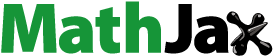
ABSTRACT
Adsorption has been traditionally modeled using equilibrium models like the Langmuir and the Freundlich isotherm models, and kinetic models such as pseudo-first and pseudo-second order equations. However, most of these equations are not in an Indepedant, and single-equation form. A novel mathematical framework that combines adsorption kinetics and isotherm equations to an independant, single-equation form is presented herin. The adsorption isotherm equations were first solved using the mass balance equations to derive an independant equation, eliminating the intermediate variables. This derivation was done for the commonly used adsorption isotherms such as Langmuir, Freundlich, Langmuir-Freundlich and Linear isotherms. The simplified equations can be used for predicting solid phase adsorption (qe) and aqueous phase concentration (Ce) in terms of equilibrium constants, and was called as Equilibrium Adsorption Solution Equation (EASE). This EASE equation was further integrated into adsorption kinetic equations to give a solution which can also predict kinetic adsorption. This framework was named as Combined Adsorption Kinetic and Equilibrium (CAKE) equation and was further validated with experimental data. The CAKE equation offers the following advantages: By using this combined equation, both the kinetics and equilibrium concentrations of the batch adsorption systems can be predicted. Further, the intermediate variables are eliminated, and the final equation involving only model constants such as maximum adsorption capacity (qmax), affinity constant (ka), initial concentration (Co), and kinetic rate constants (kt) are required to to calculate pollutant concentration at any given time. The model was validated using an experimental dataset of 2,4-Dichlorophenol adsorption studies on activated carbon. The CAKE and EASE models fitted the experimental datasets very well with an R2 of 0.85, and a normalized absolute percentage error (NAPE) of 7.5%., thus validating the developed equations.
1. Introduction
Adsorption is a convenientl approach which is commonly employed to treat various pollutants, such as organic and inorganic pollutants. The fundamental process in adsorption involves the use of high surface area adsorbents for the removal of contaminants. Some of the common adsorbents used for their good adsorptive properties are activated carbon[Citation1], montmorillonite,[Citation2] biochar,[Citation3] nanoparticles,[Citation4] and biomass[Citation5] etc. The literature on adsorption suggests the use of adsorption equilibrium Isotherms and Kinetic models for simulating the equilibrium of adsorption and the kinetics of the adsorption, respectively[Citation1,Citation6–8]
1.1. Equilibrium models
Adsorption equilibrium models such as Langmuir isotherm represent mathematical equations that describe the behavior of adsorbate molecules on the adsorbent surface.[Citation9–11] These isotherm models contribute to simulating the interaction occurring between the solute and adsorbant when the adsorption system is at equilibrium. Some of the popularly used isotherms are viz Langmuir, Freundlich, Temkin, Redlich Peterson model, etc[Citation12–15] , and are listed below in . Each model provides insights into the correlation among the adsorbate concentration (Co), surface coverage, and other appropriate parameters while basing its conclusions on specific adsorption process.[Citation16] The desired model to choose is dependant on the system, adsorption behavior and is at the discretion of the individual researcher. However, the choice of isotherm model is also based on experimental results and the individual adsorption system under consideration. In numerous scientific, industrial, and environmental applications, these isotherm models are necessary for the evaluation and optimization of adsorption processes. The following are some of the conventional adsorption equilibrium isotherm models, as listed in .
Table 1. Single and multiparameter adsorption isotherm models.
1.2. Kinetic models
Unlike adsorption isotherms as discussed in subsection 1.1, kinetic models are employed in unsteady state adsorption studies, when adsorption has not reached equilibirum, These models include the pseudo-first-order, pseudo-second-order, Elovich model, Intraparticle diffusion and Avrami model[Citation27–29] as listed in . The first two models, the Pseudo-first-order model and the Pseudo-second order model, listed in interpret the rate at which pollutants get transferred onto a solid phase and aid in recognizing physisorption/chemisorption. The third model (Avrami model) emphasizes multilayer adsorption kinetics with both physical and chemical processes and is specifically applicable to systems involving chemisorption and surface heterogeneity.[Citation34] The fourth model called the Elovich model, represents a two-step process involving chemisorption followed by a diffusion-controlled stage. The next model (Ritchies second-order model) is usually used to quantify initial particle loading.[Citation35] The subsequent models (Intraparticle diffusion and Boyd’s model) focus on the transport of adsorbate molecules within the pores of the adsorbent, offering insights into diffusion as a rate-controlling step. In addition, the Weber-Morris model sheds light on the role and significance of intraparticle diffusion in the overall adsorption process.[Citation33] Kinetic models play a crucial role in understanding and optimizing adsorption dynamics, aiding in effectively removing organic pollutants from diverse environmental and industrial contexts. Researchers often utilize a combination of these models to capture the complex kinetics and design efficient adsorption systems. In the following subsection 1.3 specifics of kinetic-equilibrium models are discussed.
Table 2. Different adsorption kinetic models and their significance.
1.3. Kinetic-equilibrium models
After a thorough examination of literature, it was possible to identify a few of the investigations wherein kinetic adsorption equation forms were investigated from isotherms such as Langmuir and Freundlich. For example, Liu and their team examined empirical kinetics equation forms, that is, first and second-order kinetic Langmuir kinetics (LK) by providing adequate conditions that could simplify LK to PFO and PSO. The analysis also emphasized that the simplification was a function of initial concentration (Co).[Citation36] Further, an integrated-order rate equation was proposed to describe the kinetics of the adsorption of methylene blue on carbon behavior of the adsorption activity. The study reported that mixed form/order of kinetic equation could illustrate the kinetics data better as compared to the existing rate equations.[Citation37] In continuation of the previous study, Marczewski derived and analyzed integrated kinetic Langmuir equation (IKL). They provided a complete analytical solution of the Langmuir kinetic equation and compared it with mixed order rate equations. The study also examined the impact of new terms equilibrium coverage (θqc) and relative equilibrium uptake (ueq) and could determine the adsorption behavior from the product of equilibrium coverage and relative equilibrium uptake, that is, Langmuir batch equilibrium factor (feq). In addition, the analysis and comparison of the new model (IKL) with the nth order, mixed 1,2-order, and multi-exponential allows one to understand fully the Langmuir kinetics. Further, the analysis yielded a similar expression as that of pseudo-first-order (PFO) and pseudo-second-order (PSO) kinetic equations. The expression of IKL was also extended to include some governing parameters, such as lateral interactions and heterogeneity.[Citation38] The reported equation exhibited good agreement with experimental data as well.[Citation39] On the other hand, 8 new models (Langmuir Kinetic) especially for homogenous adsorption were reported. These models were capable of illustrating the kinetic and adsorption behavior of varied adsorbents and were found to be more generalized as compared to existing ones. Further, the models could also quantify the maximum adsorption capacity (mg/g).