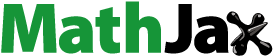
ABSTRACT
Microreaction and membrane technologies offer optimal conditions for controlling enantiomer synthesis and purification processes in continuous production, with numerous advantages over batch manufacturing. One of the many forces driving the development of such technologies for the production of single optical isomers is the need for enantiomerically pure pharmaceutical drugs because enantiomers may display opposite therapeutic effects or different treatment efficacies and side effects. Yet, despite advances in asymmetric synthesis and separation techniques, preparing enantiomerically pure compounds remains a challenging task. Here, we review the progress in microfluidics and membrane chiral separation over the last two decades. In addition to describing and critically assessing the state of the art in both disciplines, we provide an overview of their beneficial properties and characteristics for developing technologies toward producing enantiomerically pure compounds. Concomitantly, we evaluate efforts to integrate synthesis and membrane separation into a microfluidic platform and pinpoint the limiting factors that must be overcome before these platforms can be fully deployed in the industry.
1. Introduction
Chirality is a property of asymmetry between a chiral object and its mirror image, precluding their superimposition.[1] Among chiral molecules, optical isomers (enantiomers) stand out for their omnipresence in nature and organisms, including humans, and surprising homochirality, i.e., only one form of the enantiomeric pair is found in the living system.[Citation1Citation2] For example, proteins consist of only L-amino acids, and D-monosaccharide units are almost exclusively used as building blocks of nucleic acids.[Citation3] For these reasons, the homochirality of organisms has long puzzled scientists, who have proposed several theories to explain the evolution of homochirality, from the amplification of the initial imbalance in the enantiomeric concentrations to self-production autocatalysis.[Citation4]
Notwithstanding these efforts, the origin of homochirality is still unknown, even though its consequences have been described in many organisms. Homochirality is strongly reflected in the fate of individual enantiomers in organisms, e.g., in how they are processed or act. In turn, our senses have developed the ability to differentiate enantiomers of many chiral compounds.[Citation5] For instance, (-)-carvone smells like spearmint, whereas (+)-carvone smells like caraway,[Citation6] and D-asparagine is sweet, but L-asparagine is tasteless.[Citation7] Other examples of differences between enantiomers are also mirrored by pharmaceutical drugs and their therapeutic effects.[Citation8,Citation9] The administration of enantiomerically impure drugs, in particular, may have fatal consequences, such as the tragedy caused by thalidomide.[Citation10] Unlike (S)-thalidomide, which acts as a sedative, its R stereoisomer disrupts fetal development. Unbeknownst to physicians, who widely prescribed thalidomide to pregnant women suffering from morning sickness in the 1960s, this pharmaceutical drug was then produced as a racemic mixture. As a result, thalidomide soon led to a high neonatal morbidity and mortality, with an estimated survival rate ranging from 40 to 70%.[Citation11]
This example of different therapeutic effects illustrates the utmost importance of technologies for single enantiomer production, not only in the pharmaceutical but also in the cosmetic, agricultural and food industries.[Citation12–14] The two main strategies for producing pure, single-form enantiomers consist of (i) optimizing reaction conditions to exclusively yield one enantiomer[Citation15–20] and (ii) separating racemic mixtures into their individual optical isomers downstream synthesis reactions.[Citation21] The former is enabled by some enzymes under proper reaction conditions, whereas the latter requires using separation techniques for isomer purification. Regardless of their differences, both strategies have evolved as new technological developments were gradually introduced over time.
The late 1990s witnessed the emergence of micro- and nanotechnologies in chemistry and related fields. Soon after its inception, a new chemical engineering field known as microfluidics was predicted to transform chemical and pharmaceutical drug production.[Citation22,Citation23] This confidence stemmed from the intrinsic properties of microfluidic systems, such as short transport distances and large surface-to-volume ratios, empowering engineers with tools to precisely control reaction-transport processes.[Citation24,Citation25] The readiness of microfluidic systems to incorporate various functional elements paved the way to a seamless integration of several consecutive processes into a single production microfluidic unit[Citation26,Citation27] and to a continuous production of value-added chemicals.[Citation28] In 2001, Lindner and his colleagues listed microfluidics among prospective technologies for single enantiomer production, in a review on “Separation of enantiomers: needs, challenges, perspectives.”[Citation29] The authors stated that microfluidic technologies bring many advantages, including “the reduction of solvent, reagent and sample consumption, manufacturing, and maintenance costs, along with the improvement of the efficiency and the possibility of automation.”
Despite its potential, microreaction technology per se can help produce only a limited number of single enantiomers because this approach relies on separation systems for enantiomer purification. To make matters worse, the separation of optical isomers (enantiomers) is generally a complex and challenging task. In a recent review, Maier et al.[Citation29] summarized the needs and challenges of enantioseparations in various applications, including separations of proteins, oligo- and polysaccharides, antibiotics, and a group of low-molecular-mass molecules. Nevertheless, several separation techniques for optical isomers are currently available and routinely performed in many industries at full or preparative scales.[Citation30–34] These separations are primarily based on liquid chromatography and other adsorption techniques, which necessarily entail batch operations. In contrast, thanks to their compatibility with large-scale continuous production, enantioseparations employing membranes modified with enantiomeric selectors (groups) are considered alternatives to chromatographic methods.[Citation29,Citation33] Accordingly, combining microreaction technology with membrane-based microfluidic separations generates versatile platforms for single enantiomer production.
In this context, our review describes the role of microfluidic and membrane separation technologies for single enantiomer production in the last twenty years. We aim to outline essential features of microreaction and membrane separation technologies and to highlight their advantageous characteristics by describing a few experimental case studies in detail. More specifically, we assess these features and characteristics from the point of a chemical engineer seeking to apply these technologies in designing a new production process for catalyzed organic or enzymatic synthesis followed by product purification. In the last section, this review focuses on efforts to integrate synthetic and separation steps into a single microfluidic platform while providing insights into challenges engineers face in this area.
2. Continuous-flow microreactors for enantiomer and diastereoisomer synthesis
Asymmetric synthesis of value-added chemicals is the main task of catalysis as emphasized in recent reviews.[Citation35,Citation36] Continuous-flow microreactors are used in the organic,[Citation15,Citation37–63] enzyme,[Citation16–20,Citation64–76] or electrochemical[Citation68,Citation77] asymmetric synthesis of various enantiomers or diastereoisomers for various reasons. They have, however, one common characteristic, i.e., a microfluidic platform, which provides scientists with one or more significant advantages over classic laboratory or industrial equipment. Here, we summarize the main benefits of microscale by scrutinizing approximately 50 recently published papers (see ).
Table 1. Microreactors for the synthesis of enantiomers and diastereoisomers. O – organic synthesis, E – electrochemical synthesis, B – enzymatic synthesis, ee – enantiomeric excess (see EquationEq. (1)(1)
(1) for definition), er – enantiomeric ratio (concentration ratio of enantiomers), de – diastereomeric excess (analogy to ee), dr – diastereometric ratio (concentration ratio of diastereoisomers), y – reaction yield/conversion, tr – reaction/residence time, pr – productivity (quantity of a product produced per unit time), STY – space-time yield (quantity of a product produced per unit time per unit volume of a reactor), srr – specific reaction rate (quantity of a product produced per unit time and unit quantity of a catalyst), n.a. – not available.
Microreactor design and its construction must be resolved before starting the actual synthesis. Reaction conditions, including temperature, pressure, and chemical composition, determine which materials are suitable for building a specific microreactor. Some microreactors are made of transparent materials, such as glass or various plastics. Transparency allows chemical engineers to easily monitor and control reaction conditions or high photocatalytic performance.[Citation48,Citation57] Hence, microreactors used in enantiomer synthesis can be categorized based on their building materials.
Microreactors also differ in their internal arrangement. As in classical chemical reactors, microreactors can be classified into single- and multiphase reactors. The latter category can be further subdivided by phase arrangement, catalytic activity localization and function. In the following subsection, we address microreactor materials and designs.
2.1. Reactor material and design
Various types of microfluidic devices have been used for optical enantiomer and diastereoisomer production. The most straightforward options are microcapillaries with internal diameters ranging from hundreds of microns to millimeters connected by commercially available PEEK (polyether ether ketone) or steel connectors, among other materials,[Citation17–19,Citation38,Citation39,Citation41,Citation47–49,Citation51–53,Citation57–60,Citation66,Citation69] as shown in ). Fluoropolymers, such as PTFE (polytetrafluoroethylene),[Citation18,Citation38,Citation39,Citation49,Citation53,Citation58,Citation66] perfluoroalkoxy polymer,[Citation52] and fluorinated ethylene-propylene,[Citation17] remain the most popular microcapillary materials for their excellent chemical resistance and relatively high melting points. Borosilicate glass[Citation48,Citation69] and fused silica capillaries[Citation19,Citation47,Citation51] are transparent and chemically and thermally resistant. Stainless steel withstands high pressures and is, therefore, a material with optimal properties for organic synthesis.[Citation41,Citation59] In other words, even multistep processes with many inlet streams can be quickly designed thanks to the modularity of microcapillary systems.[Citation19,Citation52]
Figure 1. Basic designs of microreactors: (a) microcapillary systems, (b) microfluidic chips, (c) microcolumn systems, and (d) cascade systems.
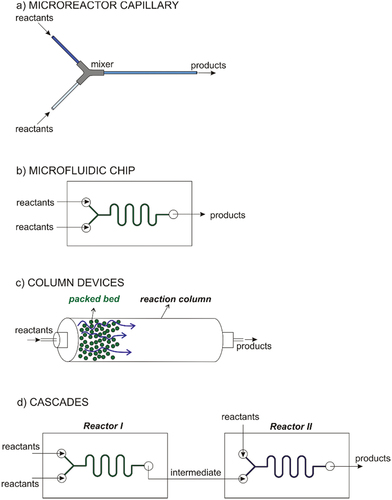
Microreactors conventionally consist of microfluidic chips ()) containing straight or meandering channels and channel bifurcations[Citation15,Citation16,Citation20,Citation40,Citation44,Citation45,Citation50,Citation57,Citation61,Citation62,Citation65,Citation67,Citation71–73,Citation77] and are built using various techniques, including advanced photolithography, soft lithography, micromachining, and 3D printing. Photolithography, soft lithography, electroerosion, and other techniques generate microchannels or embedded structures, such as electrodes with various characteristic dimensions, ranging from several microns to millimeters.[Citation62,Citation68,Citation71,Citation72] Easily accessible micromachining[Citation18,Citation73] or 3D printing[Citation50] produce microfluidic structures typically larger than several tens or hundreds of microns. The main materials used to manufacture microfluidic chips include glass,[Citation15,Citation40,Citation44,Citation45,Citation56,Citation61,Citation62,Citation65,Citation71,Citation72] which provide microreactors with high chemical resistance, cycloolefins,[Citation20] which are a less expensive alternative to glass, stainless steel,[Citation57] noble metals,[Citation68,Citation77] or various polymers, such as PMMA,[Citation73] and polylactic acid.[Citation50] Polymers are usually optically transparent and suitable for applications in an aqueous environment under mild conditions. Microreactors may also have a hybrid composition with several layers of different materials, e.g., PDMS/glass,[Citation67] metal/NafionTM,[Citation68] or glass/metal[Citation57] sandwiches.
Column devices ()) with millimeter or even centimeter diameters are also employed for enantioselective synthesis on a micro- or millimetric scale.[Citation42,Citation43,Citation54,Citation64,Citation70,Citation74,Citation76] These column devices are filled with microparticles,[Citation43,Citation64] microdroplets,[Citation76] or monolithic porous materials,[Citation42,Citation54,Citation70,Citation74] which immobilize the catalyst. The characteristic dimensions of cavities among the particles are units or tens of microns, thereby markedly intensifying mass transport in heterogeneous catalysis.
When assessing column devices, their benefits and disadvantages have to be carefully taken into account. On the one hand, they are easily designed and built. On the other hand, they lack some benefits specific to microscale applications. A case in point is the intensity of heat transport, which is limited by the small surface-to-volume ratio of the column devices. Flow control can also be difficult when non-uniform distribution of particles in a column and the wide particle size distribution. Ultimately, many experimental applications prove strong position of the column devices in the field of catalytic synthesis of optical enantiomers.
2.2. Microreactors and their benefits for enantiomer and diastereoisomer synthesis
A wide range of microreactors have been used to synthesize optically active chemicals. These microreactors contain one, two, or more phases, which actively participate in a chemical reaction. A similar number of studies have focused on homogeneous (single-phase)[Citation15,Citation16,Citation38–41,Citation45,Citation48,Citation49,Citation52,Citation53,Citation57,Citation59–61,Citation65,Citation73] and heterogeneous (multiphase) reactors,[Citation17–20,Citation42–44,Citation47,Citation50,Citation51,Citation54,Citation56,Citation58,Citation62–64,Citation66–72,Citation74,Citation76,Citation77] as outlined in .
