ABSTRACT
Objective
Individuals who sustain a traumatic spinal cord injury (SCI) often have a loss of multiple body systems. Significant functional improvement can be gained by individual SCI through the use of neuroprostheses based on electrical stimulation. The most common actions produced are grasp, overhead reach, trunk posture, standing, stepping, bladder/bowel/sexual function, and respiratory functions.
Methods
We review the fundamental principles of electrical stimulation, which are established, allowing stimulation to be safely delivered through implanted devices for many decades. We review four common clinical applications for SCI, including grasp/reach, standing/stepping, bladder/bowel function, and respiratory functions. Systems used to implement these functions have many common features, but are also customized based on the functional goals of each approach. Further, neuroprosthetic systems are customized based on the needs of each user.
Results & Conclusion:
The results to date show that implanted neuroprostheses can have a significant impact on the health, function, and quality of life for individuals with SCI. A key focus for the future is to make implanted neuroprostheses broadly available to the SCI population.
Introduction
Significant functional improvement can be gained by individuals with spinal cord injury (SCI) through the use of neuroprostheses based on electrical stimulation. This paper focuses on implanted neuroprostheses that use electrical pulses to generate action potentials in targeted peripheral nerves. In the case of SCI, the stimulation is applied to paralyzed motor nerves and produces contraction of the desired muscle. In most cases, multiple nerves and muscles are targeted and the stimulation is patterned and coordinated so that the resulting muscle contractions produce the desired functional action. The most common actions produced are grasp, overhead reach, trunk posture, standing, stepping, bladder/bowel/sexual function, and respiratory functions. We will begin this review by considering the perspectives and needs of spinal-cord-injured neuroprosthetic users.
Although this paper focuses on implantable neuroprostheses designed to provide functional independence, it should be noted that electrical stimulation can also be applied using electrodes applied to the surface and is often used as a means of exercise weak and partially paralyzed muscles. The most common application of this approach is for strengthening lower extremity muscles, and such exercise is often combined with bike riding and standing [Citation1]. Similar approaches have been applied for upper extremity strengthening [Citation2,Citation3]. Prolonged use of electrical stimulation for exercise may result in the recovery of function, although the mechanism is unknown [Citation4]. It may even be possible to strengthen denervated muscles if sufficient electrical current is applied [Citation5–7]. In general, systems utilizing surface stimulation are applied temporarily to achieve strengthening and recovery. For permanent function requiring electrical stimulation, surface stimulation has been used for walking [Citation8] but implanted systems are typically utilized due to the repeatability of the response and the minimal donning and doffing required.
Neuroprosthesis user perspectives in SCI
There is a growing recognition that the inclusion of persons living with a disease/disorder as part of the research process is critically important for many reasons. Among those reasons are the opportunity to learn about the everyday realities of living with that disease/disorder, the identification of research priorities, and the intention to target research efforts in a clinically relevant manner. When it comes to assistive technologies, the end-user perspective is critical to design choices, risk mitigation, ease of use, cosmetic appearance, and benefits to target. With respect to applications involving electrical stimulation and neuroprostheses for SCI, there is some literature revealing the preferences of people living with SCI.
What do we know about the views of people with SCI about electrical stimulation in general? There are perceived physical benefits (improvements in muscle activity and function) as a result of using electrical stimulation [Citation9]. These benefits are perceived to have a positive impact on activities of daily living and, thus, quality of life. Barriers that were identified include access to equipment and inconveniences with donning/doffing and not enough information and guidance about different types of neuroprostheses. An overarching recommendation from that study is the importance of getting user input early on in the design process.
A different group of investigators surveyed veterans living with SCI about their knowledge of and experience with various assistive technologies in general, including neuroprostheses using electrical stimulation, and design preferences for brain–computer interface (BCI) technology specifically [Citation10]. For the other assistive technologies, including computer access equipment, functional electrical stimulation (FES), robotic gait training, spinal cord stimulator, robotic arm assistant, tendon transfers, transcutaneous electrical nerve stimulation (TENS), and hand orthoses, the majority of respondents had either never heard of them or had heard of them but never used them. This demonstrates a disturbing lack of knowledge and access to clinically available technology. With regard to BCI technology, the majority of respondents were interested in using BCI to control neuroprostheses for bladder/bowel, hand grasp, and lower body function. Important design characteristics included independent operation, non-invasiveness, cost, number of functions, and daily set-up time.
With respect to implanted neuroprosthetic technology, a concerted effort has been made to understand user preferences. A group of developers of neuroprostheses held a Consumer Forum to help them better understand the priorities of people living with SCI and how technology may impact their lives [Citation11]. They included neuroprosthesis users and non-users in the forum. They found that research priorities depended on the individual, personal priorities, preferences, background, history, level of injury, etc., but there were some common themes that emerged. These included wanting technology that increased independence, ease of movement, ease of control, and spontaneity.
Brown-Triolo et al. [Citation12] surveyed 94 individuals with paraplegia to identify preferences related to mobility issues. Among various mobility tasks involving the lower extremities, walking and standing were identified as high priorities to people with paraplegia. With respect to neuroprosthetic devices to provide those functions, devices requiring invasive procedures were as acceptable as those not requiring non-invasive procedures. Furthermore, restoration of function by the neuroprosthetic devices did not need to reach pre-injury levels. What was of high concern, however, was the external visibility of devices.
The return of bladder function is a high priority to individuals with SCI regardless of injury level [Citation13]. Neuroprostheses can be used to restore bladder function in some individuals. A choice-based conjoint analysis was conducted [Citation14] to determine the importance of various features of three neuroprostheses targeting bladder function (sacral root stimulation with rhizotomy, rhizotomy-free sacral root stimulation, and pudendal nerve stimulation). Rhizotomy-free sacral root stimulation was preferred over pudendal nerve stimulation. Sacral root stimulation with rhizotomy was least preferred. Importantly, it was revealed that side effects had the greatest impact on preferences followed by the effect on continence and voiding, which were further followed by the degree of invasiveness, degree of user-friendliness, and cost.