[Citation40] Further, four unique models that can describe two-rate adsorption which are solely based on realistic processes such as adsorbing of two-species, two-sites with the difference in binding energy, re-arrangement, and bilayer adsorption modes was investigated. The said models could realistically account for systems influenced by either mass transport or activation limitations or the transition between the two. These models had practical applications in elucidating the adsorption kinetics of surfactants, proteins, and biomolecular systems, especially in cases involving surfaces with both hydrophilic and hydrophobic sites.[Citation41] An equation was derived on modification of Langmuir isotherm for adsorption processes. Here, the rate is controlled by diffusive transport phenomena referred to as Diffusion-Controlled Langmuir Kinetics (DCLK), assuming that the macroscopic forward rate of adsorption is inversely related to the square root of time. A new study by Salvestrini reported a “factorized differential Langmuir rate” form. The study examined different conditions under which the differential form would reduce to either the PFO or PFO kinetic model. They also proposed a graphical method and evaluated it with experimental data. From their investigation, the authors were also able to appropriately express the equation derived by[Citation38] as a function of time (t), initial pollutant concentration (Co), adsorbent dosage (m), adsorption rate constants (k1 or k2), adsorption capacity (qmax).[Citation42] In another research, the authors[Citation43] improvised the conventional rate (PFO/PSO) and Vermeulen equation by introducing a new parameter, that is, fractional surface coverage (θ) (fractal-like approach) for heterogeneous surfaces. The study also proposed a separation factor (RH) primarily to describe fractal-like Langmuir form. To validate the proposed expression/model, data on nitrate adsorption on PAN/AC was used. The stated outcomes showed an accurate estimation of equilibrium time by modified PSO (R2=.998). The improvisation of the previously developed equation[Citation42] was further reported and was called as “diffusion-controlled Langmuir kinetics” (DCLK) model. The model worked on the assumption that forward adsorption rate as an inverse function of time1/2 (t1/2) was assumed. The model could better describe the kinetics of adsorption as compared to the existing models. Further, the kinetics (PFO/PSO) using Statistical Rate Theory (SRT) at close to equilibrium condition (Co>> Ce and θe≈1) was explored. The model could also prove that fractional coverage is a linear function of t1/2 for initial adsorption.[Citation44] Unlike the investigation by[Citation40] on homogenous adsorption, modified kinetic equation for a solid-liquid interface assumes a heterogeneous chemical reaction. Considering this equation as a base, a working equation was also developed which was able to provide an independent variable, that is, adsorption rate constants. The investigation could also distinguish between PFO and PSO based on three dimensionless numbers.[Citation45] Further, a general kinetic model called as mixed order model (MO) was developed by[Citation46] which was able to describe the whole adsorption process with accuracy. In addition, the MO model was able to describe both the PFO and PSO kinetic of any given adsorption system. The above previous studies have limitation due to the complexity of the methods used (by using integral or differential methods mainly with Langmuir isotherm as base form).
1.4. Research gap
The Scopus survey tool was used to determine the scope of adsorption and kinetic equations.[Citation47] The Scopus survey for the keywords “Isotherm” AND “Kinetic” AND “Adsorption” displayed a total of 36,026 published articles in 50 years (from 1976–2024). Firstly, from , it can be observed that there was a continuous exponential rise in publications between the years 2004 to 2024 (from <100 articles per year to nearly 3600 articles per year). displays the list of domains in which adsorption, isotherm and kinetics are extensively used which was ≈ 75% (except red colored portion). Thus, it means, most of the adsorption studies involve the implication of either isotherm or kinetic model separately.
Figure 1: Scopus survey of publications in (a) adsorption domain (b) subject wise publication in adsorption using isotherm and kinetics models.
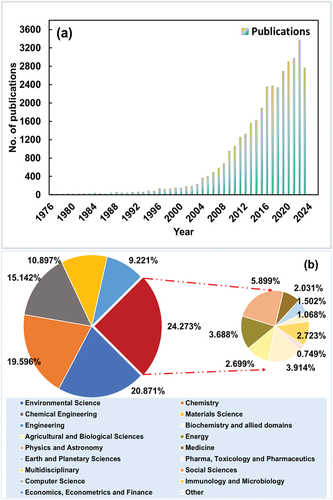
Second, from Subsection 1.3 (literature), it is evident that there are minimal studies where combined kinetics and equilibrium approaches were undertaken. There is insufficient readily available compilation of combined kinetic and equilibrium models which could aid in quantifying qmax, te, Ct. Consequently, it is clear that there is considerable need for such research work.
Thirdly, keeping in mind the practical applicability in treatment systems of large-scale, it is not necessary to carry out the adsorption process till equilibrium duration. Monitoring the remaining concentration and determining equilibrium time is a prolonged process. Therefore, when an adsorbent is prepared/commercially available, we need to know the efficiency of that adsorbent and the adsorption capacity for a particular pollutant (say 2,4-DCP) for a limited amount of time, especially in continuous processes. For this purpose readily available, simplified and solved equations are limited.
Thus, there is a need for combined adsorption kinetic equation using isotherm (Langmuir, Freundlich, Langmuir-Freundlich), which can estimate,
Equilibrium adsorption capacity (qmax) and the respective concentration (Ct) are not available.
Saturation/equilibrium time (te)
With this fundamental research gap in mind, we proposed the CAKE approach herein, which can forecast the adsorption (qt) at any given point of time (t), for any initial concentration (Co) of the pollutant for a known volume (V) of the system and known mass of adsorbent (m).
The objectives of the present investigation were to (i) Develop a simplified solution for adsorption equilibrium equation using isotherm equations and mass balance. The equation was called Equilibrium Adsorption Solution Equation (EASE) and (ii) Combine the equilibrium EASE form with kinetic equations (PFO/PSO) to develop the combined kinetic-equilibrium equations labeled as CAKE equation (iii) Validate the developed CAKE and EASE equations with experimental data.
2. Development of models
2.1. Derivation of combined adsorption kinetic equilibrium models
Before proceeding to the derivation there are some of the assumptions which are used in the equations. Assumptions: (a) The rate of adsorption reactions (k1, k2) would remain constant for all the concentrations. (b) Temperature of the closed system is constant. (c) Equilibrium is reached for the isotherm experiment within the specified equilibrium time. (d) No chemical reaction is occurring in the system other than the adsorption.
For Langmuir isotherm, the derivation follows these steps:
Initially, isotherm equations were used to arrive at the equilibrium concentration Ce.
Further, the obtained Ce was substituted in the equation representing experimental adsorption and simplified.
The simplified equation is solved for qe.
Similar steps were also taken by considering Freundlich isotherm and Langmuir-Freundlich isotherm and were substituted in the experimental adsorption capacity equation and solved for qe for all the cases. The derivation of all the CAKE models is presented in the following sub-sections.
2.2. Derivation of combined isotherm-kinetic models for Langmuirs isotherm
2.2.1. Combining Langmuir and pseudo first/second order models
For the Langmuir adsorption isotherm, the expression for adsorption capacity is given by Eq. 1
Solving for Ce gives,
Mass balance suggests that
We have an expression for adsorption capacity from mass balance, as shown below in Eq. 3
Solving for Co gives,
Substituting value Ce from Eq. 2 in Eq. 4
Simplifying further,
Rewriting Eq. 6 in the form.
ax2 + bx + c = 0
We get,
Solving for from Eq. 7 by using quadratic equation solution as below,
The solution of Eq. 7 is as given below.