2.2.1. Single-phase reactors
Organic synthesis often proceeds in a single-phase environment. Some reactions catalyzed by free enzymes fall into this category. Microfluidic reactors for homogeneous reactions are simple, consisting of either a capillary or microchannel with several inputs and outputs. Short reaction time, process safety, rapid prototyping, and fast optimization of reaction conditions are essential features of single-phase microreactors. These features have enabled the scientists to reach high reaction yields and enantiomeric or diastereoisomeric excesses.[Citation16,Citation38,Citation39,Citation49,Citation57,Citation60,Citation61]
The short reaction time and superior reaction parameters of microcapillaries and microchannels are attributed to their short transport distances. Reaction mixtures are primarily homogenized based on molecular diffusion because the flow is laminar in microreactors. Under these conditions, the diffusional mixing time is proportional to the square of the characteristic dimension of a microchannel. Consequently, in narrow microstructures, chemical reactions are completed within extremely short periods, as shown by Klusoň et al.[Citation45] These authors synthesized optical isomers of ethyl-4-chloro-3-hydroxybutyrate in a microchannel with a cross-section of 300 × 120 µm2 within 1 minute. Moreover, mixing can be enhanced by shaping the surface of the channel.[Citation73] In common microcapillaries, the reaction time mostly reaches minutes or tens of minutes. This reaction time is determined by the residence time, which can be controlled by adjusting the flow rate of the reaction mixture through the capillary as a function of its length. Such an excellent control over the residence time has allowed, e.g., the capture of unstable chiral organolithium intermediates and the consecutive synthesis of chiral allenes on a chip.[Citation59] The lack of a macroscopic alternative for this synthetic route underscores the power of microfluidics.
Microscale affects the heat management of reaction systems, as exemplified by the enantioselective borohydride reduction of ketones. This reaction proceeds successfully in a capillary microreactor at 10°C, 30°C higher than the reaction temperature used in batch systems (– 20°C). The reaction mixture can be kept at a higher temperature because the reaction heat is efficiently removed from the microreactor.[Citation41] Such an accurate temperature control has also been appreciated in the photocycloaddition of chiral cyclohexanone carboxylates with cyclopentene.[Citation48] As mentioned, e.g., in the synthesis of optically active epoxides,[Citation39] sulfoxides,[Citation38] or alcohols,[Citation40] process safety is, thus, a key standard of microreaction technologies. Microreactors for homogeneous enzyme catalysis have also opened up opportunities for synthesis and analysis integration[Citation65] and fast optimization of reaction conditions,[Citation73] further highlighting the advantages of single-phase microreactors.
2.2.2. Multiple-phase reactors
Multiphase microreactors are widely used for chiral synthesis. Contact with either partly miscible or immiscible fluid phases or with solid and fluid phases on a microscale creates the heterogeneous reaction environment.
The typical microreactors of this group are systems with two or more immiscible liquid phases[Citation56,Citation66,Citation67,Citation71,Citation72] or with one gas phase and one or more liquid phases.[Citation44,Citation78] The liquid-liquid flow takes the form of slug/droplet ()) or parallel ()) flow in microreactors.[Citation66,Citation67,Citation71,Citation72] Parallel flow provides an intensive interfacial transport of reactants/products between phases thanks to short transport distances. For example, parallel flow has guaranteed efficient substrate transport to an enzyme localized in another phase or efficient extraction of a product from the reaction phase.[Citation67,Citation71,Citation72] Gas-liquid flow in microchannels almost exclusively takes the form of a slug flow. The gas phase serves as a source of a gaseous reactant[Citation44] or as an inert medium stabilizing the complex flow.[Citation78]
Figure 2. Microreactors for heterogeneous catalysis: (a) liquid-liquid or gas-liquid slug-flow, (b) liquid-liquid co-current parallel flow, (c) hypothetical counter-current parallel flow with a catalytic membrane, (d) microcapillary, (e) packed-bed microcolumn, (f) monolithic microcolumn. Red lines and dots depict the location of catalysts.
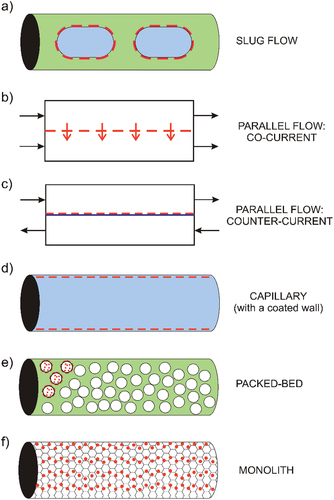
An interesting three-phase parallel flow microreactor for (S)-ibuprofen production was reported by Huh et al.[Citation67] Candida rugose lipase with partial enantioselectivity to the esterification of (S)-ibuprofen esterified racemic ibuprofen in the first phase. The resulting esters were selectively transported through a layer of ionic liquid (the second phase), subsequently entering the third phase where they were hydrolyzed back to ibuprofen by porcine pancreas lipase. Possible flow instability in longer microchannels or the inability to establish contact between phases in a counter-current arrangement ()) are some of the disadvantages of parallel-flow microreactors. However, a thin separation membrane with low transport resistance can stabilize a counter-current flow.[Citation79] As such, this is a powerful arrangement for the synthesis of enantiomers because a chiral catalyst can be immobilized directly in the membrane, or the membrane can serve as a chiral sieve.
Slug or droplet flow of two immiscible phases is an alternative to parallel flow. The slugs are the source of a reactant or catalyst[Citation66] or enable the extraction of reaction products.[Citation80] Horizontal microreactors with a slug flow operate only in a co-current arrangement. This flow is characterized by the high stability and uniform residence time of all slugs. Internal circulation in slugs and the formation of thin layers of the continuous phase between slugs and microchannel walls enhance mass transport.[Citation81] When Cech et al.[Citation66] employed a slug flow microreactor for the diastereoisomeric synthesis of L-phenylserine, they found that the choice of reaction time and the chemical composition of both phases determined the diastereoisomeric ratio of syn- and anti-L-phenylserine. L-anti-phenylserine was predominantly produced in kinetic regimes characterized by short residence times. Thus, evidence shows that asymmetric enantiomer synthesis often relies on kinetic resolution.[Citation82]
Microreactors with the catalyst immobilized on the channel or capillary walls ()) represent another group of heterogeneous systems.[Citation18–20,Citation47,Citation51,Citation63,Citation69] As mass transport is usually the rate limiting step in heterogeneous organic or enzymatic catalysis, the characteristic dimension of the microstructures must be much smaller than 1 millimeter, typically ranging from 200 to 500 µm. Asymmetric catalysts coated on microchannel walls exhibit high stability in the long-period continuous-flow synthesis of enantiomers and multiple reuses.[Citation47,Citation63,Citation69] Chiral Mn–salen imine gel,[Citation47] chiral vanadium–salen complexes on polysiloxanes,[Citation51] or dirhodium catalyst layered in hollow fibers [Citation63] are examples of how chiral catalysts for organic synthesis can be immobilized in microreactors. Furthermore, lipase immobilized in a thin film of mesoporous silica,[Citation69] cylindrical membranes composed of aminoacylase and poly-lysine matrix,[Citation18] His6-tagged enzymes transketolase and transaminase immobilized on silica microcapillaries[Citation19] or transaminase immobilized in microchannels by surface silanisation and glutaraldehyde bonding[Citation20] have all been successfully used in enzymatic transformations
Packed bed[Citation17,Citation43,Citation50,Citation58,Citation62,Citation64] ()) and monolithic[Citation42,Citation54,Citation70,Citation74] ()) micro- or minireactors are used not only in asymmetric synthesis but also in traditional applications, such as the Fischer-Tropsch synthesis. They display superior heat and mass transfer properties[Citation83] and long-term biocatalyst[Citation64] and organic catalyst[Citation42,Citation43,Citation54] stability. By way of illustration, microreactors developed by Chiroli et al.[Citation42] and Kasaplar et al.[Citation43] continuously operated for 8 h and 24 h, respectively, or phenylalanine ammonia-lyase immobilized on carboxylated single-walled carbon nanotubes arranged into a packed bed did not significantly lose enzymatic activity in ammonia addition for at least 72 h.[Citation64] The packed bed columns contained catalysts immobilized on functionalized polystyrene,[Citation43] carbon,[Citation50] silica,[Citation62] agarose,[Citation17] Eupergit[Citation58] particles and single-walled carbon nanotubes.[Citation64] MacMillan catalytic monolith,[Citation42] functionalized polystyrene polymer resin,[Citation54] silica monolith with immobilized enzymes[Citation70,Citation74] are three representative examples of catalytic microreactors with different types of monoliths.
Some uncommon heterogeneous microreactors are also available for chiral synthesis.[Citation68,Citation76,Citation77] Cheikhou et al.[Citation68] developed an electrochemical microreactor with a finely structured gold electrode for the enzymatic synthesis of L-lactate from pyruvate. The finely structured gold cathode regenerated FADH2, which, in turn, regenerated the reducing agent NADH. Gao et al.[Citation77] reported the enantioselective electrochemical α-lactonization and α-alkoxylation of diketo acid derivatives with chiral iodoarenes used as electron-transfer mediators in a continuous flow microreactor. Zhang et al.[Citation76] developed a continuous flow catalysis system, using ionic liquid (IL) droplets dispersed in oil in the form of a packed bed layer. The IL droplets accommodated the enzyme lipase with continuous substrate supply and product removal from/into the oil phase.
2.2.3. Cascade systems
The unique properties of microfluidic reactors for organic and enzymatic catalysis allow continuous multiple-step syntheses of optical enantiomers ()). The in-series connection of various catalytic microreactors and the possibility to supply reactants and to remove intermediates continuously are indisputable advantages of microsystems. Inherently, the cascade arrangement of microreactors indicates the most likely future directions in the production of special chemicals, such as enantiomers.
Microreactor cascading has successfully been demonstrated in several experimental studies.[Citation16,Citation17,Citation19,Citation50,Citation52] L-erythrulose was synthesized from β-hydroxypyruvate and glycolaldehyde by immobilized transketolase in a microcapillary reactor. L-erythrulose was then continuously fed into another capillary microreactor and converted by a transaminase to 2-aminobutentriol therein.[Citation19] The same enzymatic transformations were efficiently performed in a cascade of packed bed systems[Citation17] or in a homogeneous arrangement with cell lysates instead of immobilized enzymes.[Citation16] An example from organic catalysis is the two-step synthesis of valuable chiral 1,2-amino alcohols in a cascade system.[Citation50] In the first step, β-nitro alcohols were synthesized through nitroaldol reactions using a copper catalyst in a 3D printed microreactor. The β-nitro alcohols were then hydrogenated on a Pd/C catalyst in a packed-bed microreactor. Shu and Buchwald[Citation52] developed a three-step lithiation/borylation/1,4-addition sequence for the enantioselective β-arylation of ketones. The whole process was conducted in a series of three microcapillary reactors.
2.2.4. Productivity and space-time yield
Information on microreactor productivity, which is defined as the quantity of product produced per unit time, is reported in only a few papers listed in . Some of the microreactors were used exclusively in technology demonstrations or in the implementation of in-situ product analysis. Microreactor productivity is usually lower than industrial-scale reactors, ranging from less than 1 mg h−1 to 15 g h−1. These values imply that microreactors can be used in low-scale production technologies. The synthesis of special chemicals, such as some enantiomers, may, thus, become an essential application of microreactors. The ability to produce unstable intermediates in multiple-step syntheses further strengthens the position of microreactor systems in enantiomer synthesis.[Citation52,Citation59] Unstable intermediates can be contacted with other reactants to yield enantiomers because the residence time can be precisely controlled in microchannels or microcapillaries. When necessary, microreactor productivity can be further increased by massive parallelization.[Citation38]
The space-time yield (STY) in microreactors, defined as the quantity of a product produced per unit time per unit volume of a reactor, is usually high thanks to short transport distances and to high surface-to-volume ratios. Several studies have reported that STYs reach significantly higher values in microreactors than in benchmark batch macro-sized reactors under comparable experimental conditions.[Citation44,Citation49,Citation57] For example, Dai et al.[Citation38] achieved an STY of approximately 3750 g L−1 h−1 in the synthesis of optically active sulfoxides, and functionalized 1,2-diamines have been synthetized with an STY of 192 mmol L−1h−1.[Citation49] In enzymatic synthesis,[Citation74] STY values have even reached 1229 g L−1 h−1. These values are similar or even higher than those reported in a recent review on continuous flow biocatalysis.[Citation84] Similarly, specific reaction rates often reach higher values in microreactors than in batch reactors.[Citation42,Citation64,Citation74] Overall, the production performance of microreactors suggests that this technology has a great potential for enantiomer synthesis intensification.
3. Membrane separation technologies
3.1. Introduction
Despite significant progress in asymmetric syntheses and separation techniques, the preparation of enantiomerically pure compounds remains a challenging task. Although enantiomers exhibit identical chemical and physical behavior in achiral surroundings, their biochemical and pharmacological properties can differ significantly. For example, enantiomers may possess different therapeutic efficacy and side effects. There is currently a strong tendency to use enantiopure drugs to reduce the burden of ineffective or even harmful enantiomers in patients’ organs.[Citation85] Moreover, due to the huge quantity of racemic drugs on the market, environmental problems may arise from drug residues and metabolites – within urine and feces.[Citation86] Therefore, many chiral drugs have been redeveloped as single enantiomers; nevertheless, there is still a significant portion of racemic drugs among the recently approved new molecular entities. The examples of the chemical structures of the chiral molecules important for enantiomerically pure drugs are in .
Table 2. Examples of the chemical structures of the chiral molecules important for enantiomerically pure drugs.
Enantiomers can only be separated when they occur in a chirally active environment. The individual enantiomers interact with such an environment differently since they vary in the spatial molecular arrangement. Scriba et al. discussed in detail the problem of enantiomeric recognition in separation science,[Citation96] listing potential chiral selectors available to modify surfaces of particulate carriers or separation membranes.