User satisfaction is high for implanted neuroprosthetic devices to improve hand grasp [Citation15] and standing [Citation16]. Electrically stimulated hand grasp resulted in improvements in activities of daily living and independence, which had a positive impact on life [Citation15]. From the perspective of users of an implanted neuroprosthetic standing system, the system increased quality of life, improved health status, decreased spasticity, improved emotional well-being, increased the sense of self-worth, increased mobility, increased function in social settings, made them feel more ‘normal,’ decreased the need for caregivers, and enhanced the ability to work [Citation17].
Basic principles of electrical stimulation and neuroprostheses
Neuroprostheses using electrical stimulation have been well characterized over the past few decades. As we review here, the mechanism of action is known in detail. The parameters for safely delivering electrical stimulation have been established. The technology for delivering electrical stimulation to nerves has been refined over time and coupled with electronic control systems. The means of producing practical, deliverable systems using this approach have been developed. shows examples of the implanted neuroprosthetic components used to achieve the functions described in this review.
Figure 1. Implanted neuroprostheses components utilized for motor functions described in this review. (a] Eight-channel implanted pulse generator, used for grasp/reach and for trunk/standing applications. (b) Electrodes used to activate skeletal muscle. Electrode on the left is an epimysial style that is sutured to the muscle epimysium. Electrode on the right is an intramuscular electrode that is inserted inside the muscle via a probe. (c) Probe and sheath used for intraoperative testing and insertion of intramuscular electrodes. (d) Three-channel implantable stimulator used for sacral anterior root stimulation for bladder function and also used for epidural stimulation to generate cough. (e) Extradural electrodes that are sutured next to anterior roots for bladder function.
![Figure 1. Implanted neuroprostheses components utilized for motor functions described in this review. (a] Eight-channel implanted pulse generator, used for grasp/reach and for trunk/standing applications. (b) Electrodes used to activate skeletal muscle. Electrode on the left is an epimysial style that is sutured to the muscle epimysium. Electrode on the right is an intramuscular electrode that is inserted inside the muscle via a probe. (c) Probe and sheath used for intraoperative testing and insertion of intramuscular electrodes. (d) Three-channel implantable stimulator used for sacral anterior root stimulation for bladder function and also used for epidural stimulation to generate cough. (e) Extradural electrodes that are sutured next to anterior roots for bladder function.](/cms/asset/971b8e3a-9766-41e5-ba85-6ffbd8f74844/yner_a_1798106_f0001_oc.jpg)
Mechanism of action
An electrical pulse produces an action potential in nerves through the depolarization of the voltage-gated ion channels in the nerve membrane. If the stimulus strength exceeds the membrane threshold, the neuron will depolarize, generating an action potential. Action potentials generated along an axon in this manner will travel both orthodromically and antidromically, but otherwise are identical to a naturally-occurring action potential, and thus it is indistinguishable at the end organ. When the stimulus pulse is above the threshold, every pulse will generate an action potential. The rate of activation is directly correlated with the stimulation rate.
Safe stimulation
Electrical stimulation can be safely delivered to nerves through the proper selection of stimulus parameters and electrode design. The critical electrical stimulation parameters include charge per phase, charge density per phase, and maintaining a zero net charge. The electrode geometry and electrode materials also contribute to long-term biocompatibility and safety.
Biphasic stimulation with zero net charge should be used as this allows the pulse to pulse reversal of the electrochemical reactions at the electrode–tissue interface [Citation18,Citation19]. In general, the first phase is cathodic (at the activating electrode) and is typically a rectangular pulse defined by the pulse duration (PD) and pulse amplitude. Charge per phase and charge density per phase of the cathodic activating pulse have been identified as primary factors in determining safe stimulus levels [Citation19–22]. In general, charge densities in the range of 10–100 µC/cm2 per phase are in the safe region for brain tissue, and even higher charge densities can be tolerated in the peripheral nervous system. The maximum stimulation output for a number of commercial and research-based neurostimulation and neuromodulation devices is shown in . The maximum charge per phase and charge density per phase are also shown for these devices.
Table 1. Safe levels of electrical stimulation.
The tissue response is independent of stimulus frequency when the frequency is below 50 Hz. Most motor neuroprosthetic applications use frequencies of 20 Hz or lower, so frequency is not a factor. For stimulation rates above 50 Hz, there is some evidence of a ‘mass action’ effect, in which damage occurs in the nerves due to the increased overall nerve activity [Citation24–26]. This has the effect of reducing the expected safe levels of charge density per phase when higher frequencies are used.
A variety of safe and durable electrode materials have been identified and utilized in pre-clinical and clinically deployed electrical stimulation devices. Common electrode materials are 316LVM stainless steel, platinum, and platinum-iridium (90%-10% most commonly) [Citation27,Citation28]. Titanium is commonly used as the return current electrode, particularly when the pulse generator case serves as the return. Other materials have been evaluated due to their potential for increased charge density, such as tantalum and activated iridium, but these are of primary interest for use in intra-cortical activation, where the tissue is more sensitive and where very small surface areas are required.
Electrode geometry is primarily important for the direct mechanical effect of the electrode on the tissue rather than the electrochemical impact. Electrodes should not have sharp surfaces and should be anchored in the tissue to avoid abrasion effects during movement. Lead routing should be oriented so that the lead tethering does not place significant torques on the electrode. Leads should also be routed so that they do not lay next to a nerve, which may result in compression damage. The sizing of nerve cuff electrodes has been studied extensively, and it is important that the cuff is sized appropriately for the target nerve [Citation29].