Further simplifying Eq. 8, we get
Eq. 9 is called Equilibrium Adsorption Solution Equation-Langmuir (EASE-L).
We can also determine equation for equilibrium concentration by substituting “qe” in Eq. 4 with Eq. 9
Therefore, Eq. 10 can be labeled as Equilibrium Concentration derived from Langmuir model (EC-L)
The kinetic pseudo first order (PFO) equation is expressed as in Eq. 11
Substituting EASE-L from Eq. 9 in Eq. 11, we get the combined adsorption kinetic-equilibrium (CAKE) equation for pseudo-first -order kinetics as
From Eq. 12, the concentration at time “t” can be given as below.
The equation for PSO is expressed as in Eq. 14,
Substituting qe from Eq. 9 in Eq. 15, we get the CAKE equation for Langmuir-PSO
Similarly, concentration at time “t” can be obtained for PSO,
Eq. 12 and Eq. 15 are CAKE equations for PFO and PSO as obtained from Langmuir Isotherm, respectively.
2.3. Freundlich isotherm: derivation of CAKE and EASE equations
2.3.1. Combining Freundlich and pseudo First/Second order models
Similar derivation can be done for Freundlich isotherm equation, which is given by the expression Eq. 17
Taking the logarithm function on both sides of Eq. 17
Solving for Ce, we get
Substituting value Ce from Eq. 18 in Eq. 17
For fractional n, we can make “n” close to a nearest whole number and calculate Kf,
For “n” =1 in the Eq. 19 we get,
Therefore, Eq. 19 changes and rearranged as below,
For “n” = 1, qe is given by
Or
Similarly, substituting “n”=2 in the above Eq. 19 we get
Eq. 20 is in the quadratic form and the solution for qe is shown below,
Now, substituting EASE-F from Eq. 21 in Eq. 11 and Eq. 14
The Eq. 22 is CAKE form for PFO.
Similarly, for PSO another equation (Eq. 23) can be obtained as below,
Eq. 22 and Eq. 23 are CAKE equations (PFO and PSO) as obtained from Freundlich Isotherm for the quadratic form of Eq. 20.
Now, substituting n=3 in the above Eq. 19 we get
Eq. 24 is cubic nature and the solution for qe can be given as below,
Eq. 21 and Eq. 25 are called as Equilibrium Adsorption Solution Equation-Freundlich (EASE-F).
Eq. 26 is Equilibrium Concentration derived from Freundlich model (EC-F). Similarly, we have CAKE equations for the cubic form of Eq. 24 as given below,
Similarly, we have CAKE equations for the cubic form of eq. 24 as given in eq. 27 and eq. 29
2.4. Langmuir-Freundlich (Sips isotherm)
2.4.1. Combining Langmuir-freundlich and pseudo First/Second order models
Similar derivation can be done for Langmuir-Freundlich isotherm, which is given by Eq. 31
The EASE equation can be written as
Eq. 32 can be labeled as Equilibrium Adsorption Solution Equation-Langmuir-Freundlich (EASE-LF). In addition to this, Equilibrium concentration derived from Langmuir-Freundlich model (EC-LF) can be represented as given below in Eq. 33.
Further, the above forms can be used in PFO and PSO equations.
Eq. 34 and Eq. 36 are the CAKE (PFO and PSO) equations obtained from LF Isotherm.
2.5. Linear isotherm/Henry’s equation
2.5.1. Combining linear isotherm with pseudo First-/Second-order models
At low concentrations, the Linear isotherm (Henry’s equation), can be used and is given by,
Or
Eq 38 is the EASE form for the Henry’s isotherm, and corresponding EC eqn is given above, Substituting the qe term in Eq. 11 and Eq. 14 we get,
Or
Where, is apparent distribution coefficient for kinetic problems in linear isotherm.
Also solving for Ct gives
Similarly, solving for qt gives
And Ct is given for pseudo-second-order kinetics by following equation,
Eq. 40 is CAKE form for pseudo-second-order kinetics.
Eq. 39 and Eq. 40 are modified CAKE equations (PFO and PSO) as obtained from the Linear/Henry form of the isotherm.
Similarly, Ct is given by
At high concentrations (saturation range), is given by the derivation below, we can approximate as, qe can be approximated as qm
For pseudo-first-order kinetics
For pseudo-second-order kinetics,
provides a summary and comprehensive list of all CAKE models for qe or solid phase adsorption at equilibrium , while provides equations governing equilibrium aqueous concentration (Ce). Furthermore presents, equations to determine the concentration (Ct) at a specific time (t) in batch adsorption studies.
Table 3. List of all the kinetic and equilibrium models.
Table 4. List of equations for Ce the equilibrium concentration (EC equations derived from EASE).
Table 5. List of equations for Ct (concentration at desired time) derived from CAKE models.
2.6. Error functions
Different error functions, that is, the sum of squares error (SSE),[Citation48] mean absolute percentage error (MAPE) and normalized average percentage error (NAPE) were used in the present investigation for evaluating the models. The purpose of using these error functions was to determine the best fit between experimental (qexp) and model (qmod) data (in the Freundlich-PFO/PSO model). The lower the value of SSE, MAPE and NAPE,[Citation49] the higher the accuracy of the model. The expressions for the error functions are given below,
3. Materials and methods
3.1. Preparation of adsorbate
The experimental study used analytical grade 2,4-dichlorphenol (C6H4OCl2), orthophosphoric acid (H3PO4), hydrochloric acid (HCl) and sodium bicarbonate (NaOH). To prepare 1000 mg/L of solution, 1g of 2,4-dichlorophenol (2,4-DCP) was added to 1000 ml of distilled water and stirred well. The desired concentration was further prepared by diluting the stock solution. The concentration of the adsorbate solution was measured in a UV-Vis spectrophotometer at a wavelength (λ) of 284 nm.[Citation4]
3.2. Preparation of adsorbent
An agricultural waste, Cassia fistula pod (CFP) shell was used as a precursor of activated carbon. The shell was separated, washed, and dried under the sun. Further, the dried shells were pulverized and sieved through 425 microns. The fine particles were then collected and mixed with H3PO4 in equal ratios (w/v) and allowed overnight. The impregnated material was carbonized for 60 minutes in muffle furnace at 500°C in the presence of nitrogen supply (inert atmosphere). The carbonized material/activated carbon was cooled, washed using distilled water, dried and further used for adsorption studies labeled as Cassia fistula pod activated carbon (CFPAC).
3.3. Optimization of operational parameters
Batch experiments were performed to find the best conditions. The parameters such as initial concentration (25-500mg/L), dosage (0.2g/L-4g/L), temperature (20–50°C) and agitation speed (50-200rpm) were considered for the optimization. The pH (2–12) of the solution was adjusted using standard acid and base solution of HCl and NaOH (0.1N-1N).