Numerous methods for preparing chiral environments are currently available worldwide. An excellent overview of these methods has been recently presented by Zaera.[Citation97] In turn, Powell et al.[Citation98] have summarized some techniques for creating chiral environments, but their list is restricted to a selected group of applications. The methods for preparing optically active chiral compounds with prospective uses as chiral selectors have been reviewed by Patti.[Citation99] Presently, the separation techniques employed for separating optical isomers mainly include gas chromatography (GC) and high-performance liquid chromatography (HPLC) based on chiral stationary phases and advanced crystallization processes. These techniques support the effective production of single enantiomers with the desired degree of purity. However, the demand for even more affordable and faster continuous methods for enantiomer separation persists, thus requiring further research on alternative approaches.
Membrane separations are a promising option for enantiomer separation because they achieve high selectivity at reasonable energy costs[Citation100,Citation101] in continuous production. Moreover, membrane separations meet the criteria for zero-waste technologies, and they are applicable under various processing conditions and to existing production–separation facilities straightforwardly (e.g., industrial-scale applications of membrane modules in seawater desalination, alcohol dehydration, and gas mixture separation). This chapter reviews the main types of membranes along with their chiral modifications, focusing on their advantages and disadvantages.
Membrane separation processes are used in many technological areas at vastly different scales ranging from large-scale industrial installations (see, e.g., monographs by Rautenbach,[Citation102] Mulder[Citation103] and Baker,[Citation104] and the review by Strathmann[Citation105]) to laboratory small-scale equipment.[Citation26,Citation106,Citation107] Advancements in the preparation of membranes with tailored properties of their materials – structure and physicochemical parameters – has provided membranes which can be integrated into microfluidic devices or fabricated within them (in situ)[Citation26,Citation108] These membranes can also perform highly efficient separations of components varying in molar mass (molecular size), shape (membrane filtrations), electric charge (electro-membrane separations), or other physicochemical properties, including their optical activity. To enhance separation effects, membrane separation may be combined in situ with other separation techniques, such as liquid-liquid extraction.[Citation80] Moreover, an active catalytic membrane is able to simultaneously catalyze a reaction and separate the reaction product.[Citation108]
Striking developments in membrane engineering open up new opportunities for continuous-flow synthesis and separation of chiral chemicals. Easy membrane formatting allows their incorporation into various flow-through modules. In addition to membrane selectivity and permeability, the surface-to-volume (SV) ratio is another key property of membrane modules. A high SV ratio promotes an efficient mass transfer of chemical components through a membrane surface. Microfluidic devices are always characterized by their high SV ratios and as such are a promising platform for fully exploiting the specific properties of newly developed membranes.
3.2. Membranes for chiral separations
Enantiomerically selective membranes separate mixtures of optical isomers, such as pharmaceutical enantiomers, amines, or amino acids and their derivatives.[Citation109,Citation110] These membranes can be divided into two basics groups: i) liquid membranes[Citation104,Citation111–114] and ii) solid membranes.[Citation115–117] The separation principle is based on specific interactions of atoms and/or groups of atoms of an enantiomer molecule with the surface atoms or groups of atoms at the membrane surface. These interactions account for variations in the transport rate of a given enantiomer through the membrane. Modifying the membrane surface by attaching/bonding specific molecules known as chiral selectors can help to control or adjust these interactions (at least to a certain extent). Chiral selectors usually provide solid membranes with high selectivity, albeit, unfortunately, often at the cost of slow transport rate. In contrast, liquid membranes may offer both advantages, i.e., they afford high selectivity whilst preserving high diffusivity. Yet, they often have problems with long-term stability. While liquid membranes prevailed at the early stages of the search for enantioselective membranes, solid membranes have recently become an attractive alternative because they often contain a chiral polymer or a chiral selector, such as a protein, for example.[Citation101,Citation118]
3.2.1. Liquid membranes
Liquid membranes with mobile carriers are among the most selective membranes thanks to their fast mass transfer (see ). The type and degree of selectivity depend on the properties of the carrier molecule.[Citation120] A few studies have used hydrophilic liquid membranes to separate organic molecules.[Citation121] Ionic liquids incorporated into porous, commercially available membranes are a relatively new direction in liquid membrane development. The supported ionic liquid membranes () are usually prepared by impregnating the polymeric porous material with the ionic liquid. In direct contact with the liquid mixture, the support must be therefore well wetted by the ionic liquid and sufficiently chemically and mechanically resistant. The stability of the ionic liquid in the membrane can be improved by coating the membrane with a suitable polymer. Such a polymer also protects the liquid membrane against the corrosive effects of the liquid feed mixture.[Citation122,Citation123]
Figure 3. Scheme of the transport mechanism in a supported ionic liquid membrane with mobile carriers (inspired by[Citation119]).
![Figure 3. Scheme of the transport mechanism in a supported ionic liquid membrane with mobile carriers (inspired by[Citation119]).](/cms/asset/5beecff4-9356-43a0-82bc-c530539674ae/lctr_a_1977009_f0003_oc.jpg)
Cyclodextrins were first used as membrane carrier molecules to separate hydrophobic isomers by Armstrong et al.[Citation120] These authors reported enantiomeric and isomeric enrichments when using aqueous cyclodextrin liquid membranes. Combining catalytic ionic liquids with membrane separation technologies may thus lead to new and efficient ways to separate different enantiomers from complex mixtures.[Citation124] Such multifunctional membranes may enable the simultaneous synthesis and separation of valuable products, thus circumventing the need for multistep separation–reaction processes.
3.2.2. Solid membranes
Solid membranes (see .) are highly stable over time and suffer less from fouling than liquid membranes. The separation mechanism is based on the molecular orientation of atoms and/ or groups, leading to differences in the diffusion rates of individual enantiomeric compounds through the membrane. Their steric conformation is essential for key molecular interactions between the membrane and the chiral compound.[Citation126,Citation127] The fast transport and high selectivity of some solid membranes for enantiomer separation also solve the trade-off between permeability and selectivity underlying gas separations.[Citation128] Therefore, membrane area is a critical factor of enantiomeric separations in large-scale processes.
Figure 4. The mechanisms of facilitated and retarded transport of enantiomers through a solid Graphene oxide membrane (inspired by[Citation125]).
![Figure 4. The mechanisms of facilitated and retarded transport of enantiomers through a solid Graphene oxide membrane (inspired by[Citation125]).](/cms/asset/4b507c42-4def-4d2a-86f1-02b9a71473c5/lctr_a_1977009_f0004_oc.jpg)
Sorption-selective membranes show a higher potential for enantiomeric applications. Enantiomeric compounds are mainly separated by concentration-driven or pressure-driven permeation.[Citation129] By reaching a high optical resolution in a single step, membrane separation becomes more advantageous than traditional separation methods, such as crystallization or chemical modification, which can rarely be performed in this way.[Citation116] High selectivity often relies on membrane surface modification,[Citation130,Citation131] tailored crosslinking,[Citation131] or specific molecular conformation of the membrane.[Citation132,Citation133] Molecularly imprinted materials are thus promising selective materials because they specifically recognize the separated enantiomer. Nevertheless, other parameters such as the maximal temperature limits, mechanical resistance of the membrane, trans-membrane pressure, input flow rates, and the operating pH range must also be addressed in enantioseparation.
3.2.3. The mechanism of enantioseparation by chiral membranes
The binding affinity of an enantiomer to a chiral selector results from various intermolecular interactions, including hydrogen bonding, hydrophobic, Coulomb or van der Waals interactions, and steric effects. Two mechanisms have been proposed for enantiomer separations: facilitated and retarded transport (see ). [Citation134] In the subsections below, we analyze these mechanisms in more detail.
3.2.3.1. Facilitated transport of enantiomers through chiral membranes
Facilitated transport is characterized by enantiomer-membrane interactions which accelerate enantiomer transport through membranes.[Citation135] Membranes exhibiting facilitated transport (see , left side) are also known as diffusion-enantioselective membranes because enantioselectivity decreases abruptly when increasing driving forces, such as pressure, which create convective flow through membranes.[Citation107]
Contrary to adsorption-driven transport in solid membranes, facilitated transport is typical of liquid membranes.[Citation113,Citation136] For example, facilitated transport was observed when using N-3,5-dinitrobenzoyl-l-alanine-octylester as the chiral selector in a polypropylene hollow fiber module (with a length of 75 cm, 3 microporous polypropylene tubes with an inner diameter of 5.5 mm, and an effective area of 0.04 m2)[Citation136] for the racemic separation of D-/L-lactic acid and D-/L-alanine. The enantiomeric excess of lactic acid increased from 9.76 (after 20 hours) to 17.06% (after 164 hours), while the enantiomeric excess of alanine decreased from 20.00 (after 11 hours) to 12.06% (after 165 hours).
D-/L-lactic acid has also been separated using both chiral solid and supported liquid membranes,[Citation137] albeit with N-3,5-dinitrobenzoyl-l-alanine-octylester as the chiral selector in both cases. The solid membrane showed the highest enantiomeric excess (86.3%) after 233 hours of operation, while the liquid membrane supported with the same chiral selector reached only 24.6% enantiomeric excess after 141 hours.
The facilitated transport of enantiomers through chiral membranes is usually faster than retarded transport. However, retarded transport is usually more selective and higher enantiomeric excess is achieved.
3.2.3.2. Retarded transport of enantiomers through chiral membranes
Retarded transport occurs when one enantiomer binds more strongly or for a longer time to the membrane. Consequently, its permeation through the membrane is slower than that of the preferably transported enantiomer (see , right side). In other words, membranes exhibiting retarded transport retain the adsorbed enantiomer, while the other enantiomer crosses the membrane more easily because of its lower affinity to the chiral recognition sites. The membranes that fall under this category are known as adsorption-enantioselective membranes.
Adsorption-enantioselective membranes usually incorporate chiral selectors[Citation128] and generally maintain selectivity even when increasing transmembrane convective flow. In addition, their binding capacity determines their separation efficiency. Based on their properties, these membranes are expected to simultaneously have relatively high flux and enantioselectivity. For these reasons, they have a higher potential for industrial-scale productions of optically pure compounds than diffusion-enantioselective membranes[Citation138] and are, therefore, more commercially valuable.
Retarded transport is well documented in the enantioselective solid poly(methyl methacrylate) membrane containing (–)-oligo[methyl(10-pinanyl)siloxane] with a surface layer modified by D-/L-tryptophan. On this composite membrane, separation has reached a reasonable enantiomeric excess of 86%.[Citation115] The membrane was designed to maintain a low surface energy and to selectively sorb the L-isomer from the mixture of enantiomers. A solid membrane with improved selectivity has also been prepared by crosslinking sodium alginate and chitosan and used to separate the D-/L-tryptophan mixture[Citation139] with the enantiomeric excess surpassing 98%.
Another example of an enantioselective solid membrane is a blend of cellulose, sodium alginate, and hydroxypropyl‐β‐cyclodextrin. This membrane has reached an enantiomeric excess of more than 89% in the separation of p‐hydroxy phenylglycine[Citation117] as chirally active centers formed in spaces between helical conformations of cellulose and sodium alginate. In those spaces, hydroxypropyl‐β‐cyclodextrin was attached to the macrocyclic ring, creating a chiral recognition surface on the membrane. The separation mechanism was ascribed to three steps: adsorption, diffusion leading to specific enantiomer transport through the membrane, and desorption from the other side of the membrane. The first step of adsorption resulted from specific optical resolution, while the following diffusion depended on the concentration gradient of the separated enantiomer. The chiral selector may also be attached to the inner surface of porous hollow fiber membranes. Under these conditions, the surface of the internal membrane can be significantly higher than the typical surface of a liquid membrane with a dissolved chiral selector,[Citation137] but the mass transfer rate is lower in the former than in the latter.
3.3. Quantification of membrane separations
Enantioselective membranes show different permeabilities for different enantiomers in the mixture. Here, we focus on liquid mixtures containing a solvent and solutes represented by a pair of optical isomers. Membrane selectivity to individual optical isomers is usually expressed by an enantiomeric excess (ee), a common measure of purity used for chiral substances. In principle, ee expresses the extent to which a mixture contains one enantiomer in a higher amount than the other. An ee value of a mixture of enantiomers equals 0%, while the ee value of a single pure enantiomer equals 100%. The enantiomeric excess can be assessed using molar fractions of the enantiomers in a mixture as expressed in the following equation:
where XD and XL are the molar fractions or molar amounts of D- and L- enantiomers in a stream, typically in the permeate. The optimal membrane shows both high permeability and selectivity. For this reason, the membrane material must be selected based on the classical trade-off whereby an increase in permeability is generally followed by a decrease in selectivity and vice versa.
3.4. Nanofiltration, ultrafiltration, reverse osmosis and composite membranes
Nanofiltration membranes are attractive for various enantiomeric separations because they are relatively inexpensive and produced on a large scale. Furthermore, nanofiltration membranes can be easily modified by attaching a ligand to the surface of their selective layer.[Citation101] These membranes are used in important enantiomeric separations of amino acids and lactic acid.[Citation110,Citation140]
A membrane with specific enantioselectivity imparted by a chiral channel protein (FhuAF4) has been employed to separate a racemic mixture of D-/L-arginine.[Citation109] The membrane transport was governed by diffusion, as commonly performed for gas separations in microporous crystalline membranes, with the enantiomeric excess reaching 23.91% at a conversion of 52.39%. Thus, membranes with a specific protein as a chiral selector have a great potential for future enantiomeric separations.