It is important to note that the anterior horn cell, axon, neuromuscular junction, and muscle fiber must be intact for electrical stimulation to be effective [Citation30]. Direct activation of muscle faces challenges due to the large current and charge required for depolarization, which is approximately two orders of magnitude higher than the threshold for nerve stimulation [Citation19]. This is true even for electrodes inserted into muscle tissue.
The stimulus threshold of any neuron is inversely proportional to the diameter of the neuron. Large-diameter neurons, which have the lowest threshold for stimulation, are activated first, followed by smaller neurons down to the smallest C-fiber [Citation31]. This property of electrical stimulation is called the reverse recruitment order, as normal volitional motor activation adheres to Henneman’s size principle [Citation32] which entails progressive activation of smaller motor units recruited before large motor units. It is commonly reported that this reverse recruitment results in rapid fatigue, severely limiting the usefulness of neuroprostheses. In practice, however, this has not proven to be a major issue except for high force activities such as standing and walking.
Electrical stimulation can produce graded muscle force output either through spatial or temporal summation. Spatial summation is by far the most commonly utilized in neuroprostheses. As the intensity of the stimulus pulse (either current amplitude or pulse duration or both) is increased, the current spreads and activates a progressively larger region of neurons, resulting in increased muscle contraction strength. Current can also spread to nearby nerve fibers innervating additional muscles, a characteristic referred to as spillover. In most functional applications, it is desirable to isolate the recruitment to single muscles or muscle groups.Temporal summation occurs when the rate of stimulation is increased, resulting in a more rapid contraction of muscle fibers. In practice, however, low frequencies (less than 10 Hz) produce individual twitches that are generally not useful for producing the tonic forces necessary for functional tasks. Higher frequencies (greater than 25 Hz) result in rapid fatigue. Given these constraints, the frequency range of neuroprosthetic systems is typically 12 Hz – 20 Hz, leaving little room for temporal summation.
One aspect that separates neuroprostheses from typical neuromodulation devices or even pacemakers is that users are given direct control over when and how the stimulation is delivered. This is because the stimulation produces a motor activity that must be incorporated into the individual’s daily routine. shows some of the different control methods used for implanted neuroprostheses. For some systems, such as those for cough or bladder function, the user activates a switch that triggers the function. A preprogrammed stimulation pattern is then delivered to the target nerves/muscles to produce the desired effect. For other systems, particularly for upper and lower extremity function, the control systems are much more incorporated into the patterning of the stimulation. For these systems, real-time adjustments to the stimulation are necessary – such as step-to-step adjustments or smooth control of grasp opening and closing. A key goal in these systems is to develop a control method and algorithm that is as natural and unobtrusive as possible. ‘Natural’ in this context means that as the user volitionally attempts to make the desired movement and the control system properly interprets the intention and then delivers the appropriate stimulation pattern to accomplish the desired movement. In practice, however, it is rarely possible to interpret an individual’s intended movement with a high degree of accuracy. Therefore, control systems usually require a period of training in order to gain proficiency in the use of the system.
Figure 2. Methods of user control of neuroprosthetic systems. (a) Control of grasp and release can be achieved using myoelectric control. Shown is the placement of a bipolar myoelectric signal recording electrode placed on the wrist extensor. Inset shows electrode skirt sutured to muscle epimysium. Leads are routed subcutaneously to implanted controller. (b) Finger-mounted switch used to control standing and stepping functions for implanted neuroprostheses. Switches are mounted on a ring and used to initiate and terminate desired stimulation patterns. (c) Diaphragm pacing control system, worn by the user but generally controlled by caregiver. Stimulation intensity and breathing rate can be adjusted as necessary. (d) External control device for implanted bladder/bowel control system. The user depressed one of three pre-programmed patterns, depending on the desired function.
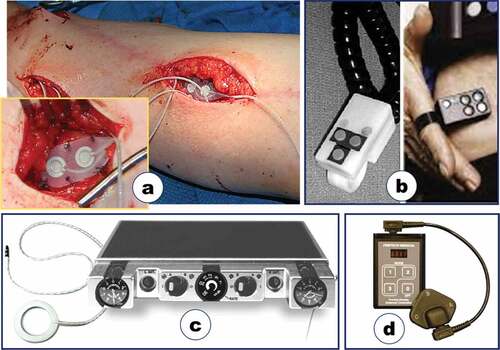
Implanted grasp and reach neuroprostheses
Hand and arm control is the most highly desired function for people with spinal cord injury at the cervical level [Citation13]. Electrical stimulation of the paralyzed muscles of the hand and arm can be used to provide grasp and reach for individuals with cervical SCI. A key aspect of these upper extremity neuroprostheses is that the user requires real-time control over the movements of the arm and hand so that these movements can be incorporated into intentional functional tasks [Citation33]. This control is derived from the user’s remaining voluntary function and can include joint movement, myoelectric signals, and direct brain control through a brain–computer interface (BCI).
Upper extremity neuroprostheses are often combined with other surgical interventions such as tendon transfers and nerve transfers [Citation34,Citation35]. Tendon transfers involve disconnecting the tendon insertion of a muscle under voluntary control and connecting it to the tendon of a paralyzed muscle so that contraction of the donor muscle results in movement that was previously paralyzed. Tendon transfers have been used quite extensively for the restoration of pinch and elbow extension in cervical SCI [Citation36]. The criteria are that the patients have a transferrable muscle available as the donor. Common transfers can provide elbow extension, wrist extension, thumb flexion, and finger flexion. These functions can be combined with the electrically stimulated function obtained from a neuroprosthesis.