Initially, the experiments were conducted in a 250 mL standard conical flask with a stopper. A working volume (V) of 50 ml for varied initial concentrations (Co) as mentioned above, an adsorbent dosage(m) of 50 mg, working speed of 150rpm, and temperature at 30°C in a thermostat till equilibrium was considered. Once equilibrium was attained the samples were collected in clean, dry vials. Further, samples are centrifuged to reduce interference of particles in the sample and analyzed using a UV-visible spectrophotometer (Shimadzu, Japan).
3.4. Isotherm study and model fit
The isotherm studies were performed in batch mode at optimized conditions for different initial concentrations ranging from 25-500mg/L till equilibrium time (2 hours). The experimental data were further fitted to isotherm models such as Langmuir isotherm,[Citation10] Freundlich isotherm,[Citation50] and LF-model[Citation51] as listed in . Furthermore, the error functions are also calculated to identify the best isotherm model.
3.5. Kinetic study and model fit
In kinetic studies, samples were collected and analyzed at different intervals of time until equilibrium is achieved.[Citation18] The data obtained from kinetic studies were modeled using linear and non-linear forms of equations.[Citation9] The details of the kinetic models used for the study are listed in .
3.6. Non-linear optimization
Microsoft Excel solver was used for non-linear optimization by minimizing the errors. GRG non-linear solution method was used to minimize the sum of squares of errors.
4. Experimental results
4.1. Equilibrium studies of 2,4-DCP adsorption
The experimental data of adsorption of 2,4-DCP was fitted to different isotherm models to obtain different isotherm parameters (qm, kL, kf, 1/n, kg, nL). The below shows plots of different isotherms considered for the study.
Figure 2: Plot of various isotherms for the adsorption of 2,4-DCP.
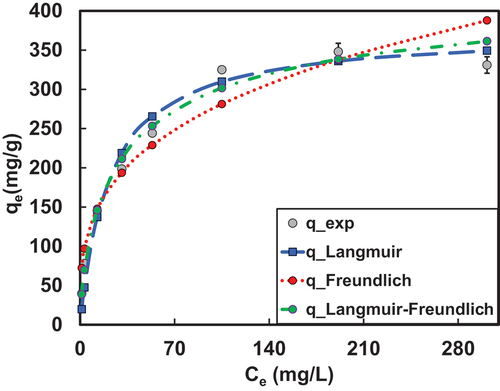
The graphs depict that, Langmuir-Freundlich model is better fitted with experimental data. The value of nL approaching unity which means monolayer adsorption is dominating the adsorption of 2,4-DCP.[Citation52] shows plots of comparison of model values with experimental data. The details of error values of different EASE models are listed .
Figure 3: Comparison of experimental results with new EASE models.
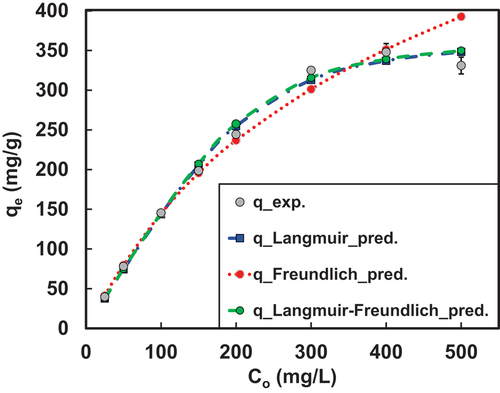
Table 6. Comparison of error values of all EASE models.
The EASE equation could provide a good fit as observed in . The order of fit was found to be EASE-LF>EASE-L>EASE-F which was exclusively based on all the error functions listed in subsection 2.3.
4.2. Application of CAKE model for 2,4-DCP adsorption
As mentioned in the methodology, data pertaining to isotherms and kinetics experiments were obtained for adsorption of 2,4-DCP on Cassia fistula (CF) pods derived activated carbon (CFPAC). The model parameters for the present investigation are listed in . The obtained experimental plots are compared with the modeled values. The comparison was based on values of R2 and mean absolute percentage error (MAPE).
Table 7. Summary of model parameters (Isotherm & kinetic) used in the study.
4.2.1. Langmuir CAKE model (PFO and PSO)
shows the adsorption kinetics of 2,4-DCP onto CFPAC at different initial solute concentrations. The graph also incorporates a comparison of experimental data with CAKE Langmuir models (PFO and PSO) values. From , it can be visualized that, the equilibrium time as obtained from the new kinetic Langmuir model i.e., Eq. 12 for lower concentrations say 25ppm and 50ppm was approximately 20mins, which was similar to the experimental values. The experimental values were forecasted well by the CAKE equations, irrespective of the concentration chosen. Further, mean absolute percentage error (MAPE) was found to vary from 5–7%. Similarly, from , the predicted equilibrium time was also 20mins as found after applying Eq. 15. In this case, the value of MAPE varied from 6–10%. Based on the error %, it could be concluded that the model fit was better for Langmuir-PFO compared to Langmuir-PSO. The dotted portion of the graph indicates experimental data and continuous lines indicate modeled data.
4.2.2. Freundlich CAKE model (PFO and PSO)
,(b) demonstrates the plot of adsorption kinetics. In addition, the graph incorporates a comparison of experimental (PFO and PSO) with the new model i.e., the Freundlich CAKE model. It can be visualized that the equilibrium time as obtained from the new kinetic model i.e., Eq. 27 for all the concentrations was approximately 20mins which was in congruence with the experimental values. The modified Freundlich-PFO is given below. However, it was possible to fit experimental data with the least MAPE ranging 4–7% from the new model using different n and kf values. Further, in the case of Freundlich-PSO (Eq. 29), the equilibrium time was approximately 30mins. The average % error varied from 5–6.5% indicating a good fit for lower concentrations. The R2PSO was found to be greater as compared to R2PFO. Thus, the model fit was better for Freundlich-PSO. The dotted portion of the graph indicates experimental data and continuous lines indicate predicted data.
4.2.3. Langmuir-freundlich CAKE model (PFO and PSO)
denotes the chart of experimental and predicted values using the new model i.e., LF-kinetic model. In the case of LF-PFO , it can be observed that the equilibrium time achieved by using i.e., Eq. 34 was nearly 20mins for all the concentrations. An early equilibrium in 15min was observed in the computed time similar to the experimental values. The interesting part here was it was possible to get the best fit with the least % average error in the range of 6–9% for the new model for the same constant (nL and kg) and the lowest NAPE value ranging from 2.99% to 7.4%. Further, in in the case of LF-PSO using Eq. 36, an equilibrium time of 25mins was observed. The deviation of 5 mins w.r.t experimental data was observed.
The NAPE of 4.102 and 7.39% was obtained with PFO and PSO, respectively. Thus, indicating that the LF-PSO model fit was better for FL-PSO. The error values for different CAKE models are listed in . The dotted portion of the graph indicates experimental data and continuous lines indicate predicted data.
Table 8. Error values for different models.