Singh et al.[Citation141] separated an aqueous mixture of enantiomers of arginine and alanine on a chiral selective nanofiltration (NF) membrane incorporated with S-(-)-2-acetoxypropionyl chloride as the chiral selector. The membrane support layer was made of polysulfone (PS). The D- enantiomer preferentially permeated through this membrane, and the flux of arginine and alanine through the PS support was more than 5 times higher than that through the composite membrane. This difference in fluxes implies that the chiral selector acted as a flow restriction element exhibiting a proper selective function. The feed was aqueous solutions with a concentration of 1 g/L, and the transmembrane pressures ranged between 172 kPa and 861 kPa, which are reasonable from a technological and economic point of view. The membrane modules necessary for industrial-scale production consisted of four permeate cells arranged in series. The effective circular area of the individual flat membranes was 15.2 cm2. The enantiomeric excess was higher than 90% for the D- enantiomer of both arginine and alanine. The selectivity and the membrane rejection (), where cDP and cDF are the solute concentration in the permeate and feed/retentate solutions, respectively) of arginine and alanine ranged from 76 to 92%, depending on the experimental conditions. The solute molecules did not interact with the chiral centers at higher trans-membrane pressures. Therefore, the permeability increased with the trans-membrane pressure, but the pressure had the opposite effect on the membrane enantioselectivity. The nanocomposite membrane showed a good potential for enantiomeric separation due to its time-independent enantioselectivity.
A new chiral selective thin film, supported by a nanofiltration polysulfone membrane has been used to separate a mixture of enantiomers of α-amino acids (arginine and asparagine).[Citation142] The 0.5–1.0-µm-thick selective thin layer was prepared from a mixture of D-arginine, piperazine, and trimesoyl chloride. The permeability experiments were performed for 2 to 10 hours at a pressure of 1034.21 kPa in continuous mode. The circular membrane sheet had an effective area of 1.994 × 10−3 m2. The feed contained 5.7 mM aqueous solutions of racemic arginine and asparagine, with L-arginine preferentially permeating through the composite membrane due to possible interactions between chiral centers present in the membrane and arginine enantiomers. The permeate composition was independent of the experimental time (10 hours). This finding suggests that the membrane-solute interactions did not lead to irreversible changes in membrane properties and separation performance. The highest enantiomeric excess (ee) was >91% for L-arginine and >56% for L-asparagine in the permeate stream.
Another interesting approach was reported by Singh et al.,[Citation143] who studied the separation of α-amino acids (arginine and alanine) by reverse osmosis. They employed an enantioselective composite nanofiltration membrane prepared from a mixture of zinc metal Schiff base complex and piperazine with trimesoyl chloride on the top of a polysulfone ultrafiltration membrane. The feed (5.7 mM and 11.4 mM solutions of racemic arginine and alanine) flowed through the module at pressures of 517.1 kPa and 1034.21 kPa. The D-enantiomers of both α-amino acids permeated preferentially, with an enantiomeric excess in retentate for D-arginine of 54% when using a 11.4 mM arginine solution at a pressure of 517.1 kPa for 10 hours. The selectivity stemmed from the attached chiral centers of the Schiff complex. The interactions between the chiral membrane and D-arginine were stronger than between the chiral membrane and D-alanine, whose enantiomeric excess was only 21%. Membrane separation was independent of time. Therefore, incorporating the metal Schiff base complex led to a stable membrane with improved enantioselectivity.
Ingole et al.[Citation110] have successfully separated a mixture of enantiomers of α-amino acids (lysine and asparagine) using a membrane consisting of porous support (polysulfone ultrafiltration membrane) and a polymeric selective layer. The selective layer was based on polyamide polymerized with a mixture of L-arginine and trimesoyl chloride. The feed containing 1.0 g/L of racemic lysine and asparagine was kept at a pressure of 689.5 kPa. The membrane had an effective circular area of 19.54 cm2. The asparagine flux was slightly higher than that of lysine. The D-lysine permeated preferentially and reached an enantiomeric excess as high as 92% in the permeate stream. A lower enantiomeric excess of 68% was achieved for asparagine. Although the membrane was less enantioselectivity to asparagine than to lysine because the former is smaller and its interactions with the chiral centers are weaker, the transport properties of the membrane changed over time for both lysine and asparagine enantiomers under constant experimental conditions. Therefore, this study has helped to understand interactions between a chiral membrane and individual enantiomers, showing that membrane surface modifications improve mechanical resistance and membrane flux, as well as enantioselectivity owing to preferential interactions between the chiral membrane and the specific enantiomer.
Recently, Izak et al. reported a unique composite nanoµ fibrous membrane displaying enantioselective properties.[Citation144] The supporting layer was made of a polyethylene/polypropylene bicomponent non-woven fabric/material – Polyamide 6 nanofibre. The thin-film nanocomposite layer consisted of m-phenylene diamine mixed with 1,3,5-trimesoyl chloride and (S,S)-1,2-diaminocyclohexane. The (S,S)-1,2-diaminocyclohexane included m-phenylene diamine reacted with 1,3,5-trimesoyl chloride. Then, the polyamide (PA) active layer on the PA6 nanofibre surface was created. The thin PA layer was bound to the fibers in the membrane by Van der Waals forces. The membrane achieved 99% enantioselectivity for the mixture of D-/L-tryptophan, as shown in .[Citation146] Based on the above, incorporating chiral centers into the nanoµ fibrous membrane is a promising concept for future enantiomeric separations, which may be accelerated by applying another (magnetic or electric) driving force for mass transfer through the membrane. A more detailed study is underway.
Table 3. Chiral membranes and their performance for representative enantiomers.
3.5. Membranes based separations in microfluidic or nanofluidic devices
Continuous production is a sought-after mode of operation on an industrial scale. As mentioned in the introduction of this review, the concept of integrated microfluidic platforms is consistent with the continuous production of chemicals. Surprisingly, only a few papers on membrane-based microfluidic or nanofluidic chiral or enantioselective separations have been published thus far, especially considering how appealing simultaneous reaction and chiral separations of racemic ibuprofen in an inorganic polymer micro-contactor are from a technological point of view.[Citation147] In fact, one of the ibuprofen enantiomers has been transported through a pseudo-membrane through a stream of an ionic liquid separating an aqueous and organic phase inside a microchannel under laminar flow conditions. The enzymatic reaction in the organic phase flow yielded the S-ibuprofen-ester intermediate. This intermediate was converted into S-ibuprofen in a reaction with sulfuric acid in the aqueous acceptor phase. The conversion of the enzymatic reaction depended on the thickness of the ionic-liquid membrane and increased up to 92.5% at 100 μm thickness, reaching an enantiomeric excess of 99.9%.[Citation147]
A different approach to microfluidic device-based enantioselective separations can be found in a paper by Wang et al.[Citation148] They replaced a stationary chromatography phase with a porous enantioselective membrane. The porous PVDF membrane functionalized with BSA separated tryptophan/thiopental mixtures.[Citation148] The membrane was fixed in a sandwich-like structure between PDMS slabs with microchannels. The high SV ratio of the membrane structure and the variations in the mobile phase conditions (pH and chemical composition) were used to reach optimal chiral separation efficiency. The maximum separation factor of 1.74 was achieved for thiopental by adding ammonium sulfate to Tris buffer of pH 6.[Citation148]
Another approach for enantioselective separation on a microscale was applied by Fakhari et al.,[Citation149] who performed surfactant-assisted electromembrane extraction of Tranylcypromine enantiomers. The addition of surfactant facilitated the transport of the particular enantiomer into a hollow fiber (HF) and consequently to the acceptor solution.[Citation149] The hollow fiber was submersed in 2-nitrophenyl octyl ether for 15 s to create a supported liquid membrane. The fiber was thermally closed at one end. The acidic acceptor solution was injected with a microsyringe into the lumen of the hollow fiber. Lastly, the positive electrode was inserted directly into a 4.5 ml vial containing the racemic sample/surfactant mixture, and the negative electrode was placed inside the HF lumen (inner diameter of 0.6 mm, wall thickness of 200 µm, polypropylene). The power supply was then turned on, performing electromembrane extraction[Citation149] using a procedure similar to that previously reported by Nojavan et al., who applied electromembrane extraction on a microscale to extract amlodipine enantiomers using HF with a supported liquid membrane.[Citation150] The results clearly that showed electromembrane extraction with capillary electrophoresis is a fast and straightforward combination for the chiral separation of pharmaceuticals in biological matrices.[Citation150]
4. Coupled reactor-separator microsystems for chiral synthesis and separation
In the previous two chapters, we described various microreactors and membranes for the enantiomer synthesis and separation, respectively, discussing their performance. Both the synthetic step in a microfluidic reactor and the downstream processing in membrane modules are typically continuous operations. Therefore, their direct integration should naturally be a continuous operation providing an optimal process arrangement for producing optically active species. Although this integration offers optimal production conditions, only a few published reports address such integrated systems. In this section, we focus on these systems with arrangements of microreactors and separation technologies adapted to a microscale. These integrated separation technologies are membrane separations, microextraction, capillary electrophoresis, and microchromatography (see ). Basic information on these integrated technologies is summarized in .
Figure 5. Integrated microfluidic systems for enantiomer production: (a) microreactor coupled with a membrane microseparator, (b) microreactor coupled with a microextractor, (c) microreactor coupled with microcapillary electrophoresis, (d) microreactor coupled with microchromatography.
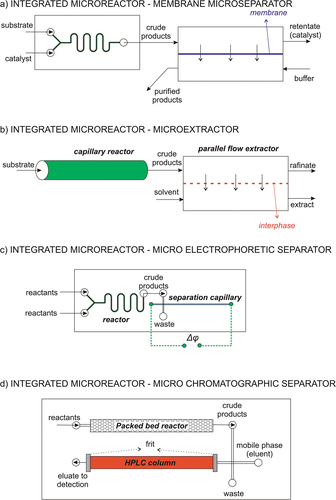
Table 4. Coupled reactor-separator microsystems for chiral synthesis and separation.
4.1. Membrane methods
The reaction product, usually of low molecular weight, synthesized in a microreactor is continuously fed into a membrane micromodule in these integrated systems. In its easiest embodiment, the separation process is based on ultrafiltration/dialysis membranes with a given molecular weight cutoff. These membranes act as molecular sieves, i.e., low-molecular-weight products cross the membranes and are collected on their permeate side. The high-molecular-weight catalysts or enzymes remain in the retentate, as shown in ). Such systems operate either in ultrafiltration[Citation73] or electrodialysis mode,[Citation79] and a valuable catalyst can also be reused.[Citation80]
Such a modular system has been reported by O’Sullivan et al.[Citation73] describing a microreactor using free enzyme transketolase as a homogeneous catalyst for the chiral synthesis of L-erythrulose and (3S)-1,3-dihydroxypentan-2-one. The reaction products were separated by a Durapore ultrafiltration membrane, with a molecular weight cutoff of 10 kDa. The membrane was incorporated into a microfluidic chip with a controlled pressure difference between the permeate and retentate sides. The ultrafiltration membrane completely rejected the valuable catalyst. However, the membrane lacked chiral selectivity to the reaction products.
A membrane with chiral activity was used by El-Feky et al.[Citation151] These authors synthesized a monolithic membrane inside a microfluidic channel by ethylene dimethacrylate (EDMA), hydroxy-methyl methacrylate (HEMA), and 2-aminoethylmethacrylate (AME) copolymerization in the presence of cyclohexanol and dodecanol as porogenic solvents. An enzyme lipase preferentially esterifying (S)-ibuprofen was immobilized in the membrane. Racemic ibuprofen, ethanol, and phosphate buffer were fed into the microfluidic module. The immobilized enzyme converted (S)-ibuprofen into (S)-ibuprofen ethyl ester, which permeated through the membrane. The permeate phase contained another free lipase hydrolyzing the ester back to (S)-ibuprofen. Huh et al.[Citation67] reported a similar principle of (S)-ibuprofen separation. Unlike El-Feky et al.,[Citation151] they used both lipases in their free form and a thin layer of hexafluorophosphate ionic liquid as the membrane for selective ester separation.
4.2. Extraction methods
Coupling a microreactor to a microextractor also allows continuous processing. Microfluidic extractors can operate in a parallel co-current arrangement even without employing any stabilizing layer. The co-current flow of the retentate and extract phases provides intensive mass transfer ()).
Honda et al.[Citation18] developed a microreactor-microextractor system for synthesizing L-amino acids. The enzyme aminoacylase enantioselectively catalyzed the formation of L-amino acids from a mixture of enantiomers of N-acetylamino acids in a capillary microreactor. After acidification, the unconverted N-acetyl-D-amino acids were selectively removed by ethyl acetate in a microextractor with parallel flow of the immiscible phases.
A more complex integrated system was reported by Grossehilmann et al.[Citation152] The reaction of ethyl pyruvate and nitromethane, according to the Henry reaction mechanism, yielded a chiral product. The reaction product underwent several steps of downstream processing. First, the product was extracted by switchable-hydrophilicity solvents on a chip with an integrated microsettler to separate immiscible liquids. Then, the product stream was stripped of carbon dioxide by argon in a falling-film microreactor in the next step. Lastly, the product was finally extracted by dichloromethane again in a mixer-settler microextractor.
4.3. Electric field-driven methods
The combinations of a microreactor with capillary electrophoresis () or chromatography ()) for enantiomer separation in integrated microdevices have also been reported. While these systems did not enable continuous enantiomer production, they showed future possibilities in this area. Currently, these methods are primarily used for efficient on-a-chip analysis of products.