Recently, the use of nerve transfers has begun to be studied in SCI [Citation37]. In this case, a donor nerve is selected and crossed to a nerve that innervates a paralyzed muscle that one wishes to have under voluntary control. The transferred nerve grows down the paralyzed nerve tract and reinnervates the target muscles. Recovery of function typically requires 6–12 months. Nerve transfers usually need to be performed within six months of injury in order to utilize a viable nerve tract for regrowth.
The first commercially available implanted neuroprosthesis to provide significant gains in hand and arm function for individuals with tetraplegia was the Freehand System. The Freehand ® neuroprosthesis utilized an eight-channel receiver-stimulator (IRS-8), eight epimysial or intramuscular electrodes, leads, and connectors [Citation38]. Electrodes were surgically placed on or in the paralyzed muscles of the forearm and hand, and an inductive link provided the communication and power to the implanted pulse generator. shows the steps for the implantation of intramuscular electrodes. The external components of the neuroprosthesis were an external control unit, a transmitting coil, and an external shoulder position transducer. The external control unit performed the signal processing of the control inputs and generated the output signal delivered to the implant. Two grasp patterns were provided for functional activities: lateral pinch and palmar prehension [Citation39]. Control of grasp opening and closing was achieved through graded elevation of the user’s contralateral shoulder [Citation40]. The Freehand system was transferred to industry (NeuroControl Corp.) and was implemented successfully in over 220 patients [Citation41,Citation42]. Premarket approval (PMA) from the FDA was received in 1997, and over 40 sites internationally were trained in its deployment.
Figure 3. Implantation of an intramuscular electrode in the dorsal intrinsic muscles of the hand. (a) After probe with sheath has been used to verify stimulated response, the probe is removed and the electrode inserted into the sheath until it protrudes from the sheath and anchors in the muscle. (b) Close-up showing electrode about to be inserted into sheath. The electrode is delivered on a shuttle that can be used to push the electrode down the sheath. (c) Electrode and shuttle are fully inserted into the sheath. (d) Shuttle is removed, leaving electrode in the muscle. (e) Sheath is removed. (f) Electrode lead is tunneled subcutaneously to the implanted stimulator using a polymer tunneler. Suction is connected to one end of the tunneler and saline is squirted into the other end to draw the electrode lead through the tunneler.
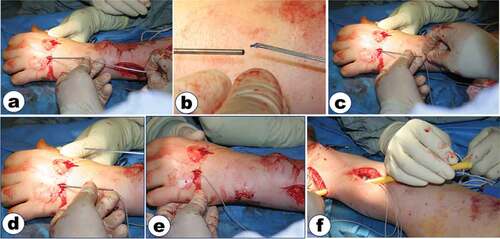
The functional impact of the Freehand System was high. The neuroprosthesis produced increased pinch force in every recipient, and there was a significant increase in the ability to manipulate objects of different size and weight [Citation42,Citation43]. The independence provided by the neuroprosthesis was directly compared to the maximum independence that could be provided by any other means, e.g. orthotics or tendon transfers. With the neuroprosthesis, 100% of the participants (n = 28) had greater independence in at least one task, and 78% were more independent using the neuroprosthesis in at least three tasks. All participants preferred to use the neuroprosthesis for at least one task and 96% preferred to use the neuroprosthesis for at least three tasks [Citation42]. More than 90% of the participants were satisfied with the neuroprosthesis [Citation15].
The team that originally developed the Freehand System subsequently developed a second-generation system referred to as the implanted stimulator-telemeter (IST-12) [Citation44]. The functional specifications for the second generation neuroprosthesis were developed based on interviews with neuroprosthesis users and clinicians. The IST-12 System was a myoelectrically controlled neuroprosthesis providing control of grasp, forearm pronation, and elbow extension for individuals who are paralyzed with cervical level spinal cord injury. A key feature of this system was the implantation of both the control and the stimulation source, which reduced the external components by 50% compared to the Freehand System. The IST-12 consisted of 12 stimulation channels and two channels of myoelectric signal recording acquisition.
Twelve subjects were implanted with the IST-12 device, including three with systems for restoring movement in both hands [Citation35,Citation45]. Subjects were able to successfully use the myoelectric signal from their wrist extensor for proportional control of grasp opening and closing. Subjects also demonstrated the ability to generate myoelectric signals from trapezius, platysma, deltoid, and biceps muscles. The use of myoelectric control in neuroprostheses allowed considerable flexibility in the control algorithms, enabling them to be tailored to each individual subject. The study results indicated that every subject improved significantly in pinch force strength and in the ability to manipulate objects [Citation35]. The ability to perform activities of daily living was assessed for 13 arms in 10 subjects. Every subject demonstrated improvement in at least two activities, with one subject demonstrating improvement in 11 of 12 activities tested and another subject demonstrating improvement in 9 of 9 activities tested. All 13 arms in the 10 subjects showed improved function in eating with a fork and 12/13 showed improvement in writing with a pen. Other tasks in which subjects showed improvement included office tasks, using a cell phone, getting money out of a wallet, and embroidery.
The use of implanted neuroprostheses for individuals with high tetraplegia (C4 or higher) has been rare [Citation46], although implanted intracortical arrays have been used with the surface [Citation47] or percutaneous [Citation48] stimulation systems. Two high tetraplegic individuals were implanted with dual IST-12 systems for shoulder, arm, and hand function using myoelectric control from the shoulder or neck [Citation46]. Each individual was able to perform at least two activities of daily living using the neuroprostheses, whereas they were completely dependent without the neuroprosthesis. Difficulties included significant denervation in the deltoid and biceps muscles and developing control algorithms that were easy to learn and use.