5. Discussion
Our literature survey showed that both the Langmuir model and the Freundlich model are the extensively employed isotherm models in more than 95% cases.[Citation18,Citation39] Moreover, for simulating adsorption kinetics, the pseudo-first order and pseudo-second order models are frequently employed. However, there are limited equations combining the isotherms and kinetics.
A new kinetic-equilibrium modeling approach has been presented in this research article, wherein, the adsorption isotherm model and kinetic model are linked by following simple mathematical steps (see Subsection 2.2). This linkage was done for Langmuir model,[Citation36,Citation53] Freundlich and LF-models. The advantage of the developed CAKE and EASE models is that a single set of parameters can be used for both types of models, isotherms and kinetics unlike earlier investigations.[Citation42,Citation45,Citation46]
Earlier investigations have proposed an integrated kinetic Langmuir (IKL) equation to describe the typical kinetic behavior of the adsorption activity.[Citation38] Further, in another research, the conventional rate equations were improvised by introducing a new parameter (θ) called fractional surface coverage,[Citation43] which is difficult to measure directly. Also, the preceding investigations lack ease of usability (e.g. Langmuir rate equation’s solution[Citation36]). In some cases, it is difficult to determine fraction surface coverage at a given time. The absence of a clear single equation has made it challenging to use the adsorption models in chemical industries. Most chemical industries mainly operate in the kinetic regime. In such cases intermediate parameters such as qe are difficult to determine.
For example, the Pseudo-second order equation is given by,
But qe is not known either; hence the determination of qt becomes hard.
“qe” can be determined using the isotherm equation as above. However, again the problem is that Ce is not known. In such cases, the value of qe cannot be found since Ce is not available readily and has to be measured. This is similar to chicken and egg? which came first puzzle. To disentangle this puzzle, we have distinctly solved for qe in terms of constants such as qm, V, kt, and kL, which are constants which need to be determined only once. Thus, the measurement of Ce for every Co is eliminated. Thus, the listed equations (as mentioned in ) have been formulated to enhance their practical applicability. By presenting these equations in an independant, clear and usable formr, we aim to facilitate their seamless utilization in various chemical processes and applications aspects.[Citation36,Citation41,Citation44]
Also in this article, an experimental study on the adsorption of 2,4-DCP on CFPAC was conducted and utilized for validation of the derived equations. In the kinetic investigation, the PSO model gave a better fit than the PFO model. When considering CAKE models, the Langmuir-PFO and Langmuir-PSO models gave the best overall fits to experimental data (). This suggests that the behavior was single-layer homogenous adsorption. The model’s superiority was in the order, of LF-PSO>Langmuir-PFO> LF-PFO > Langmuir-PSO> Freundlich-PSO > Freundlich-PFO. The better fit of LF-PSO was credited to the fact that the LF equation represents an improvement over the conventional Langmuir and Freundlich models. This was due to the presence of additional heterogeneity term in LF model. Freundlich-PFO isotherm gave highest errors, which suggests that isotherm did not follow the Freundlich model, and a mono-layer adsorption was likely.
A linear isotherm CAKE equation was also developed. This is particularly useful at low concentrations. Concentrations of contaminants in groundwater usually are low levels, where use of linear isotherm is warranted. This form of CAKE and EASE can be used for problems in several other domains where pollutants are at ppb levels.
The combined adsorption Kinetics and Equilibrium (CAKE) approach showed the clear failure of Freundlich models, which is not visible in Equilibrium Freundlich isotherms alone. An early equilibrium was observed for this experiemntal system in the models, and the equilibirum time was about 20 minutes. Thus, the CAKE models can give a better clarity on the proper choice of adsorption isotherms, which are suited for kinetic and equilibrium scenarios. The MAPE and NAPE error analysis was performed to determine the goodness of fit of the CAKE modeling framework (). The CAKE approach would be especially useful for industries and field-scale applications where kinetics processes predominate. This is because CAKE gives a single equation which can be incorporated into software like MS Excel to easily give predictions at desired times. The use of a single equation also makes it usable in various other contaminant transport softwares as well.
6. Conclusions
To summarize, this study has introduced a novel analytical modeling framework designated as Combined Adsorption Kinetic and Equilibrium (CAKE) and Equilibrium Adsorption Solution Equation (EASE). The equations were formulated through the utilization of existing adsorption isotherm, mass balance and kinetic rate equations, specifically PFO/PSO. The incorporation of various isotherms, including Langmuir, Freundlich, and LF into the kinetic models, enhances the usefulness of the modeling framework. The obtained models were used to estimate equilibrium concentration (Ct), equilibirum time (te) and adsorption capacity (qt) at any time. The CAKE models estimated te= 20mins for the concentration 25ppm and 50ppm, which was in congruence with our experimental data. Notably, the LF-PFO equation emerges as the most effective model in portraying the adsorption kinetics of 2,4-DCP onto CFPAC, based on higher values of R2. The CAKE models also gave a better clarity on the proper choice of adsorption isotherms and suggested that the Freundlich model did not suit the data relatively well. The exact selection of a suitable model proves pivotal for accurately estimating both the equilibrium time (te) and adsorption capacity (qmax). Ranking the order of fit, LF-PSO surpasses other models, followed by Langmuir-PFO, LF-PFO, Langmuir-PSO, Freundlich-PSO, and Freundlich-PFO. The superiority of LF-PSO was attributed to the fact that the LF equation represents an improvement over the conventional Langmuir and Freundlich models. Also, error analysis showed that the CAKE and EASE models demonstrated lower errors, as quantified by mean absolute percentage error (MAPE) of 8.5%, Normalized absolute percentage error (NAPE) of 7.5% and R2 of 0.85.
The development of these computational models carries substantial practical implications for the chemical industry. For instance, these models can help optimize operations by reducing the time, chemicals and energy , as the predictions can be made for kinetic adsorption processes for qt and Ct (). Also, the mass of adsorbent required can be minimized by predicting adsorption capacity qt () ,thus saving the excess usage of adsorbent. This not only improves efficiency but also has the potential to save costs and enhance the overall usability of adsorption processes. Ultimately, this work serves as a valuable contribution to the field of adsorption, offering more accurate and efficient methods for modeling and predicting adsorption behavior.
For the future prospects, researchers can adopt the developed CAKE and EASE models to simulate adsorption of pollutants in field scale wastewater treatment systems. Furthermore, one can formulate combined equations by considering other isotherm models and kinetic equations. Also, artificial intelligence and machine learning systems can be developed with the experimental data and predictions from CAKE equations.