Krone et al.[Citation140] presented an integrated microfluidic platform for (S)-3-phenoxy-1,2-propanediol synthesis, enantioseparation, and quantitative analysis. Escherichia coli cells expressing Aspergillus niger epoxide hydrolase were entrapped in a microfluidic reactor. The in-cell enzyme catalyzed the enantioselective transformation of 1,2-epoxy-3-phenoxypropane to the product. The product containing both (S) and (R) forms was injected into an electrophoretic microchannel made on the same chip. The enantiomers were separated in the presence of heptakis(2,3-dihydroxy-6-sulfato)-β-cyclodextrin heptasodium salt acting as a chiral selector. The integrated microfluidic chip enabled the study of stereoselective reactions on a single-cell level. This enantioselective transformation was also performed with a free enzyme.[Citation65]
This reaction strategy followed by electrophoretic separation has also been employed in organic synthesis.[Citation153] Homogeneous Pictet–Spengler reaction of dopamine with acetaldehyde using a chiral organocatalyst (R)-3,3ʹ-Bis(2,4,6-triisopropylphenyl)-1,1ʹ-binaphthyl-2,2ʹ-diyl hydrogen phosphate was performed in a microfluidic channel. The resulting enantiomers of a tetrahydroisoquinoline derivative were separated electrophoretically in the same microchip in the presence β-cyclodextrin as a chiral selector.
We believe that modern methods such as planar free flow electrophoresis (FFE) realized in microfluidic devices will allow for efficient and continuous separation of enantiomers in future. In such an arrangement, electric field is applied perpendicularly to the electrolyte flowing in a microfluidic channel. Electrolyte components are then separated into zones due to different electrophoretic mobilities.[Citation156] FFE has been used as a downstream process in a continuous-flow microfluidic system for oligopeptide production.[Citation157] FFT can be in principle used for the separation of enantiomers after adding a chiral selector.
4.4. Chromatographic methods
Anticoagulant (S)-warfarin was synthesized from 4-hydroxycoumarin and benzalacetone in the presence of (1S,2S)-1,2-diphenylethylenediamine as a catalyst in a microfluidic channel.[Citation154] The reaction mixture was then led to two HPLC microcolumns integrated into the same chip, and the reactants were separated in a reverse-phase ProntoSIL C18 column. The other column filled with Chiralpak IB microparticles served to separate the (S)- and (R)-warfarin. The products of the Michael addition performed in a microfluidic system were separated using a 2D chromatographic method.[Citation155] More specifically, (2 R,3S)-2-ethyl-4-nitro-3-phenylbutanal was prepared in a three-step process, synthesizing the product in a column filled with silica particles, functionalized by a silica-supported prolyl-peptide catalyst. The unreacted reactants and other stereoisomers were separated in two chromatography columns filled with SiO2 and Chiralpak OD-H particles, respectively. Warias et al.[Citation62] developed a microfluidic system, combining a packed-bed microreactor with a chromatographic column. An asymmetric reaction of heptanal and N-PMP-protected ethyl iminoglyoxylate, catalyzed by the Ley-Arvidsson-Yamamoto catalyst immobilized on silica particles, formed a syn-Mannich adduct. This product was separated in the integrated chromatography column containing Chiralpak IG-3 microparticles.
5. Conclusions
Over the last twenty years, technological advances in microfluidics, from its infancy to maturity, have been reflected in the synthesis and purification of optically active chemicals. By building on its characteristic features, such as fast prototyping, increased process safety, scale-up by numbering-up, or mass and heat transfer intensification, microfluidics has become an inherent chemical engineering tool for controlling reaction-transport processes in chemical production. Concurrently, microreactors have been successfully employed in the organic and enzymatic synthesis of many essential stereoisomers. These microscale reactions are characterized by large yields, conversions, and selectivities, often exceeding those obtained in conventional macro-reactors.
Microfluidic technologies offer a wide range of other chemical and physical processes. Processes such as micro-extraction, micro-adsorption, or micro-membrane separation have been seamlessly integrated with microreactors for the downstream separation and purification of synthesized products. Continuous-flow microfluidics has shown its potential for the production of special chemicals in which high yield and conversion are preferred to large quantities. Many key players in the pharmaceutical industry, such as Eli Lilly, GlaxoSmithKline, Merck, Novartis, or Bristol-Myer Squibb, invest heavily in developing versatile mini plants with continuous production because they appreciate process intensification coupled with savings on space, energy and reactants, in harmony with principles of green chemistry. In this context, manufacturing active pharmaceutical ingredients (APIs) in continuous microfluidic platforms should become a standard technology.
The synthesis of optically active compounds may also shift toward new, innovative approaches and solutions in the near future. First, new membranes for chiral resolution are being continuously developed as Materials Science advances. Chirally selective membranes have the potential to play a key role in enantiomer separation, which is very important in pharmacy to get enantiopure drugs without negative side effects. They are compatible with continuous production and can be incorporated or directly synthesized in microfluidic modules and structures. Second, membranes serve as supports of catalysts. A catalytically active layer can provide chiral resolution, or the same membrane, when functionalized with a chiral selector, can resolve a catalytically synthesized product. Third, modularity may become an intrinsic property of developing a given production process and its operation. In fact, modular systems combining reaction and membrane separation or reaction and microextraction have been reported already.
While microreaction technology has reached its maturity, membrane separation still needs further technological development and progress in both separation selectivity and throughput by understanding fundamental separation principles. This knowledge gap is highlighted when comparing the characteristics of both technologies. On the one hand, microreactors operated in a continuous mode usually reach good conversion within minutes or tens of minutes. Numbering-up offers then a possibility to scale the synthesis to the required throughput. On the other hand, membrane separations of enantiomers sometimes require hundreds of hours. Such a significant difference in the characteristic times of these two technologies cannot be solved only by adjusting the throughput by increasing the membrane area. The enantiomeric excess, which characterizes membrane separation quality, also suggests that chiral membranes must be improved before their deployment in industrial production lines. Ultimately, this multifaceted problem will require collaborative efforts between the chemists who design membranes and the engineers who use those membranes in various applications.
Acknowledgments
This work was supported by grant of Czech Science Foundation No. 20-09980S.
Disclosure statement
The author(s) declare no conflict of interest.
References
- Shekhar, C. Chirality Meets Topology. Nat. Mater. 2018, 17(11), 953–954. DOI: 10.1038/s41563-018-0210-6.
- Barron, L. D. Chirality and Life. Space Sci. Rev. 2008, 135(1–4), 187–201. DOI: 10.1007/s11214-007-9254-7.
- Kuncha, S. K.; Kruparani, S. P.; Sankaranarayanan, R. Chiral Checkpoints during Protein Biosynthesis. J. Biol. Chem. 2019, 294(45), 16535–16548. DOI: 10.1074/jbc.REV119.008166.
- Blackmond, D. G. The Origin of Biological Homochirality. Cold Spring Harbor Perspect.Biol. 2019, 11(3), a032540. DOI: 10.1101/cshperspect.a032540.
- Bentley, R. The Nose as a Stereochemist. Enantiomers and Odor. Chem. Rev. 2006, 106(9), 4099–4112. DOI: 10.1021/cr050049t.
- Leitereg, T. J.; Guadagni, D. G.; Harris, J.; Mon, T. R.; Teranishi, R. Evidence for the Difference between the Odours of the Optical Isomers (+)- and (−)-carvone. Nature. 1971, 230(5294), 455–456. DOI: 10.1038/230455a0.
- Gal, J. The Discovery of Stereoselectivity at Biological Receptors: Arnaldo Piutti and the Taste of the Asparagine Enantiomers-History and Analysis on the 125th Anniversary. Chirality. 2012, 24(12), 959–976. DOI: 10.1002/chir.22071.
- Scott, A. K. Stereoisomers and Drug Toxicity - the Value of Single Stereoisomer Therapy. Drug Safety. 1993, 8(2), 149–159. DOI: 10.2165/00002018-199308020-00005.
- Chhabra, N.; Aseri, M. L.; Padmanabhan, D. A Review of Drug Isomerism and Its Significance. Int J Appl Basic Med Res. 2013, 3(1), 16–18. DOI: 10.4103/2229-516x.112233.
- Brown, J. M.; Davies, S. G. Chemical Asymmetric-Synthesis. Nature. 1989, 342(6250), 631–636. DOI: 10.1038/342631a0.
- Miller, M. T. Thalidomide Embryopathy: A Model for the Study of Congenital Incomitant Horizontal Strabismus. Transact. Am. Ophthalmol. Soc. 1991, 89, 623–674.
- Breuer, M.; Ditrich, K.; Habicher, T.; Hauer, B.; Kesseler, M.; Sturmer, R.; Zelinski, T. Industrial Methods for the Production of Optically Active Intermediates. Angewandte Chemie International Edition. 2004, 43(7), 788–824. DOI: 10.1002/anie.200300599.
- Ren, Q.; Ruth, K.; Thony-Meyer, L.; Zinn, M. Enatiomerically Pure Hydroxycarboxylic Acids: Current Approaches and Future Perspectives. Appl. Microbiol. Biotechnol. 2010, 87(1), 41–52. DOI: 10.1007/s00253-010-2530-6.
- Ghanem, A. Trends in Lipase-catalyzed Asymmetric Access to Enantiomerically Pure/enriched Compounds. Tetrahedron. 2007, 63(8), 1721–1754. DOI: 10.1016/j.tet.2006.09.110.
- Delville, M. M. E.; Van Gool, J. J. F.; van Wijk, I. M.; Van Hest, J. C. M.; Rutjes, F. Synthesis of Methoxyisopropyl (Mip)-protected (R)-mandelonitrile and Derivatives in a Flow Reactor. J. Flow Chem. 2012, 2(4), 124–128. DOI: 10.1556/jfc-d-12-00008.
- Gruber, P.; Carvalho, F.; Marques, M. P. C.; O’Sullivan, B.; Subrizi, F.; Dobrijevic, D.; Ward, J.; Hailes, H. C.; Fernandes, P.; Wohlgemuth, R.; et al. Enzymatic Synthesis of Chiral Amino-alcohols by Coupling Transketolase and Transaminase-catalyzed Reactions in a Cascading Continuous-flow Microreactor System. Biotechnol. Bioeng. 2018, 115(3), 586–596. DOI: 10.1002/bit.26470.
- Halim, A. A.; Szita, N.; Baganz, F. Characterization and Multi-step Transketolase-omega-transaminase Bioconversions in an Immobilized Enzyme Microreactor (IEMR) with Packed Tube. J. Biotechnol. 2013, 168(4), 567–575. DOI: 10.1016/j.jbiotec.2013.09.001.
- Honda, T.; Miyazaki, M.; Yamaguchi, Y.; Nakamura, H.; Maeda, H. Integrated Microreaction System for Optical Resolution of Racemic Amino Acids. Lab Chip. 2007, 7(3), 366–372. DOI: 10.1039/b614500k.
- Matosevic, S.; Lye, G. J.; Baganz, F. Immobilised Enzyme Microreactor for Screening of Multi-step Bioconversions: Characterisation of a De Novo Transketolase-omega-transaminase Pathway to Synthesise Chiral Amino Alcohols. J. Biotechnol. 2011, 155(3), 320–329. DOI: 10.1016/j.jbiotec.2011.07.017.
- Milozic, N.; Stojkovic, G.; Vogel, A.; Bouwes, D.; Znidarsic-Plazl, P. Development of Microreactors with Surface-immobilized Biocatalysts for Continuous Transamination. New Biotechnol. 2018, 47, 18–24. DOI: 10.1016/j.nbt.2018.05.004.
- Sheldon, R. A. Biocatalytic Vs Chemical Synthesis of Enantiomerically Pure Compounds. Chimia. 1996, 50(9), 418–419.
- Dittrich, P. S.; Manz, A. Lab-on-a-chip: Microfluidics in Drug Discovery. Nat. Rev. Drug Discovery. 2006, 5(3), 210–218. DOI: 10.1038/nrd1985.
- Abou-Hassan, A.; Sandre, O.; Cabuil, V. Microfluidics in Inorganic Chemistry. Angew. Chem-Int. Ed. 2010, 49(36), 6268–6286. DOI: 10.1002/anie.200904285.
- deMello, A. J. Control and Detection of Chemical Reactions in Microfluidic Systems. Nature. 2006, 442(7101), 394–402. DOI: 10.1038/nature05062.
- Whitesides, G. M. The Origins and the Future of Microfluidics. Nature. 2006, 442(7101), 368–373. DOI: 10.1038/nature05058.
- de Jong, J.; Lammertink, R. G. H.; Wessling, M. Membranes and Microfluidics: A Review. Lab Chip. 2006, 6(9), 1125–1139. DOI: 10.1039/b603275c.
- Lin, W. Y.; Wang, Y. J.; Wang, S. T.; Tseng, H. R. Integrated Microfluidic Reactors. Nano Today. 2009, 4(6), 470–481. DOI: 10.1016/j.nantod.2009.10.007.
- Makgwane, P. R.; Ray, S. S. Synthesis of Nanomaterials by Continuous-Flow Microfluidics: A Review. J. Nanosci. Nanotechnol. 2014, 14(2), 1338–1363. DOI: 10.1166/jnn.2014.9129.
- Maier, N. M.; Franco, P.; Lindner, W. Separation of Enantiomers: Needs, Challenges, Perspectives. J. Chromatogr. A. 2001, 906(1–2), 3–33. DOI: 10.1016/S0021-9673(00)00532-X.
- Abe, K.; Goto, M.; Nakashio, F. Novel Optical Resolution of Phenylalanine Racemate Utilizing Enzyme Reaction and Membrane Extraction. Sep. Sci. Technol. 1997, 32(11), 1921–1935. DOI: 10.1080/01496399708000745.