Implanted neuroprostheses for exercise, standing and stepping
Both cervical and thoracic SCI result in paralysis of the torso and pelvis that can lead to unhealthy and non-functional seated postures which may be one of the primary contributing factors to chronic health problems in wheelchair users [Citation49]. Following SCI, lack of voluntary or reflexive control of the paralyzed trunk and hip musculature compromises seated posture and balance, significantly limiting the independent performance of activities of daily living (ADLs) and contributing to secondary health complications such as pressure ulcers, pathological posterior pelvic tilt, and pathological vertebral alignment, and even leading to secondary health complications such as reduced respiratory capacity. Stiffening the paralyzed trunk and hip extensors with continuous electrical stimulation has a multitude of benefits: it can correct kyphotic seated postures, normalize lateral vertebral alignment, improve ventilation and respiratory volumes, and alter interface pressures. It can also expand bimanual workspace, statically stabilize the torso, increase the forces that can be exerted on objects with the upper extremities, return users to erect sitting from a fully forward-flexed posture, and improve manual wheelchair propulsion efficiency at comfortable speeds [Citation50,Citation51].
The inability to stand or step is another limitation that significantly limits the performance of individuals with many activities of daily living (ADL) such as washing dishes at a counter, reaching items on high shelves, or essential transfers between surfaces of varying heights. For individuals with thoracic-level SCI, stimulated contractions of the lower extremity muscles can enable standing and stepping, facilitate independent transfers, increase personal mobility, and improve general health and quality of life after complete paralysis, as well as significantly enhance walking performance after incomplete injuries [Citation52,Citation53].
Since the long paraspinal muscles (longissimus, iliocostalis, and spinalis) are segmentally innervated, they can only be partially activated with single-channel stimulation. Therefore, stimulation of the L1-L2 or T12-L1 spinal roots with intramuscular electrodes results in the activation of multiple lumbar segments of the erector spinae muscles to provide trunk extension. Carefully placed intramuscular electrodes at the T11-L2 spinal roots can also activate the iliopsoas, quadratus lumborum, rectus abdominus, obliques, and other core trunk muscles [Citation54,Citation55]. Bilateral activation of the lumbar erector spinae with intramuscular electrodes inserted at these locations can reduce the posterior pelvic tilt, restore a more normal lumbar curve, and shift the workspace of seated neuroprosthesis users forward and upward. shows the implantation procedure for electrodes inserted in the lumbar paraspinal muscles. Stabilizing the lumbar spine with stimulation of the paraspinal muscles can allow heavier objects to be manipulated greater distances from the body, and improve stable seated posture [Citation56–58].
Figure 4. Surgical placement of electrodes in the erector spinae muscles under fluoroscopic guidance. (a) Probes are inserted directly into the target muscles. Probes are insulated stainless steel with exposed tips, allowing for stimulation to be applied to the tip. (b) C-arm fluoroscopy is used to visualize the tip of the probes in relation to the vertebrae. (c) Electrical stimulation is applied to the probe to observe muscle response. (d) Once the desired location has been achieved, the electrode is inserted via a sheath into the target muscle.
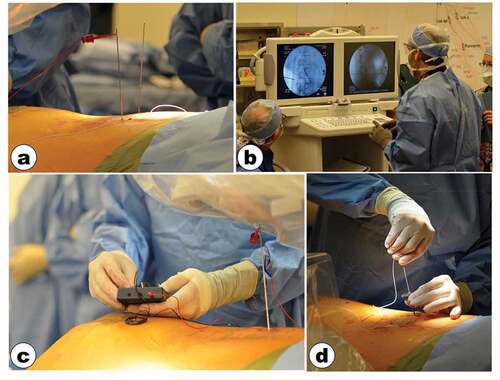
Recipients of an implanted neuroprosthesis with epimysial and intramuscular electrodes that continuously activated the vasti, gluteals, hamstrings, and lumbar erector spinae exhibited mean standing times of 10 minutes, and maintained the usage patterns and functional abilities for up to 17 years post-rehabilitation with their systems [Citation52,Citation53]. This is sufficient for facilitating transfers to high surfaces, performing swing-to gait for short distances in wheelchair inaccessible environments, and participating in other social, work, and personal activities. Some implant recipients in a Phase II clinical trial of the system were able to stand for more than 20 minutes, and all were able to release one hand from a walker or assistive device to reach objects overhead. Long-term use of neuroprostheses for standing was safe and effective and had no adverse physiological effects. Stepping of up to 100 m has also been achieved after paralysis with simple pre-programmed patterns of open-loop stimulation delivered from the surface electrodes or via 8- and 16-channel implanted pulse generators [Citation52].
Implanted neuroprostheses for respiratory function
Spinal cord injury at C4 or higher can result in full or partial paralysis of the diaphragm, making the individual dependent on mechanical ventilation for breathing function. Side effects of mechanical ventilation include respiratory tract infections, difficulty with speech, as well as the practical issues of physical discomfort, and limited mobility. Diaphragm pacing is a significant improvement over mechanical ventilation systems. Electrically stimulated pacing reduces or eliminates these effects, providing a more natural form of breathing.
Neuroprostheses for respiration can be produced via stimulation of the phrenic nerve bilaterally. A number of implanted diaphragm pacing systems were commercialized [Avery, Medimplant, Atrotech]. These systems use an electrode placed around the phrenic nerve, connected to an implanted stimulator that drives breathing at a constant rate [Citation59–61]. The proper function requires that the phrenic nerve is intact without significant lower motor neuron damage, and this is a key screening criterion. Phrenic nerve electrodes are typically placed through a thoracic approach. Longitudinal studies demonstrate that these electrodes, when properly placed, do not damage the phrenic nerve [Citation62,Citation63].
The stimulated respiratory rate is typically 8–14 breaths/minute [Citation64]. Tidal volume can be adjusted by altering the stimulation frequency, but should not exceed 20 Hz. It is important to note that diaphragm pacing requires a period of training before the individual achieves full-time ventilatory support using electrical pacing. Specifically, it is necessary to build diaphragm strength and fatigue resistance in order to achieve adequate ventilation using electrical stimulation. In general, users first achieve stimulated ventilation during the day and continue to use mechanical ventilation at night. With weeks of regular use, many users can wean entirely off of mechanical ventilation.