Nomenclature
= | Equilibrium adsorption capacity of adsorbents, (mg/g) | |
= | Equilibrium concentration of adsorbates, (mg/L) | |
= | Initial concentration of adsorbates, (mg/L) | |
= | Henry constant (L/g) | |
qm | = | Maximum adsorption capacity of adsorbent, (mg/L) |
kL | = | Langmuir constant, (L/mg) |
= | Freundlich constant, (L/mg) | |
n | = | Magnitude of adsorption driving force |
AT | = | Heat of adsorption (kJ/mol) |
bT | = | Equilibrium binding energy (J/mol) |
Ks, | = | Sips equilibrium constant (L/g) |
βs | = | Sips model exponent (unitless) |
qmS | = | Sips maximum adsorption capacity (mg/g) |
Cs | = | Saturated solute concentration (mg/L) |
z | = | Factor of heterogeneity |
B, C | = | Interaction with the surface pertaining to BET constant |
KT | = | Toth Equilibrium constant, (L/mg) |
aT | = | Affinity co-efficient dependent on temperature (L/mg) |
t | = | Toth constant (Heterogeneity of adsorption) |
kRP | = | Redlich peterson constant (L/g) |
∝RP | = | Redlich Peterson constants (L/g)β |
= | Redlich Peterson exponential constant | |
bk | = | Khan isotherm model constant (unitless) |
ak | = | Khan isotherm exponent (unitless) |
qs | = | Khan isotherm maximum adsorption capacity (mg/g) |
ka | = | Affinity constant (unitless) |
pH | = | pH of solution |
n1 | = | Index of heterogeneity (unitless) |
kg | = | Affinity co-efficient for adsorption, (L/mg) |
qt | = | Adsorption capacity at time “t” |
qe | = | Adsorption capacity at equilibrium (mg/g) |
k1 | = | Pseudo first-order rate constant (min−1) |
k2 | = | Pseudo-second-order rate constant (g/mg/min) |
kAV | = | Avrami Kinetic constant (min−1) |
nAV | = | Avrami exponent (unitless) |
kpi | = | Diffusion constant (mg/g min) |
Ci | = | Thickness of boundary layer (mg/g) |
a | = | Initial adsorption rate (mg/g/min−1) |
βt | = | Desorption constant (g/mg) |
qexp | = | Experimental adsorption capacity (mg/g) |
qmod | = | Model adsorption capacity (mg/g) |
V | = | Volume of solution (mg/g) |
m | = | Dosage of adsorbent (mg) |
r | = | Rate coefficient (min−1) |
∝ | = | nth order rate constant (min−1) |
q∞ | = | Equilibrium adsorption capacity at infinite time (mg/g) |
ns | = | Active sites occupied by an adsorbate |
Authors contributions
Praveengouda Patil: Conceptualization, methodology, Investigation, Data curation, Validation, Formal analysis, modeling.
Bhojaraja Mohan: Data collection, survey, methodology, and investigation.
Gautham Jeppu: Project administration, Conceptualization, Validation, Resources, Supervision, review & editing.
Chikmagalur Raju Girish: Project administration, Validation, Resources, Supervision, review & editing.
Availability of data and materials
All the data are available and can be provided to the editorial team on request.
Consent to Publish
We declare that there is no conflict of interest associated with this manuscript. As the corresponding author, we confirm that the manuscript has been proofread and approved for submission by all named authors.
Mr. Praveengouda Patil
Mr. Bhojaraja Mohan
Dr. Gautham Jeppu
Dr. Chikmagalur Raju Girish (corresponding author)
Novelty Statement
1. A new modeling framework connecting kinetic and equilibrium equations called the Combined Adsorption Kinetic and Equilibrium (CAKE) approach for adsorption kinetics and adsorption equilibrium is developed.
2. Various forms of CAKE equations are developed by considering different combinations of kinetic and equilibrium models, such as Langmuir, Freundlich, Langmuir-Freundlich and Linear isotherms.
3. Isotherm and Kinetic constants are incorporated into the above-developed forms and the adsorption capacity (qt), concentration (Ct) at a given time were predicted for an experimental dataset of the 2,4-Dichlorophenol adsorption system, thus validating the models.
Disclosure statement
No potential conflict of interest was reported by the author(s).
Additional information
Funding
References
- Sathishkumar, M., et al. Adsorption Potential of Maize Cob Carbon for 2,4-dichlorophenol Removal from Aqueous Solutions: Equilibrium, Kinetics and Thermodynamics Modeling. Chem. Eng. J. 2009, 147(2–3), 265–271. DOI: 10.1016/j.cej.2008.07.020.
- Wu, C. D.; Wang, L.; Hu, C. X.; He, M. H. Single-solute and Bisolute Sorption of Phenol and Trichloroethylene from Aqueous Solution onto Modified Montmorillonite and Application of Sorption Models. Water Sci. Technol. 2013 Jan, 67(1), 152–158. doi:10.2166/wst.2012.488.
- Pehlivan, E.; Özbay, N.; Yargıç, A. S.; Şahin, R. Z. Production and Characterization of Chars from Cherry Pulp via Pyrolysis. J. Environ. Manage. 2017, 203, 1017–1025. DOI: 10.1016/j.jenvman.2017.05.002.
- Li, Z.; Mao, Y.; Yan, X.; Song, Z.; Liu, C.; Liu, Z. Design a flower-like Magnetic Graphite Carbon Microsphere for Enhanced Adsorption of 2,4-dichlorophenol. Environ. Sci. Pollut. Res.2022, 29(55), 83138–83154. DOI: 10.1007/s11356-022-21364-x.
- Ali, I.; Afshinb, S.; Poureshgh, Y.; Azari, A.; Rashtbari, Y.; Feizizadeh, A.; Hamzezadeh, A. Green Preparation of Activated Carbon from Pomegranate Peel Coated with zero-valent Iron Nanoparticles (Nzvi) and Isotherm and Kinetic Studies of Amoxicillin Removal in Water. Environ. Sci. Pollut. Res. 2020 Oct, 27(29), 36732–36743. DOI: 10.1007/s11356-020-09310-1.
- Akhtar, M.; Bhanger, M. I.; Iqbal, S.; Hasany, S. M. Sorption Potential of Rice Husk for the Removal of 2,4-dichlorophenol from Aqueous Solutions: Kinetic and Thermodynamic Investigations. J. Hazard. Mater. 2006, 128(1), 44–52. DOI: 10.1016/j.jhazmat.2005.07.025.
- Wang, J. P.; Feng, H. M.; Yu, H. Q. Analysis of Adsorption Characteristics of 2,4-dichlorophenol from Aqueous Solutions by Activated Carbon Fiber. J. Hazard. Mater. 2007, 144(1–2), 200–207. DOI: 10.1016/j.jhazmat.2006.10.003.
- Al Bsoul, A.; Hailat, M.; Abdelhay, A.; Tawalbeh, M.; Al-Othman, A. Efficient Removal of Phenol Compounds from Water Environment Using Ziziphus Leaves Adsorbent. Sci. Total Environ. 2021, 761, 143229. DOI: 10.1016/j.scitotenv.2020.143229.
- Mahmoudian, M. H.; Azari, A.; Jahantigh, A.; Sarkosh, M.; Yousefi, M. Statistical Modeling and Optimization of Dexamethasone Adsorption from Aqueous Solution by Fe3O4@NH2-MIL88B Nanorods: Isotherm, Kinetics, and Thermodynamic. Environ. Res, 2023 Nov, 236, 116773. DOI: 10.1016/j.envres.2023.116773.