- Hsu, L. C.; Kim, H.; Yang, X.; Ross, D. Large Scale Chiral Chromatography for the Separation of an Enantiomer to Accelerate Drug Development. Chirality. 2011, 23(4), 361–366. DOI: 10.1002/chir.20931.
- Pinto, M. M. M.; Fernandes, C.; Tiritan, M. E. Chiral Separations in Preparative Scale: A Medicinal Chemistry Point of View. Molecules. 2020, 25(8), 1931. DOI: 10.3390/molecules25081931.
- Wu, D.; Pan, F.; Tan, W.; Gao, L.; Tao, Y.; Kong, Y. Recent Progress of Enantioseparation under Scale Production (2014–2019). J. Sep. Sci. 2020, 43(1), 337–347. DOI: 10.1002/jssc.201900682.
- van der Ent, E. M.; Thielen, T. P. H.; Cohen Stuart, M. A.; van der Padt, A.; Keurentjes, J. T. F. Electrodialysis System for Large-Scale Enantiomer Separation. Ind. Eng. Chem. Res. 2001, 40(25), 6021–6027. DOI: 10.1021/ie0103759.
- Praveen, C. Dexterity of Gold Catalysis in Controlling the Regioselectivity of Cycloaddition Reactions. Catal. Rev.-Sci. Eng. 2019, 61(3), 406–446. DOI: 10.1080/01614940.2019.1594016.
- Wu, Y. H.; Zhang, L. Y.; Wang, N. X.; Xing, Y. L. Recent Advances in the Rare-earth Metal Triflates-catalyzed Organic Reactions. Catal. Rev.-Sci. Eng. 2020, 1–37. DOI: 10.1080/01614940.2020.1831758.
- Appun, J.; Boomhoff, M.; Hoffmeyer, P.; Kallweit, I.; Pahl, M.; Belder, D.; Schneider, C. A Highly Stereoselective Synthesis of Tetrahydrofurans. Angew. Chem-Int. Ed. 2017, 56(24), 6758–6761. DOI: 10.1002/anie.201700774.
- Dai, W.; Mi, Y.; Lv, Y.; Chen, B.; Li, G. S.; Chen, G. W.; Gao, S. Development of a Continuous-Flow Microreactor for Asymmetric Sulfoxidation Using a Biomimetic Manganese Catalyst. Adv. Synth. Catal. 2016, 358(4), 667–671. DOI: 10.1002/adsc.201501023.
- Dai, W.; Mi, Y.; Lv, Y.; Shang, S. S.; Li, G. S.; Chen, G. W.; Gao, S. Development of a Continuous-Flow Microreactor for Asymmetric Epoxidation of Electron-Deficient Olefins. Synthesis-Stuttgart. 2016, 48(16), 2653–2658. DOI: 10.1055/s-0035-1561955.
- De Angelis, S.; De Renzo, M.; Carlucci, C.; Degennaro, L.; Luisi, R. A Convenient Enantioselective CBS-reduction of Arylketones in Flow-microreactor Systems. Org. Biomol. Chem. 2016, 14(18), 4304–4311. DOI: 10.1039/c6ob00336b.
- Hayashi, T.; Kikuchi, S.; Asano, Y.; Endo, Y.; Yamada, T. Homogeneous Enantioselective Catalysis in a Continuous-Flow Microreactor: Highly Enantioselective Borohydride Reduction of Ketones Catalyzed by Optically Active Cobalt Complexes. Org. Process Res. Dev. 2012, 16(6), 1235–1240. DOI: 10.1021/op300061k.
- Chiroli, V.; Benaglia, M.; Puglisi, A.; Porta, R.; Jumde, R. P.; Mandoli, A. A Chiral Organocatalytic Polymer-based Monolithic Reactor. Green Chem. 2014, 16(5), 2798–2806. DOI: 10.1039/c4gc00031e.
- Kasaplar, P.; Rodriguez-Escrich, C.; Pericas, M. A. Continuous Flow, Highly Enantioselective Michael Additions Catalyzed by a PS-Supported Squaramide. Org. Lett. 2013, 15(14), 3498–3501. DOI: 10.1021/ol400974z.
- Kluson, P.; Stavarek, P.; Penkavova, V.; Vychodilova, H.; Hejda, S.; Bendova, M. Microfluidic Chip Reactor and the Stereoselective Hydrogenation of Methylacetoacetate over (R)-ru-binap in the N-8222 Tf2N /Methanol/water Mixed Phase. Chem Eng Processing-Process Intensif. 2017, 115, 39–45. DOI: 10.1016/j.cep.2017.02.002.
- Kluson, P.; Stavarek, P.; Penkavova, V.; Vychodilova, H.; Hejda, S.; Jaklova, N.; Curinova, P. Stereoselective Synthesis of Optical Isomers of Ethyl 4-chloro-3-hydroxybutyrate in a Microfluidic Chip Reactor. J. Flow Chem. 2019, 9(4), 221–230. DOI: 10.1007/s41981-019-00043-y.
- Laudadio, G.; Gemoets, H. P. L.; Hessel, V.; Noel, T. Flow Synthesis of Diaryliodonium Triflates. J. Org. Chem. 2017, 82(22), 11735–11741. DOI: 10.1021/acs.joc.7b01346.
- Liu, H. L.; Feng, J.; Zhang, J. Y.; Miller, P. W.; Chen, L. P.; Su, C. Y. A Catalytic Chiral Gel Microfluidic Reactor Assembled via Dynamic Covalent Chemistry. Chem. Sci. 2015, 6(4), 2292–2296. DOI: 10.1039/c5sc00314h.
- Nishiyama, Y.; Mori, R.; Nishida, K.; Tanimoto, H.; Morimoto, T.; Kakiuchi, K. Diastereodifferentiating 2+2 Photocycloaddition of a Chiral Cyclohexenone with Cyclopentene in Supercritical Carbon Dioxide Using a Flow Microreactor. J. Flow Chem. 2014, 4(4), 185–189. DOI: 10.1556/jfc-d-14-00012.
- Pirola, M.; Compostella, M. E.; Raimondi, L.; Puglisi, A.; Benaglia, M. A Continuous-Flow, Two-Step, Metal-Free Process for the Synthesis of Differently Substituted Chiral 1,2-Diamino Derivatives. Synthesis-Stuttgart. 2018, 50(7), 1430–1438. DOI: 10.1055/s-0036-1591911.
- Rossi, S.; Porta, R.; Brenna, D.; Puglisi, A.; Benaglia, M. Stereoselective Catalytic Synthesis of Active Pharmaceutical Ingredients in Homemade 3D-Printed Mesoreactors. Angew. Chem-Int. Ed. 2017, 56(15), 4290–4294. DOI: 10.1002/anie.201612192.
- Sandel, S.; Weber, S. K.; Trapp, O. Oxidations with Bonded Salen-catalysts in Microcapillaries. Chem. Eng. Sci. 2012, 83, 171–179. DOI: 10.1016/j.ces.2011.10.034.
- Shu, W.; Buchwald, S. L. Enantioselective ss-Arylation of Ketones Enabled by Lithiation/Borylation/1,4-Addition Sequence under Flow Conditions. Angew. Chem-Int. Ed. 2012, 51(22), 5355–5358. DOI: 10.1002/anie.201202221.
- Schober, L.; Ratnam, S.; Yamashita, Y.; Adebar, N.; Pieper, M.; Berkessel, A.; Hessel, V.; Groger, H. An Asymmetric Organocatalytic Aldol Reaction of a Hydrophobic Aldehyde in Aqueous Medium Running in Flow Mode. Synthesis-Stuttgart. 2019, 51(5), 1178–1184. DOI: 10.1055/s-0037-1610404.
- Solodenko, W.; Jas, G.; Kunz, U.; Kirschning, A. Continuous Enantioselective Kinetic Resolution of Terminal Epoxides Using Immobilized Chiral Cobalt-salen Complexes. Synthesis-Stuttgart. 2007, 4, 583–589. doi: 10.1055/s-2007-965877.
- Tanaka, D.; Kawakubo, W.; Tsuda, E.; Mitsumoto, Y.; Yoon, D. H.; Sekiguchi, T.; Akitsu, T.; Shoji, S. Microfluidic Synthesis of Chiral Salen Mn(II) and Co(II) Complexes Containing Lysozyme. RSC Adv. 2016, 6(85), 81862–81868. DOI: 10.1039/c6ra09975k.
- Tang, X. F.; Zhao, J. N.; Wu, Y. F.; Zheng, Z. H.; Ma, C. F.; Yu, Z. Y.; Yun, L.; Liu, G. Z.; Meng, Q. W. Asymmetric Alpha-hydroxylation Of Beta-dicarbonyl Compounds By C-2 ‘ modified cinchonine-derived phase-transfer catalysts in batch and flow microreactors. Synth. Commun. 2020, 50(16), 2478–2487. DOI: 10.1080/00397911.2020.1781183.
- Terao, K.; Nishiyama, Y.; Aida, S.; Tanimoto, H.; Morimoto, T.; Kakiuchi, K. Diastereodifferentiating 2+2 Photocycloaddition of Chiral Cyclohexenone Carboxylates with Cyclopentene by a Microreactor. J. Photochem. Photobiol. a-Chem. 2012, 242, 13–19. DOI: 10.1016/j.jphotochem.2012.05.021.
- Tibhe, J. D.; Fu, H.; Noel, T.; Wang, Q.; Meuldijk, J.; Hessel, V. Flow Synthesis of Phenylserine Using Threonine Aldolase Immobilized on Eupergit Support. Beilstein J. Org. Chem. 2013, 9, 2168–2179. DOI: 10.3762/bjoc.9.254.
- Tomida, Y.; Nagaki, A.; Yoshida, J. Asymmetric Carbolithiation of Conjugated Enynes: A Flow Microreactor Enables the Use of Configurationally Unstable Intermediates before They Epimerize. J. Am. Chem. Soc. 2011, 133(11), 3744–3747. DOI: 10.1021/ja110898s.
- Vile, G.; Schmidt, G.; Richard-Bildstein, S.; Abele, S. Enantiospecific Cyclization of Methyl N-(tert-butoxycarbonyl)-N-(3-chloropropyl)-D-alaninate to 2-methylproline Derivative via ‘Memory of Chirality’ in Flow. J. Flow Chem. 2019, 9(1), 19–25. DOI: 10.1007/s41981-018-0022-5.
- Wang, Y. F.; Jiang, Z. H.; Chu, M. M.; Qi, S. S.; Yin, H.; Han, H. T.; Xu, D. Q. Asymmetric Copper-catalyzed Fluorination of Cyclic Beta-keto Esters in a Continuous-flow Microreactor. Org. Biomol. Chem. 2020, 18(26), 4927–4931. DOI: 10.1039/d0ob00588f.
- Warias, R.; Zaghi, A.; Heiland, J. J.; Piendl, S. K.; Gilmore, K.; Seeberger, P. H.; Massi, A.; Belder, D. An Integrated Lab-on-a-chip Approach to Study Heterogeneous Enantioselective Catalysts at the Microscale. Chemcatchem. 2018, 10(23), 5382–5385. DOI: 10.1002/cctc.201801637.
- Yoo, C. J.; Rackl, D.; Liu, W. B.; Hoyt, C. B.; Pimentel, B.; Lively, R. P.; Davies, H. M. L.; Jones, C. W. An Immobilized-Dirhodium Hollow-Fiber Flow Reactor for Scalable and Sustainable C-H Functionalization in Continuous Flow. Angew. Chem-Int. Ed. 2018, 57(34), 10923–10927. DOI: 10.1002/anie.201805528.
- Bartha-Vari, J. H.; Tosa, M. I.; Irimie, F. D.; Weiser, D.; Boros, Z.; Vertessy, B. G.; Paizs, C.; Poppe, L. Immobilization of Phenylalanine Ammonia-Lyase on Single-Walled Carbon Nanotubes for Stereoselective Biotransformations in Batch and Continuous-Flow Modes. Chemcatchem. 2015, 7(7), 1122–1128. DOI: 10.1002/cctc.201402894.
- Belder, D.; Ludwig, M.; Wang, L. W.; Reetz, M. T. Enantioselective Catalysis and Analysis on a Chip. Angew. Chem-Int. Ed. 2006, 45(15), 2463–2466. DOI: 10.1002/anie.200504205.
- Cech, J.; Hessel, V.; Pribyl, M. Aldolase Catalyzed L-phenylserine Synthesis in a Slug-flow Microfluidic System - Performance and Diastereoselectivity Studies. Chem. Eng. Sci. 2017, 169, 97–105. DOI: 10.1016/j.ces.2016.08.033.
- Huh, Y. S.; Jun, Y. S.; Hong, Y. K.; Hong, W. H.; Kim, D. H. Microfluidic Separation of (S)-ibuprofen Using Enzymatic Reaction. J. Mol. Catal. B Enzym. 2006, 43(1–4), 96–101. DOI: 10.1016/j.molcatb.2006.06.017.
- Cheikhou, K.; Tzedakis, T. Electrochemical Microreactor for Chiral Syntheses Using the Cofactor NADH. AIChE J. 2008, 54(5), 1365–1376. DOI: 10.1002/aic.11463.
- Kataoka, S.; Takeuchi, Y.; Harada, A.; Yamada, M.; Endo, A. Microreactor with Mesoporous Silica Support Layer for Lipase Catalyzed Enantioselective Transesterification. Green Chem. 2010, 12(2), 331–337. DOI: 10.1039/b917374a.