The outcomes show that diaphragm pacing has significant advantages over mechanical ventilation. [Citation64] reported effective stimulation was achieved in 82%–90% of cases. Phrenic pacing has successfully been used for as long as 21 years [Citation60]. Respiratory tract infections may be significantly reduced, and complication is low.
It is also possible to activate the diaphragm via electrodes placed in the diaphragm itself [Citation65,Citation66]. Intramuscular electrodes are used in this case, with leads extending percutaneously to an external stimulator. This approach has the advantage of being minimally invasive, particularly relative to the surgical risks of placement of electrodes on the phrenic nerve.
Respiratory muscles are partially paralyzed in many cervical level injuries because the respiratory muscles of the chest are innervated by spinal roots in the thoracic cord. Even when the diaphragm is spared, breathing is compromised due to the lack of major inspiratory and expiratory intercostal muscles. Abdominal muscles also play a role in expiration. Therefore, in particular, cough is weak and thus it can be difficult for individuals to clear their airway.
A clinical trial using lower thoracic spinal cord stimulation (SCS) to restore an effective cough system was conducted in 12 tetraplegic subjects [Citation67,Citation68]. A fully implantable electrical stimulation system was surgically placed in each subject. Partial hemi-laminotomies were used to place single-disc electrodes in the dorsal epidural space at the T9, T11, and L1 spinal levels. A radiofrequency receiver (Finetech Medical) was also positioned in a subcutaneous pocket over the anterior chest. Electrode wires were tunneled subcutaneously and connected to the radiofrequency receiver. Stimulation was applied via an external antenna by activating a small portable external stimulus controller box powered by a rechargeable battery to each electrode lead alone and in combination. Nine patients were followed for a minimum of 12 months and demonstrated that supramaximal SCS generates large positive airway pressures and high peak airflow rates during stimulation at each electrode lead. Maximum airway pressures and peak airflow rates were achieved with combined stimulation of any two leads. At total lung capacity, mean maximum airway pressure generation, and peak airflow rates were 130 ± 30 cmH2O and 8.6 ± 1.8 L/s, respectively. Importantly, repetitive stimulation every 30 sec for 30 min did not result in the development of fatigue as pressure generation remained unchanged [Citation68]. SCS was performed at home 2–3 times/day on a chronic basis, and also as needed for secretion management. The degree of difficulty in raising secretions improved markedly and the need for alternative methods of secretion removal were virtually eliminated. Subject life quality related to respiratory care also improved with subjects reporting greater control of their breathing problems. The incidence of pneumonia fell significantly from 1.7 ± 0.5 to 0.2 ± 0.1 events/subject year (p < 0.01) and mean level of trained caregiver support related to secretion management decreased from 16.9 ± 7.9 to 2.9 ± 1.2 times/week. Subject mobility also increased significantly as many individuals no longer had to travel with trained caregivers and/or with any apparatus for suctioning. Importantly, there were no instances of bowel or bladder leakage. These preliminary results strongly suggest that the SCS cough system facilitates secretion management, reduces the need for caregiver support and reduces the incidence of respiratory tract infection. Application of this system may also reduce overall health-care costs as reduce the morbidity and mortality associated with respiratory complications in subjects with SCI. The SCS cough system therefore may become an important clinical tool in the respiratory management of subjects with spinal cord injury. A further study evaluated a less invasive techniques for electrode installation using a wire (or ‘linear’) electrode [Citation69]. This electrode can be inserted through the vertebral foramen or inter-vertebral space eliminating the need for multiple laminotomies.
Implanted neuroprostheses for bladder and bowel function
The goal of electrical stimulation of the urinary system is to restore on-command micturition and then continence at all other times. Of all body functions desired by the combination populations of tetraplegia and paraplegia, this is the highest priority need in SCI [Citation13]. It is important not only for the personal and social implications, but poor bladder management has medical implications. These include inducing autonomic dysreflexia, and bladder infections, which create significant health risks. The alternative means for bladder management provide for the external collection of urine with indwelling catheters of various forms. Necessarily, these are not very desirable and create health risks of their own.
Sacral root stimulation can be used to activate bladder, bowel, and sexual function [Citation70]. A U.S. study of the sacral anterior root stimulator (SARS) showed that the majority of patients utilized the system routinely for producing micturition 4–6 times per day. In a study of 20 subjects who received this system, the average post-void residual volume with the stimulator was 22 ± 14 ml and the median number of urine infections decreased from 7 to 1 per year [Citation71], which substantially reduces the use of antibiotics. Eighteen of these 20 subjects became catheter-free. The abolition of detrusor hyperreflexia by posterior rhizotomy allowed patients to discontinue anticholinergic medications, which in turn reduced constipation and other side effects such as a dry mouth, blurred vision, and drowsiness. Continence was achieved in over 85% of patients, but this is largely attributable to the abolition of detrusor hyperreflexia and increase in bladder compliance which follow posterior sacral rhizotomy. Interviews by a life care plan analyst with patients between six months and six years after implantation and rhizotomy indicated a six-fold reduction in the costs of bladder and bowel care, predicting that savings in bladder and bowel care would equal the cost of purchasing and implanting the stimulator after about five years, and thereafter be expected to result in progressive savings to the health-care payer [Citation72].
The SARS system can also have a positive impact on bowel management. Most users report a reduction in constipation and reduced need for laxatives and stool softeners. Some users are able to defecate by a pattern of intermittent stimulation similar to that used for micturition but with longer intervals between bursts of stimulation to allow passage of stool. In the Cleveland series, the time spent on bowel emptying was reduced by 75% [Citation71].
