI: 10.1016/J.BIOMATERIALS.2005.05.084.
- Chen, J.; Chu, B.; Hsiao, B. S. Mineralization of Hydroxyapatite in Electrospun Nanofibrous Poly(L-Lactic Acid) Scaffolds. J. Biomed. Mater. Res. A. 2006, 79, 307–317. DOI: 10.1002/JBM.A.30799.
- Yu, H. S.; Jang, J. H.; Kim, T. I.; Lee, H. H.; Kim, H. W. Apatite-Mineralized Polycaprolactone Nanofibrous Web as a Bone Tissue Regeneration Substrate. J. Biomed. Mater. Res. A. 2009, 88, 747–754. DOI: 10.1002/JBM.A.31709.
- Miyasako, H.; Yamamoto, K.; Aoyagi, T. Preparation, Characterization and Biocompatibility Study of the Scaffold Prototype Derived from Cross-Linked Poly[(ε-Caprolactone)-Co-Lactide] for Tissue Engineering Materials. Polym. J. 2008, 40, 806–812. DOI: 10.1295/polymj.PJ2008036.
- Pisani, S.; Genta, I.; Modena, T.; Dorati, R.; Bruni, G.; Benazzo, M.; Conti, B. A Proof of Concept to Define the Parameters Affecting Poly-l-Lactide-Co-Poly-ε-Caprolactone Shape Memory Electrospun Nanofibers for Biomedical Applications. Drug. Deliv. Transl. Res. 2023, 13, 593–607. DOI: 10.1007/S13346-022-01218-2/FIGURES/6.
- Vergroesen, P. P. A.; Kroeze, R. J.; Helder, M. N.; Smit, T. H. The Use of Poly(L-Lactide-Co-Caprolactone) as a Scaffold for Adipose Stem Cells in Bone Tissue Engineering: Application in a Spinal Fusion Model. Macromol. Biosci. 2011, 11, 722–730. DOI: 10.1002/MABI.201000433.
- Ma, R.; Guo, D. Evaluating the Bioactivity of a Hydroxyapatite-Incorporated Polyetheretherketone Biocomposite. J. Orthop. Surg. Res. 2019, 14, 32. DOI: 10.1186/s13018-019-1069-1.
- Lü, L. X.; Zhang, X. F.; Wang, Y. Y.; Ortiz, L.; Mao, X.; Jiang, Z. L.; Xiao, Z. D.; Huang, N. P. Effects of Hydroxyapatite-Containing Composite Nanofibers on Osteogenesis of Mesenchymal Stem Cells in Vitro and Bone Regeneration in Vivo. ACS Appl. Mater. Interfaces. 2013, 5, 319–330. DOI: 10.1021/AM302146W/SUPPL_FILE/AM302146W_SI_001.PDF.
- Liu, H.; Peng, H.; Wu, Y.; Zhang, C.; Cai, Y.; Xu, G.; Li, Q.; Chen, X.; Ji, J.; Zhang, Y.; OuYang, H. W. The Promotion of Bone Regeneration by Nanofibrous Hydroxyapatite/Chitosan Scaffolds by Effects on Integrin-BMP/Smad Signaling Pathway in BMSCs. Biomaterials. 2013, 34, 4404–4417. DOI: 10.1016/j.biomaterials.2013.02.048.
- Rouahi, M.; Champion, E.; Hardouin, P.; Anselme, K. Quantitative Kinetic Analysis of Gene Expression during Human Osteoblastic Adhesion on Orthopaedic Materials. Biomaterials. 2006, 27, 2829–2844. DOI: 10.1016/J.BIOMATERIALS.2006.01.001.
- Smith, I. O.; McCabe, L. R.; Baumann, M. J. MC3T3-E1 Osteoblast Attachment and Proliferation on Porous Hydroxyapatite Scaffolds Fabricated with Nanophase Powder. Int J Nanomedicine. 2006, 1, 189–194. DOI: 10.2147/NANO.2006.1.2.189.