- Kawakami, K.; Ueno, M.; Takei, T.; Oda, Y.; Takahashi, R. Application of a Burkholderia Cepacia Lipase-immobilized Silica Monolith Micro-bioreactor to Continuous-flow Kinetic Resolution for Transesterification of (R, S)-1-phenylethanol. Process Biochem. 2012, 47(1), 147–150. DOI: 10.1016/j.procbio.2011.09.017.
- Koch, K.; Van Den Berg, R. J. F.; Nieuwland, P. J.; Wijtmans, R.; Schoemaker, H. E.; Van Hest, J. C. M.; Rutjes, F. Enzymatic Enantioselective C-C-bond Formation in Microreactors. Biotechnol. Bioeng. 2008, 99(4), 1028–1033. DOI: 10.1002/bit.21649.
- Koch, K.; Van Den Berg, R. J. F.; Nieuwland, P. J.; Wijtmans, R.; Wubbolts, M. G.; Schoemaker, H. E.; Rutjes, F.; Van Hest, J. C. M. Enzymatic Synthesis of Optically Pure Cyanohydrins in Microchannels Using a Crude Cell Lysate. Chem. Eng. J. 2008, 135, S89–S92. DOI: 10.1016/j.cej.2007.07.013.
- O’Sullivan, B.; Al-Bahrani, H.; Lawrence, J.; Campos, M.; Cazares, A.; Baganz, F.; Wohlgemuth, R.; Hailes, H. C.; Szita, N. Modular Microfluidic Reactor and Inline Filtration System for the Biocatalytic Synthesis of Chiral Metabolites. J. Mol. Catal. B Enzym. 2012, 77, 1–8. DOI: 10.1016/j.molcatb.2011.12.010.
- Van Der Helm, M. P.; Bracco, P.; Busch, H.; Szymanska, K.; Jarzebski, A. B.; Hanefeld, U. Hydroxynitrile Lyases Covalently Immobilized in Continuous Flow Microreactors. Catal. Sci. Technol. 2019, 9(5), 1189–1200. DOI: 10.1039/c8cy02192a.
- Weiser, D.; Bencze, L. C.; Banoczi, G.; Ender, F.; Kiss, R.; Kokai, E.; Szilagyi, A.; Vertessy, B. G.; Farkas, O.; Paizs, C.; et al. Phenylalanine Ammonia-Lyase-Catalyzed Deamination of an Acyclic Amino Acid: Enzyme Mechanistic Studies Aided by a Novel Microreactor Filled with Magnetic Nanoparticles. Chembiochem. 2015, 16(16), 2283–2288. DOI: 10.1002/cbic.201500444.
- Zhang, M.; Ettelaie, R.; Yan, T.; Zhang, S. J.; Cheng, F. Q.; Binks, B. P.; Yang, H. Q. Ionic Liquid Droplet Microreactor for Catalysis Reactions Not at Equilibrium. J. Am. Chem. Soc. 2017, 139(48), 17387–17396. DOI: 10.1021/jacs.7b07731.
- Gao, W. C.; Xiong, Z. Y.; Pirhaghani, S.; Wirth, T. Enantioselective Electrochemical Lactonization Using Chiral Iodoarenes as Mediators. Synthesis-Stuttgart. 2019, 51(1), 276–284. DOI: 10.1055/s-0037-1610373.
- Cech, J.; Pribyl, M.; Snita, D. Three-phase Slug Flow in Microchips Can Provide Beneficial Reaction Conditions for Enzyme Liquid-liquid Reactions. Biomicrofluidics. 2013, 7(5), 054103. DOI: 10.1063/1.4821168.
- Romanov, A.; Slouka, Z.; Pribyl, M. Electric-field-enhanced Selective Separation of Products of an Enzymatic Reaction in a Membrane Micro-contactor. Biotechnol. Bioeng. 2021, 118(2), 715–724. DOI: 10.1002/bit.27597.
- Vobecka, L.; Ticha, L.; Atanasova, A.; Slouka, Z.; Hasal, P.; Pribyl, M. Enzyme Synthesis of Cephalexin in Continuous-flow Microfluidic Device in ATPS Environment. Chem. Eng. J. 2020, 396, 125236. DOI: 10.1016/j.cej.2020.125236.
- Cech, J.; Schrott, W.; Slouka, Z.; Pribyl, M.; Broz, M.; Kuncova, G.; Snita, D. Enzyme Hydrolysis of Soybean Oil in a Slug Flow Microsystem. Biochem. Eng. J. 2012, 67, 194–202. DOI: 10.1016/j.bej.2012.06.015.
- Zhang, Y.; Zhang, Y.; Ramstrom, O. Dynamic Covalent Kinetic Resolution. Catal. Rev.-Sci. Eng. 2020, 62(1), 66–95. DOI: 10.1080/01614940.2019.1664031.
- Gholami, Z.; Tisler, Z.; Rubas, V. Recent Advances in Fischer-Tropsch Synthesis Using Cobalt-based Catalysts: A Review on Supports, Promoters, and Reactors. Catal. Rev.-Sci. Eng. 2020, 1–84. DOI: 10.1080/01614940.2020.1762367.
- Britton, J.; Majumdar, S.; Weiss, G. A. Continuous Flow Biocatalysis. Chem. Soc. Rev. 2018, 47(15), 5891–5918. DOI: 10.1039/c7cs00906b.
- Calcaterra, A.; D’Acquarica, I. The Market of Chiral Drugs: Chiral Switches versus De Novo Enantiomerically Pure Compounds. J. Pharm. Biomed. Anal. 2018, 147, 323–340. DOI: 10.1016/j.jpba.2017.07.008.
- Smith, S. W. Chiral Toxicology: It’s the Same Thing … Only Different. Toxicol. Sci. 2009, 110(1), 4–30. DOI: 10.1093/toxsci/kfp097.
- Evans, C. H.; Mankin, H. J.; Ferguson, A. B.; Robbins, P. D.; Ghivizzani, S. C.; Herndon, J. H.; Kang, R.; Tomaino, M. M.; Wright, T. M. Clinical Trial to Assess the Safety, Feasibility, and Efficacy of Transferring a Potentially Anti-arthritic Cytokine Gene to Human Joints with Rheumatoid Arthritis. Hum. Gene Ther. 1996, 7(10), 1261–1280. DOI: 10.1089/hum.1996.7.10-1261.
- Tsai, R. K.; Lee, Y. H. Reversibility of Ethambutol Optic Neuropathy. J. Ocul. Pharmacol. Ther. 1997, 13(5), 473–477. DOI: 10.1089/jop.1997.13.473.
- Vargesson, N. Thalidomide-induced Teratogenesis: History and Mechanisms. Birth Defect. Res. Part C-Embryo Today-Rev. 2015, 105(2), 140–156. DOI: 10.1002/bdrc.21096.
- Albert, K. S.; Gernaat, C. M. Pharmacokinetics of Ibuprofen. Am. J. Med. 1984, 77(1A), 40–46. DOI: 10.1016/s0002-9343(84)80017-0.
- Burke, W. J.; Kratochvil, C. J. Stereoisomers in Psychiatry: The Case of Escitalopram. Prim. Care Compan. J. Clin. Psychiatry. 2002, 4(1), 20–24. DOI: 10.4088/pcc.v04n0107.
- Ignarro, L. J. Different Pharmacological Properties of Two Enantiomers in a Unique Beta-blocker, Nebivolol. Cardiovascul. Therap. 2008, 26(2), 115–134. DOI: 10.1111/j.1527-3466.2008.00044.x.
- da Cunha, L. C.; Gondim, F. A.; de Paola, A. A.; Barros, I. C.; Santos, S. R. Kinetic Disposition of (+)-S- and (-)-r-sotalol Enantiomers in Cardiac Patients with Tachyarrhythmias Using an Improved HPLC-fluorescence Stereoselective Method. Boll. Chim. Farm. 2002, 141(1), 45–51.
- Kean, W. F.; Lock, C. J. L.; Rischke, J.; Butt, R.; Buchanan, W. W.; Howardlock, H. Effect of R-enantiomer and S-enantiomer of Naproxen on Aggregation and Thromboxane Production in Human-platelets. J. Pharm. Sci. 1989, 78(4), 324–327. DOI: 10.1002/jps.2600780413.
- Dorszewska, J.; Prendecki, M.; Lianeri, M.; Kozubski, W. Molecular Effects of L-dopa Herapy in Parkinson’s Disease. Curr. Genomics. 2014, 15(1), 11–17. DOI: 10.2174/1389202914666131210213042.
- Scriba, G. K. Chiral Recognition in Separation Science–an Update. J. Chromatogr. A. 2016, 1467, 56–78. DOI: 10.1016/j.chroma.2016.05.061.
- Zaera, F. Chirality in Adsorption on Solid Surfaces. Chem. Soc. Rev. 2017, 46(23), 7374–7398. DOI: 10.1039/C7CS00367F.
- Powell, M. E.; Evans, C. D.; Bull, S. D.; James, T. D.; Fordred, P. S. 8.29 Spectroscopic Analysis: Diastereomeric Derivatization for Spectroscopy. In Comprehensive Chirality; Carreira, E. M., Yamamoto, H., Eds.; Elsevier: Amsterdam, 2012; pp 571–599.
- Patti, A. Methods for the Preparation of Optically Active Chiral Compounds. In Green Approaches To Asymmetric Catalytic Synthesis; Springer Netherlands: Dordrecht, 2011; pp 1–27.
- Fernandes, C.; Tiritan, M. E.; Pinto, M. M. M. Chiral Separation in Preparative Scale: A Brief Overview of Membranes as Tools for Enantiomeric Separation. Symmetry. 2017, 9(10), 206. DOI: 10.3390/sym9100206.
- García Doménech, N.; Purcell-Milton, F.; Gun’ko, Y. K. Recent Progress and Future Prospects in Development of Advanced Materials for Nanofiltration. Mater. Today Commun. 2020, 23, 100888. DOI: 10.1016/j.mtcomm.2019.100888.
- Rautenbach, R.; Albrecht, R. Membrane Separation Processes; J. Wiley & Sons: Chichester, 1989.
- Mulder, M. Basic Principles of Membrane Technology; Kluwer Academic Publishers: Dordrecht, 1991.
- Baker, R. W. Membrane Technology and Applications; John Wiley & Sons: Chichester, 2004.
- Strathmann, H. Membrane Separation Processes. J. Membr. Sci. 1981, 9(1), 121–189. DOI: 10.1016/S0376-7388(00)85121-2.
- Slouka, Z.; Senapati, S.; Chang, H.-C. Microfluidic Systems with Ion-Selective Membranes. Ann. Rev. Analyt. Chem. 2014, 7(1), 317–335. DOI: 10.1146/annurev-anchem-071213-020155.
- Xie, R.; Chu, L.-Y.; Deng, J.-G. Membranes and Membrane Processes for Chiral Resolution. Chem. Soc. Rev. 2008, 37(6), 1243–1263. DOI: 10.1039/B713350B.
- Kim, D.; Judy, J. W. Analysis of Donnan-dialyzer Irreproducibility and Experimental Study of a Microfluidic Parallel-plate Membrane-separation Module for Total Analysis Systems. J. Membr. Sci. 2014, 460, 148–159. DOI: 10.1016/j.memsci.2014.02.029.
- Anand, D.; Dhoke, G. V.; Gehrmann, J.; Garakani, T. M.; Davari, M. D.; Bocola, M.; Zhu, L.; Schwaneberg, U. Chiral Separation of D/l-arginine with Whole Cells through an Engineered FhuA Nanochannel. Chem. Commun. 2019, 55(38), 5431–5434. DOI: 10.1039/C9CC00154A.
- Ingole, P. G.; Bajaj, H. C.; Singh, K. Membrane Separation Processes: Optical Resolution of Lysine and Asparagine Amino Acids. Desalination. 2014, 343, 75–81. DOI: 10.1016/j.desal.2013.10.009.
- Abbasi, A.; Rahbar-Kelishami, A.; Ghasemi, M. J. Development of a Microfluidic-chip System Based on Parallel Flow for Intensified Gd(III) Extraction from Nitrate Media Using Cationic Extractant. J. Rare Earths. 2018, 36(11), 1198–1204. DOI: 10.1016/j.jre.2018.03.024.
- Bryjak, M.; Kozłowski, J.; Wieczorek, P.; Kafarski, P. Enantioselective Transport of Amino Acid through Supported Chiral Liquid Membranes. J. Membr. Sci. 1993, 85(3), 221–228. DOI: 10.1016/0376-7388(93)85276-3.
- Miyako, E.; Maruyama, T.; Kubota, F.; Kamiya, N.; Goto, M. Optical Resolution of Various Amino Acids Using a Supported Liquid Membrane Encapsulating a Surfactant−Protease Complex. Langmuir. 2005, 21(10), 4674–4679. DOI: 10.1021/la046789z.
- Murai, Y.; Yoshikawa, M. Polymeric Pseudo-liquid Membranes from Poly(dodecyl Methacrylate): KCl Transport and Optical Resolution. Polym. J. 2013, 45(10), 1058–1063. DOI: 10.1038/pj.2013.30.
- Aoki, T.; Maruyama, A.; Shinohara, K.-I.; Oikawa, E. Optical Resolution by Use of Surface-Modified Poly(methyl Methacrylate) Membrane Containing (–)-oligo{methyl(10-pinanyl)siloxane}. Polym. J. 1995, 27(5), 547–550. DOI: 10.1295/polymj.27.547.