Bladder hyperreflexia is a major problem that must be addressed in an effective neural prosthesis for bladder control. Stimulation of peripheral sensory pathways can access and influence the spinal neural circuits that control pelvic reflexes and function. Afferent-mediated neural prostheses take advantage of natural nervous system processes and are potentially less invasive than spinal-root-based approaches such as the SARS system. This approach has the potential to provide more natural function than motor-driven approaches, though it is more dependent on stimulation patterns and other inputs to the spinal circuits. One such approach uses genital nerve stimulation to achieve direct spinal level bladder inhibition [Citation73,Citation74]. This approach has primarily been used acutely, but it has also shown to improve urinary continence and bladder capacity in persons with SCI during short-duration use. If longer-term use is effective, then this approach may provide both a noninvasive and implanted option. Bladder inhibition via implanted electrodes on the pudendal nerve and sacral roots can also provide bladder inhibition in individuals with SCI [Citation75,Citation76].
Summary
The success of neuroprostheses in providing increased independence, when compared to all existing alternative strategies for this population, is nearly universal [Citation77,Citation78]. Arguably, the most important indication of the success of these systems is user acceptance and level of satisfaction. The following paragraphs include spontaneous comments from various individuals who have sustained a spinal cord injury who are using implantable neuroprosthetic systems.
From a person with a C5 complete SCI who is an upper extremity neuroprosthesis user: ‘I was very physical all of my life and worked as an assistant dog trainer, but after my SCI, I could not wash my face, comb my hair, write, or even drink a glass of juice. After I was implanted with the upper extremity neuroprosthesis I could write, comb my hair, apply lipstick, wash my face, drink a glass of juice, and I have returned to doing embroidery.’
From a person with C6 complete SCI who has a trunk and hand neuroprosthesis: ‘To go for 21-plus years as a quadriplegic – no feeling, no ability to move – it’s scary sometimes. I turn my trunk system on, I feel rigid, I feel muscles below my injury line that I haven’t felt for a long time, and it feels good.’
From a person with C2 tetraplegia who uses a cough neuroprosthesis: ‘I was a college student living independently of my family before my SCI. The injury was devastating, and I lost my freedom. I could not go anywhere without my nurse or mom, as when I had difficulty breathing I needed them to suction me. Following implantation of the cough system, I was able to go out with my friends without my mom or nurse and enjoy myself. … This system has opened up my life to my friends without being a burden to my mom or nursing staff.’
From a person who is a T9 paraplegic who uses a bladder stimulation neuroprosthesis: ‘I had a [urinary tract infection] UTI every month for the first 10 months after SCI. Worse than that, I was afraid to go out because I was afraid I would have an accident. Once I got the bladder stimulation system, I could void when I wanted, I could leave my house without fear of an accident, and I have had only 4 infections in the last 6 years.’
From a person with a C6 complete SCI who is an upper extremity neuroprosthesis user: ‘I’m a daily user and rely on it to enable me to work full-time with no support hours required during work hours.’
The cost-benefit of neuroprostheses has been studied for bladder and hand neuroprostheses. In the case of bladder neuroprostheses, the reduction in secondary health conditions, particularly urinary tract infections, resulted in an overall reduction in the cost of care which exceeded the cost of the implant and maintenance within five years [Citation72]. In the case of hand neuroprostheses, it was demonstrated that a reduction in attendant care time could offset the cost of the surgical procedure and system maintenance over the lifetime of the recipient [Citation79]. Further analysis of the costs-benefits of implanted neuroprostheses needs to consider the impact of improved quality of life.
In summary, it is possible to utilize advanced implantable neuroprostheses to restore function for individuals with SCI. These systems have demonstrated use for more than two decades, indicating a high degree of utility, functional benefits, and, in some cases, significant health benefits. The surgical installation methods are well established, and the technology has proven to be robust and safe. A key focus for the future is to make implanted neuroprostheses broadly available to the SCI population within existing and evolving health-care delivery systems.
Disclosure statement
No potential conflict of interest was reported by the authors.
Additional information
Funding
Notes on contributors
Kevin L. Kilgore
Kevin L. Kilgore received a BS in Biomedical Engineering from the University of Iowa and an MS and PhD in Biomedical Engineering from Case Western Reserve University. He is Professor of Orthopaedics and Professor of Physical Medicine and Rehabilitation (Joint) at MetroHealth Medical Center and Biomedical Engineer in the Research Service at the VA Northeast Ohio Healthcare System. His research interests include the design, development, and implementation of implanted neuroprostheses for individuals with spinal cord injury and the development of new techniques for electrical block of nerve conduction.
Kimberly D. Anderson
Kimberly D. Anderson is a Professor in the Department of Physical Medicine and Rehabilitation at the MetroHealth System and Case Western Reserve University (CWRU) School of Medicine, and Associate Director of the Institute for Functional Restoration. She also has lived with a cervical spinal cord injury for 31 years. Her research focuses on translational investigations and bridging the gap between basic science, clinical science, and the public community living with spinal cord injury (SCI). At MetroHealth-CWRU she is continuing her involvement in clinical trials with the team pursuing implanted stimulation devices for SCI and further developing her independent research efforts addressing issues important to people living with SCI with an emphasis on translational research to deploy treatments to the clinic.
P. Hunter Peckham
P. Hunter Peckham completed a BS in mechanical engineering at the Clarkson College of Technology and a MS and PhD in Biomedical Engineering at Case Western Reserve University (CWRU). He is Donnell Professor of Biomedical Engineering and Orthopaedics at CWRU and a member of the National Academy of Engineering. His research interests include functional electrical stimulation and neural prostheses for the restoration of function following spinal cord injury.