- Azari, A.; Nabizadeh, R.; Mahvi, A. H.; Nasseri, S. Magnetic multi-walled Carbon nanotubes-loaded Alginate for Treatment of Industrial Dye Manufacturing Effluent: Adsorption Modelling and Process Optimisation by Central Composite face-central Design. Int. J. Environ. Anal. Chem. 2023 May, 103(7), 1509–1529. doi:10.1080/03067319.2021.1877279.
- Azari, A., et al. Magnetic NH2-MIL-101(Al)/Chitosan Nanocomposite as a Novel Adsorbent for the Removal of Azithromycin: Modeling and Process Optimization. Sci. Rep. 2022 Nov, 12(1), 18990. DOI: 10.1038/s41598-022-21551-3.
- Özkaya, B. Adsorption and Desorption of Phenol on Activated Carbon and a Comparison of Isotherm Models. J. Hazard. Mater. 2006, 129(1–3), 158–163. DOI: 10.1016/j.jhazmat.2005.08.025.
- Beker, U.; Ganbold, B.; Dertli, H.; Gülbayir, D. D. Adsorption of Phenol by Activated Carbon: Influence of Activation Methods and Solution pH. Energy Convers. Manag. 2010, 51(2), 235–240. DOI: 10.1016/j.enconman.2009.08.035.
- Ortiz-Martínez, K.; Reddy, P.; Cabrera-Lafaurie, W. A.; Román, F. R.; Hernández-Maldonado, A. J. Single and multi-component Adsorptive Removal of Bisphenol A and 2,4-dichlorophenol from Aqueous Solutions with Transition Metal Modified inorganic–organic Pillared Clay Composites: Effect of pH and Presence of Humic Acid. J. Hazard. Mater. 2016 Jul, 312, 262–271. DOI: 10.1016/j.jhazmat.2016.03.073.
- Zanella, H. G.; Spessato, L.; Lopes, G.K.P.; Yokoyama, J.T.C.; Silva, M.C.; Souza, P.S.C. Caffeine Adsorption on Activated Biochar Derived from Macrophytes (Eichornia Crassipes). J. Mol. Liq. 2021, 340, 117206. DOI: 10.1016/j.molliq.2021.117206.
- Al-Ghouti, M. A.; Da’ana, D. A. Guidelines for the Use and Interpretation of Adsorption Isotherm Models: A Review. J. Hazard. Mater. 2020, 393, 122383. DOI: 10.1016/j.jhazmat.2020.122383.
- Fornstedt, T. Characterization of Adsorption Processes in Analytical Liquid – Solid Chromatography. Journal of Chromatography. A. 2010, 1217(6), 792–812. DOI: 10.1016/j.chroma.2009.12.044.
- Hashemi, S. Y.; Azari, A.; Raeesi, M.; Yaghmaeian, K. Application of Response Surface Methodology (RSM) in Optimisation of Fluoride Removal by Magnetic chitosan/graphene Oxide Composite: Kinetics and Isotherm Study. Int. J. Environ. Anal. Chem. 2023 Dec, 103(17), 5368–5386. doi:10.1080/03067319.2021.1938021.
- Wang, W.; Wang, Z.; Li, K.; Liu, Y.; Xie, D. Enhanced Adsorption of Aqueous Chlorinated Aromatic Compounds by Nitrogen auto-doped Biochar Produced through Pyrolysis of rubber-seed Shell. Environ. Technol. 2023 Feb, 44(5), 631–646. DOI: 10.1080/09593330.2021.1980829.
- Girish, C. R.; Ramachandra Murty, V. Adsorption of Phenol from Aqueous Solution Using Lantana Camara, Forest Waste: Kinetics, Isotherm, and Thermodynamic Studies. Int. Sch. Res. Not. 2014 Oct, 2014(4), 1–16. doi:10.1155/2014/201626.
- Lu, H.; Zhu, Z.; Zhang, H.; Zhu, J.; Qiu, Y. Simultaneous Removal of Arsenate and Antimonate in Simulated and Practical Water Samples by Adsorption onto Zn/Fe Layered Double Hydroxide. Chem. Eng. J. 2015, 276, 365–375. DOI: 10.1016/j.cej.2015.04.095.
- Al-Duri, B. A Review in Equilibrium in Single and Multicomponent Liquid Adsorption Systems. Rev. Chem. Eng. 1995, 11(2), 101–144. DOI: 10.1515/REVCE.1995.11.2.101.
- Jeppu, A. G.; Girish, C. R.; Prabhu, B,; Mayer, K. Multi-component Adsorption Isotherms: Review and Modeling Studies; Springer International Publishing, 2023; Vol. 10. DOI: 10.1007/s40710-023-00631-0.
- León, A. Y.; Rincón, J. R.; Rodríguez, N.; Molina, D. R. Optimization of the Preparation Conditions for Cocoa shell-based Activated Carbon and Its Evaluation as Salts Adsorbent Material. Int. J. Environ. Sci. Technol. 2022, 19(8), 7777–7790. DOI: 10.1007/s13762-021-03687-3.
- Foo, K. Y.; Hameed, B. H. Insights into the Modeling of Adsorption Isotherm Systems. Chem. Eng. J. 2010, 156(1), 2–10. DOI: 10.1016/j.cej.2009.09.013.
- Jeppu, G. P.; Clement, T. P. A Modified Langmuir-Freundlich Isotherm Model for Simulating pH-dependent Adsorption Effects. J. Contam. Hydrol. 2012, 129–130, 46–53. DOI: 10.1016/j.jconhyd.2011.12.001.
- Qin, F.; Wen, B.; Shan, X. Q.; Xie, Y. N.; Liu, T.; Zhang, S. Z.; Khan, S. U. Mechanisms of Competitive Adsorption of Pb, Cu, and Cd on Peat. Environ. Pollut. 2006, 144(2), 669–680. DOI: 10.1016/j.envpol.2005.12.036.
- Mohammed, N. A. S.; Abu-Zurayk, R. A.; Hamadneh, I.; Al-Dujaili, A. H. Phenol Adsorption on Biochar Prepared from the Pine Fruit Shells: Equilibrium, Kinetic and Thermodynamics Studies. J. Environ. Manage. 2018 Nov, 226(July), 377–385. doi:10.1016/j.jenvman.2018.08.033.
- Pan, J.; Zou, X.; Wang, X.; Guan, W.; Yan, Y.; Han, J. Adsorptive Removal of 2,4-didichlorophenol and 2,6-didichlorophenol from Aqueous Solution by β-cyclodextrin/attapulgite Composites: Equilibrium, Kinetics and Thermodynamics. Chem. Eng. J. 2011, 166(1), 40–48. DOI: 10.1016/j.cej.2010.09.067.
- Hadi, S.; Taheri, E.; Amin, M. M.; Fatehizadeh, A.; Aminabhavi, T. M. Adsorption of 4-chlorophenol by Magnetized Activated Carbon from Pomegranate Husk Using Dual Stage Chemical Activation. Chemosphere. 2021, 270, 128623. DOI: 10.1016/j.chemosphere.2020.128623.