- Kołodziejska, B.; Kaflak, A.; Kolmas, J. Biologically Inspired Collagen/Apatite Composite Biomaterials for Potential Use in Bone Tissue Regeneration-A Review. Mater. 2020, 13, 1748. DOI: 10.3390/MA13071748.
- Vagaská, B.; Bačáková, L.; Filová, E.; Balík, K. Osteogenic Cells on Bio-Inspired Materials for Bone Tissue Engineering. Physiol. Res. 2010, 59, 309–322. DOI: 10.33549/PHYSIOLRES.931776.
- Long, J. R.; Dindot, J. L.; Zebroski, H.; Kiihne, S.; Clark, R. H.; Campbell, A. A.; Stayton, P. S.; Drobny, G. P. A Peptide That Inhibits Hydroxyapatite Growth is in an Extended Conformation on the Crystal Surface. Proc. Natl. Acad. Sci. USA. 1998, 95, 12083–12087. DOI: 10.1073/PNAS.95.21.12083/ASSET/60DD21E9-D437-4838-B799-C98CA4866094/ASSETS/GRAPHIC/PQ2182888003.JPEG.
- Gorbunoff, M. J.; Timasheff, S. N. The Interaction of Proteins with Hydroxyapatite. III. Mechanism. Anal. Biochem. 1984, 136, 440–445. DOI: 10.1016/0003-2697(84)90241-0.
- Castner, D. G.; Ratner, B. D. Proteins Controlled with Precision at Organic, Polymeric, and Biopolymer Interfaces for Tissue Engineering and Regenerative Medicine. In Principles of Regenerative Medicine; Atala, A., Lanza, R., Mikos, A. G., Nerem, R., Eds.; Academic Press: Cambridge, 2019; pp.523–534. DOI: 10.1016/B978-0-12-809880-6.00031-X.
- Fu, C.; Bai, H.; Zhu, J.; Niu, Z.; Wang, Y.; Li, J.; Yang, X.; Bai, Y. Enhanced Cell Proliferation and Osteogenic Differentiation in Electrospun PLGA/Hydroxyapatite Nanofibre Scaffolds Incorporated with Graphene Oxide. PLoS One. 2017, 12, e0188352. DOI: 10.1371/JOURNAL.PONE.0188352.
- Prosecká, E.; Buzgo, M.; Rampichová, M.; Kocourek, T.; Kochová, P.; Vysloužilová, L.; Tvrdík, D.; Jelínek, M.; Lukáš, D.; Amler, E. Thin-Layer Hydroxyapatite Deposition on a Nanofiber Surface Stimulates Mesenchymal Stem Cell Proliferation and Their Differentiation into Osteoblasts. J. Biomed. Biotechnol. 2012, 2012, 428503. DOI: 10.1155/2012/428503.
- Guo, X.; Gough, J. E.; Xiao, P.; Liu, J.; Shen, Z. Fabrication of Nanostructured Hydroxyapatite and Analysis of Human Osteoblastic Cellular Response. J. Biomed. Mater. Res. A. 2007, 82, 1022–1032. DOI: 10.1002/JBM.A.31200.
- Shi, Z.; Huang, X.; Cai, Y.; Tang, R.; Yang, D. Size Effect of Hydroxyapatite Nanoparticles on Proliferation and Apoptosis of Osteoblast-like Cells. Acta. Biomater. 2009, 5, 338–345. DOI: 10.1016/J.ACTBIO.2008.07.023.
- Filová, E.; Suchý, T.; Sucharda, Z.; Šupová, M.; Žaloudková, M.; Balík, K.; Lisá, V.; Šlouf, M.; Bačáková, L. Support for the Initial Attachment, Growth and Differentiation of MG-63 Cells: A Comparison between Nano-Size Hydroxyapatite and Micro-Size Hydroxyapatite in Composites. Int. J. Nanomedicine. 2014, 9, 3687–3706. DOI: 10.2147/IJN.S56661.