- Aoki, T.; Shinohara, K. I.; Oikawa, E. Optical Resolution through the Solid Membrane from (+)‐poly {1‐[dimethyl (10‐pinanyl) Silyl]‐1‐propyne}. Die Makromolekulare Chemie, Rapid Communicat. 1992, 13(12), 565–570. DOI: 10.1002/marc.1992.030131206.
- Yuan, L. M.; Ma, W.; Xu, M.; Zhao, H. L.; Li, Y. Y.; Wang, R. L.; Duan, A. H.; Ai, P.; Chen, X. X. Optical Resolution and Mechanism Using Enantioselective Cellulose, Sodium Alginate and hydroxypropyl‐β‐cyclodextrin Membranes. Chirality. 2017, 29(6), 315–324. DOI: 10.1002/chir.22693.
- Singh, K.; Bajaj, H. Optical Resolution of Racemic Tryptophan through Non-chiral Membranes by Ultrafiltration Using Chiral Selector in Solution. Indian J. Chem. Technol. 2007, 14(6), 547–551.
- Schuur, B.; Verkuijl, B. J. V.; Minnaard, A. J.; De Vries, J. G.; Heeres, H. J.; Feringa, B. L. Chiral Separation by Enantioselective Liquid-liquid Extraction. Org. Biomol. Chem. 2011, 9(1), 36–51. DOI: 10.1039/c0ob00610f.
- Armstrong, D. W.; Jin, H. L. Enrichment of Enantiomers and Other Isomers with Aqueous Liquid Membranes Containing Cyclodextrin Carriers. Anal. Chem. 1987, 59(18), 2237–2241. DOI: 10.1021/ac00145a005.
- Wang, J. F.; Luo, J. Q.; Feng, S. C.; Li, H. R.; Wan, Y. H.; Zhang, X. P. Recent Development of Ionic Liquid Membranes. Green Energy Environ. 2016, 1(1), 43–61. DOI: 10.1016/j.gee.2016.05.002.
- Izák, P.; Kragl, U.; Köckerling, M. Multiphase Membrane. DE 10 2006 024 397 2006.
- Izak, P.; Ruth, W.; Fei, Z.; Dyson, P. J.; Kragl, U. Selective Removal of Acetone and Butan-1-ol from Water with Supported Ionic Liquid-polydimethylsiloxane Membrane by Pervaporation. Chem. Eng. J. 2008, 139(2), 318–321. DOI: 10.1016/j.cej.2007.08.001.
- Izak, P.; Bobbink, F. D.; Hulla, M.; Klepic, M.; Friess, K.; Hovorka, S.; Dyson, P. J. Catalytic Ionic-Liquid Membranes: The Convergence of Ionic-Liquid Catalysis and Ionic-Liquid Membrane Separation Technologies. Chempluschem. 2018, 83(1), 7–18. DOI: 10.1002/cplu.201700293.
- Wang, S. F.; Wu, Y. Z.; Zhang, N.; He, G. W.; Xin, Q. P.; Wu, X. Y.; Wu, H.; Cao, X. Z.; Guiver, M. D.; Jiang, Z. Y. A Highly Permeable Graphene Oxide Membrane with Fast and Selective Transport Nanochannels for Efficient Carbon Capture. Energy Environ. Sci. 2016, 9(10), 3107–3112. DOI: 10.1039/c6ee01984f.
- Hoek, E. M. V.; Tarabara, V. V. Encyclopedia of Membrane Science and Technology; Hoboken: John Wiley & Sons, 2013.
- Xie, S.-M.; Wang, W.-F.; Ai, P.; Yang, M.; Yuan, L.-M. Chiral Separation of (R,s)-2-phenyl-1-propanol through Cellulose Acetate Butyrate Membranes. J. Membr. Sci. 2008, 321(2), 293–298. DOI: 10.1016/j.memsci.2008.05.011.
- van der Ent, E. M.; van’t Riet, K.; Keurentjes, J. T. F.; van der Padt, A. Design Criteria for Dense Permeation-selective Membranes for Enantiomer Separations. J. Membr. Sci. 2001, 185(2), 207–221. DOI: 10.1016/S0376-7388(00)00647-5.
- Aoki, T.; Shinohara, K.-I.; Kaneko, T.; Oikawa, E. Macromolecular Design for Optical Resolution Membrane. J. Synthetic Org. Chem., Japan. 1996, 54(6), 525–536. DOI: 10.5059/yukigoseikyokaishi.54.525.
- Higuchi, A.; Ishida, Y.; Nakagawa, T. Surface Modified Polysulfone Membranes: Separation of Mixed Proteins and Optical Resolution of Tryptophan. Desalination. 1993, 90(1), 127–136. DOI: 10.1016/0011-9164(93)80170-R.
- Nakamura, M.; Kiyohara, S.; Saito, K.; Sugita, K.; Sugo, T. Chiral Separation of Dl-tryptophan Using Porous Membranes Containing Multilayered Bovine Serum Albumin Crosslinked with Glutaraldehyde. J. Chromatogr. A. 1998, 822(1), 53–58. DOI: 10.1016/S0021-9673(98)00501-9.
- Yuan, L.; Su, Y.; Duan, A.; Zheng, Y.; Ai, P.; Chen, X. Optical Resolution of D, L-Phenylglycine and Chiral Separation Mechanism Using an Enantioselective Membrane of Vancomycin. Chem. J. Chin. Univ. 2016, 37(11), 1960–1965. DOI: 10.7503/cjcu20160460.
- Liu, L.; Zang, Y.; Hadano, S.; Aoki, T.; Teraguchi, M.; Kaneko, T.; Namikoshi, T. New Achiral Phenylacetylene Monomers Having an Oligosiloxanyl Group Most Suitable for Helix-Sense-Selective Polymerization and for Obtaining Good Optical Resolution Membrane Materials. Macromolecules. 2010, 43(22), 9268–9276. DOI: 10.1021/ma101999k.
- Keating, J. J.; Bhattacharya, S.; Belfort, G. Separation of D, L-amino Acids Using Ligand Exchange Membranes. J. Membr. Sci. 2018, 555, 30–37. DOI: 10.1016/j.memsci.2018.03.030.
- Michaels, A. S. Membranes, Membrane Processes, and Their Applications - Needs, Unsolved Problems, and Challenges of the 1990s. Desalination. 1990, 77(1–3), 5–34. DOI: 10.1016/0011-9164(90)85018-6.
- Hadik, P.; Szabó, L.-P.; Nagy, E. D,L-lactic Acid and D,L-alanine Enantioseparation by Membrane Process. Desalination. 2002, 148(1), 193–198. DOI: 10.1016/S0011-9164(02)00697-5.
- Hadik, P.; Szabó, L. P.; Nagy, E.; Farkas, Z. Enantioseparation of D,L-lactic Acid by Membrane Techniques. J. Membr. Sci. 2005, 251(1), 223–232. DOI: 10.1016/j.memsci.2004.10.044.
- Ingole, P. G.; Ingole, N. P. Methods for Separation of Organic and Pharmaceutical Compounds by Different Polymer Materials. Korean J. Chem. Eng. 2014, 31(12), 2109–2123. DOI: 10.1007/s11814-014-0284-z.
- Kim, J. H.; Kim, J. H.; Jegal, J.; Lee, K.-H. Optical Resolution of α-amino Acids through Enantioselective Polymeric Membranes Based on Polysaccharides. J. Membr. Sci. 2003, 213(1), 273–283. DOI: 10.1016/S0376-7388(02)00534-3.
- Krone, K. M.; Warias, R.; Ritter, C.; Li, A. T.; Acevedo-Rocha, C. G.; Reetz, M. T.; Beldert, D. Analysis of Enantioselective Biotransformations Using a Few Hundred Cells on an Integrated Microfluidic Chip. J. Am. Chem. Soc. 2016, 138(7), 2102–2105. DOI: 10.1021/jacs.5b12443.
- Singh, K.; Devi, S.; Bajaj, H. C.; Ingole, P.; Choudhari, J.; Bhrambhatt, H. Optical Resolution of Racemic Mixtures of Amino Acids through Nanofiltration Membrane Process. Sep. Sci. Technol. 2014, 49(17), 2630–2641. DOI: 10.1080/01496395.2014.911023.
- Ingole, P. G.; Singh, K.; Bajaj, H. C. Enantioselective Polymeric Composite Membrane for Optical Resolution of Racemic Mixtures of α-amino Acids. Sep. Sci. Technol. 2011, 46(12), 1898–1907. DOI: 10.1080/01496395.2011.585625.
- Singh, K.; Ingole, P. G.; Bajaj, H. C.; Bhattacharya, A.; Brahmbhatt, H. R. Optical Resolution of α-Amino Acids by Reverse Osmosis Using Enantioselective Polymer Membrane Containing Chiral Metal-Schiff Base Complex. Sep. Sci. Technol. 2010, 45(10), 1374–1384. DOI: 10.1080/01496391003758028.
- Gaálová, J.; Yalcinkaya, F.; Cuřínová, P.; Kohout, M.; Stibor, I.; Izák, P. Composite chiral membrane, preparation method and methods of enrichment of mixtures of enantiomers. CZ 308513, 2020.
- Hadik, P.; Kotsis, L.; Eniszné-Bódogh, M.; Szabó, L.-P.; Nagy, E. Lactic Acid Enantioseparation by Means of Porous Ceramic Disc and Hollow Fiber Organic Membrane. Sep. Purif. Technol. 2005, 41(3), 299–304. DOI: 10.1016/j.seppur.2004.03.020.
- Gaálová, J.; Yalcinkaya, F.; Cuřínová, P.; Kohout, M.; Yalcinkaya, B.; Koštejn, M.; Jirsák, J.; Stibor, I.; Bara, J. E.; Van der Bruggen, B. Separation of Racemic Compound by Nanofibrous Composite Membranes with Chiral Selector. J. Membr. Sci. 2020, 596, 117728. DOI: 10.1016/j.memsci.2019.117728.
- Yoon, T.-H.; Hong, L.-Y.; Kim, D.-P. Chiral Separation by a Pseudo Membrane in a Triple-Laminar Flow with a Microfluidic Contactor. Chem. Asian J. 2011, 6(4), 1015–1018. DOI: 10.1002/asia.201000798.
- Wang, P.-C.; Gao, J.; Lee, C. S. High-resolution Chiral Separation Using Microfluidics-based Membrane Chromatography. J. Chromatogr. A. 2002, 942(1–2), 115–122. DOI: 10.1016/s0021-9673(01)01399-1.
- Fakhari, A. R.; Mohammadi Kosalar, H.; Asadi, S.; Hasheminasab, K. S. Surfactant‐assisted Electromembrane Extraction Combined with Cyclodextrin‐modified Capillary Electrophoresis for the Separation and Quantification of Tranylcypromine Enantiomers in Biological Samples. J. Sep. Sci. 2018, 41(2), 475–482. DOI: 10.1002/jssc.201700488.
- Nojavan, S.; Fakhari, A. R. Electro Membrane Extraction Combined with Capillary Electrophoresis for the Determination of Amlodipine Enantiomers in Biological Samples. J. Sep. Sci. 2010, 33(20), 3231–3238. DOI: 10.1002/jssc.201000242.
- El-Feky, H. H.; Cano-Odena, A.; Gumi, T. Facile Synthesis of Porous Monolithic Membrane Microdevice. J. Membr. Sci. 2013, 439, 96–102. DOI: 10.1016/j.memsci.2013.03.008.
- Grossehilmann, J.; Vanderveen, J. R.; Jessop, P. G.; Kragl, U. Switchable-Hydrophilicity Solvents for Product Isolation and Catalyst Recycling in Organocatalysis. Chemsuschem. 2016, 9(7), 696–702. DOI: 10.1002/cssc.201501654.
- Ohla, S.; Beyreiss, R.; Fritzsche, S.; Glaser, P.; Nagl, S.; Stockhausen, K.; Schneider, C.; Belder, D. Monitoring On-Chip Pictet-Spengler Reactions by Integrated Analytical Separation and Label-Free Time-Resolved Fluorescence. Chemistry. 2012, 18(4), 1240–1246. DOI: 10.1002/chem.201101768.
- Lotter, C.; Poehler, E.; Heiland, J. J.; Mauritz, L.; Belder, D. Enantioselective Reaction Monitoring Utilizing Two-dimensional Heart-cut Liquid Chromatography on an Integrated Microfluidic Chip. Lab Chip. 2016, 16(24), 4648–4652. DOI: 10.1039/c6lc01138a.
- Scatena, G. S.; de la Torre, A. F.; Cass, Q. B.; Rivera, D. G.; Paixao, M. W. Multicomponent Approach to Silica-Grafted Peptide Catalysts: A 3D Continuous-Flow Organocatalytic System with On-line Monitoring of Conversion and Stereoselectivity. Chemcatchem. 2014, 6(11), 3208–3214. DOI: 10.1002/cctc.201402501.
- Johnson, A. C.; Bowser, M. T. Micro Free Flow Electrophoresis. Lab Chip. 2018, 18(1), 27–40. DOI: 10.1039/c7lc01105a.
- Lu, N.; Sticker, D.; Kretschmann, A.; Petersen, N. J.; Kutter, J. P. A Thiol-ene Microfluidic Device Enabling Continuous Enzymatic Digestion and Electrophoretic Separation as Front-end to Mass Spectrometric Peptide Analysis. Anal. Bioanal. Chem. 2020, 412(15), 3559–3571. DOI: 10.1007/s00216-020-02609-5.