- Liu, J.; Wu, L.; Chen, X. Kinetic Model Investigation on lead(II) Adsorption Using silica-based Hybrid Membranes. Desalin. Water Treat. 2015, 54(8), 2307–2313. DOI: 10.1080/19443994.2014.898000.
- Lütke, S. F.; Igansi, A. V.; Pegoraro, L.; Dotto, G. L.; Pinto, L. A. A.; Cadaval, T. R. S. Preparation of Activated Carbon from Black Wattle Bark Waste and Its Application for Phenol Adsorption. J. Environ. Chem. Eng. 2019, 7(5), 103396. DOI: 10.1016/j.jece.2019.103396.
- Mohammadi, S. Z.; Darijani, Z.; Karimi, M. A. Fast and Efficient Removal of Phenol by Magnetic Activated carbon-cobalt Nanoparticles. J. Alloys Compd. 2020 Aug, 832, 154942. DOI: 10.1016/j.jallcom.2020.154942.
- Taheri, E.; Fatehizadeh, A.; Lima, E. C.; Rezakazemi, M. High Surface Area acid-treated Biochar from Pomegranate Husk for 2,4-dichlorophenol Adsorption from Aqueous Solution. Chemosphere. 2022, 295(February), 133850. DOI: 10.1016/j.chemosphere.2022.133850.
- Wang, J.; Guo, X. Adsorption Kinetic Models: Physical Meanings, Applications, and Solving Methods. J. Hazard. Mater. 2019, 390(November), 122156. DOI: 10.1016/j.jhazmat.2020.122156.
- Liu, Y.; Shen, L. From Langmuir Kinetics to First- and second-order Rate Equations for Adsorption. Langmuir. 2008, 24(20), 11625–11630. DOI: 10.1021/la801839b.
- Marczewski, A. W. Application of Mixed Order Rate Equations to Adsorption of Methylene Blue on Mesoporous Carbons. Appl. Surf. Sci. 2010, 256(17), 5145–5152. DOI: 10.1016/j.apsusc.2009.12.078.
- Marczewski, A. W. Analysis of Kinetic Langmuir Model. Part I: Integrated Kinetic Langmuir Equation (IKL): A New Complete Analytical Solution of the Langmuir Rate Equation. Langmuir. 2010 Oct, 26(19), 15229–15238. doi:10.1021/la1010049.
- Bashiri, H. A New Solution of Langmuir Kinetic Model for Dissociative Adsorption on Solid Surfaces. Chem. Phys. Lett. 2013, 575, 101–106. DOI: 10.1016/j.cplett.2013.04.072.
- Largitte, L.; Pasquier, R. New Models for Kinetics and Equilibrium Homogeneous Adsorption. Chem. Eng. Res. Des. 2016, 112, 289–297. DOI: 10.1016/j.cherd.2016.06.021.
- Tripathi, S.; Tabor, R. F. Modeling two-rate Adsorption Kinetics: Two-site, two-species, Bilayer and Rearrangement Adsorption Processes. J. Colloid Interface Sci. 2016, 476, 119–131. DOI: 10.1016/j.jcis.2016.05.007.
- Salvestrini, S. Analysis of the Langmuir Rate Equation in Its Differential and Integrated Form for Adsorption Processes and a Comparison with the Pseudo First and Pseudo Second Order Models. React. Kinet. Mech. Catal. 2018, 123(2), 455–472. DOI: 10.1007/s11144-017-1295-7.
- Hu, Q.; Liu, Y.; Feng, C.; Zhang, Z.; Lei, Z.; Shimizu, K. Predicting Equilibrium Time by Adsorption Kinetic Equations and Modifying Langmuir Isotherm by fractal-like Approach. J. Mol. Liq. 2018, 268, 728–733. DOI: 10.1016/j.molliq.2018.07.113.
- Ezzati, R. Derivation of Pseudo-First-Order, Pseudo-Second-Order and Modified Pseudo-First-Order Rate Equations from Langmuir and Freundlich Isotherms for Adsorption. Chem. Eng. J. 2020, 392, 123705. DOI: 10.1016/j.cej.2019.123705.
- Islam, A.; Khan, M. R.; Mozumder, S. I. Adsorption Equilibrium and Adsorption Kinetics: A Unified Approach. Chem. Eng. Technol. 2004, 27(10), 1095–1098. DOI: 10.1002/ceat.200402084.
- Guo, X.; Wang, J. A General Kinetic Model for Adsorption: Theoretical Analysis and Modeling. J. Mol. Liq. 2019, 288, 111100. DOI: 10.1016/j.molliq.2019.111100.
- Patil, P.; Jeppu, G. P.; Ramachandra Murthy, V.; Girish, C. R. A Review on Interaction of Phenolic Pollutant with Other Pollutants in the Binary Adsorption System. Desalin. WATER Treat. 2023, 285, 213–241. DOI: 10.5004/dwt.2023.29313.
- Revellame, E. D.; Fortela, D. L.; Sharp, W.; Hernandez, R.; Zappi, M. E. Adsorption Kinetic Modeling Using pseudo-first Order and pseudo-second Order Rate Laws: A Review. Clean. Eng. Technol. 2020, 1(October), 100032. DOI: 10.1016/j.clet.2020.100032.
- Acharya, A.; Jeppu, G.; Raju Girish, C.; Prabhu, B. Development of a Multicomponent Adsorption Isotherm Equation and Its Validation by Modeling. Langmuir. 2023 Dec, 39(49), 17862–17878. doi:10.1021/acs.langmuir.3c02496.
- Azari, A.; Yeganeh, M.; Gholami, M.; Salari, M. The Superior Adsorption Capacity of 2,4-Dinitrophenol under ultrasound-assisted Magnetic Adsorption System: Modeling and Process Optimization by Central Composite Design. J. Hazard. Mater. 2021 Sep, 418, 126348. DOI: 10.1016/j.jhazmat.2021.126348.
- Giri, D. D.; Jha, J. M.; Srivastava, N.; Hashem, A.; Abd Allah E. F.; Shah, M.; Pal, D. B. Sustainable Removal of Arsenic from Simulated Wastewater Using Solid Waste Seed Pods Biosorbents of Cassia Fistula L. Chemosphere.2022, 287(P3), 132308. DOI: 10.1016/j.chemosphere.2021.132308.
- Pei, T.; Shi, F.; Hou, D.; Yang, F.; Lu, Y.; Liu, C.; Lin, X.; Lu, Y.; Zheng, Z.; Zheng, Y. Enhanced Adsorption of Phenol from Aqueous Solution by KOH Combined Fe-Zn Bimetallic Oxide co-pyrolysis Biochar: Fabrication, Performance, and Mechanism. Bioresour. Technol. 2023, 388(August), 129746. DOI: 10.1016/j.biortech.2023.129746.
- Tien, C.; Ramarao, B. V. Further Examination of the Relationship between the Langmuir Kinetics and the Lagergren and the second-order Rate Models of Batch Adsorption. Sep. Purif. Technol. 2014, 136, 303–308. DOI: 10.1016/j.seppur.2014.08.013.