References
- Tefertiller C, Gerber D. Step ergometer training augmented with functional electrical stimulation in individuals with chronic spinal cord injury: a feasibility study. Artif Organs. 2017 Nov;41(11):E196–E202.
- Alon G, McBride K. Persons with C5 or C6 tetraplegia achieve selected functional gains using a neuroprosthesis. Arch Phys Med Rehabil. 2003;84(1):119–124.
- Popovic MR, Thrasher TA, Adams ME, et al. Functional electrical therapy: retraining grasping in spinal cord injury. Spinal Cord. 2006;44(3):143–151.
- Inanici F, Samejima S, Gad P, et al. Transcutaneous electrical spinal stimulation promotes long-term recovery of upper extremity functions in chronic tetraplegia: a case study. IEEE Trans Neural Syst Rehabil Eng (TNSRE). 2018;26(6):1272–1278.
- Albertin G, Hofer C, Zampieri S, et al. In complete SCI patients, long-term functional electrical stimulation of permanent denervated muscles increases epidermis thickness. Neurol Res. 2018;40:277–282.
- Kern H, Carraro U, Adami N, et al. One year of home-based daily FES in complete lower motor neuron paraplegia: recovery of tetanic contractility drives the structural improvements of denervated muscle. Neurol Res. 2010;32:26–31.
- Lømo T, Westgaard RH, Hennig R, et al. The response of denervated muscle to long-term electrical stimulation. Eur J Transl Myol. 2014;24(1):3300. eCollection 2014 Mar 31.
- Gallien P, Brissot R, Eyssette M, et al. Restoration of gait by functional electrical stimulation for spinal cord injured patients. Paraplegia. 1995 Nov;33(11):660–664.
- Donovan-Hall MK, Burridge J, Dibb B, et al. The views of people with spinal cord injury about the use of functional electrical stimulation. Artif Organs. 2011;35(3):204–211.
- Collinger JL, Boninger ML, Bruns TM, et al. Functional priorities, assistive technology, and brain-computer interfaces after spinal cord injury. J Rehabil Res Dev. 2013;50(2):145–160.
- Kilgore KL, Scherer M, Bobblitt R, et al. Neuroprosthesis consumers’ forum: consumer priorities for research directions. J Rehabil Res Dev. 2001;38(6):655–660.
- Brown-Triolo DL, Roach MJ, Nelson K, et al. Consumer perspectives on mobility: implications for neuroprosthesis design. J Rehabil Res Dev. 2002;39(6):659–669.
- Anderson KD. Targeting recovery: priorities of the spinal cord-injured population. J Neurotrauma. 2004;21(10):1371–1383.
- Sanders PM, Ijzerman MJ, Roach MJ, et al. Patient preferences for next generation neural prostheses to restore bladder function. Spinal Cord. 2011;49(1):113–119.
- Stroh Wuolle K, Van Doren CL, Bryden AM, et al. Satisfaction with and usage of a hand neuroprosthesis. Arch Phys Med Rehabil. 1999;80:206–213.
- Agarwal S, Triolo RJ, Kobetic R, et al. Long-term user perceptions of an implanted neuroprosthesis for exercise, standing, and transfers after spinal cord injury. J Rehabil Res Dev. 2003;40(3):241–252.
- Rohde LM, Bonder BR, Triolo RJ. Exploratory study of perceived quality of life with implanted standing neuroprostheses. J Rehabil Res Dev. 2012;49(2):265–278.
- Merrill DR, Bikson M, Jefferys JG. Electrical stimulation of excitable tissue: design of efficacious and safe protocols. J Neurosci Methods. 2005;141(2):171–198.
- Mortimer JT. Motor prostheses. In: Brookhart JM, Mountcastle VB, editors. Handbook of physiology-the nervous system II. Bethesda, MD: American Physiolgical Society; 1981. p. 155–187.
- Agnew WF, McCreery DB, Yuen TG, et al. Histologic and physiologic evaluation of electrically stimulated peripheral nerve: considerations for the selection of parameters. Ann Biomed Eng. 1990a;17(1):39–60.
- McCreery DB, Agnew WF, Yuen TG, et al. Charge density and charge per phase as cofactors in neural injury induced by electrical stimulation. IEEE Trans Biomed Eng. 1990;37(10):996–1001.
- Pudenz RH, Agnew WF, Yuen TG, et al. Adverse effects of electrical energy applied to the nervous system. Appl Neurophysiol. 1977;40(2–4):72–87.
- Yuen Ted G. H., Agnew WF, Bullara LA. Histopathological evaluation of dog sacral nerve after chronic electrical stimulation for micturition. Neurosurgery. 1984 Apr;14(4):449–455.
- Agnew WF, McCreery DB, Bullara LA, et al. Effects of prolonged electrical stimulation of peripheral nerve. In: Agnew WF, McCreery DB, editors. Neural prostheses: fundamental studies. Englewood Cliffs, NJ: Prentice Hall; 1990b. p. 226–252.
- McCreery DB, Agnew WF, Yuen TG, et al. Damage in peripheral nerve from continuous electrical stimulation: comparison of two stimulus waveforms. Med Biol Eng Comput. 1992;30:109–114.
- McCreery DB, Agnew WF, Yuen TGH, et al. Relationship between stimulus amplitude, stimulus frequency and neural damage during electrical stimulation of sciatic nerve of cat. Med Biol Eng Comput. 1995;33:426–429.
- Naples GG, Mortimer JT, Yen TGH. Overview of peripheral nerve electrode design and implantation. In: Agnew WF, McCreery DB, editors. Neural prosthetics: fundamental studies. Englewood Cliffs, NJ: Prentice Hall; 1990. p. 107–145.
- Smith B, Buckett JR, Peckham PH, et al. An externally powered, multichannel, implantable stimulator for versatile control of paralyzed muscle. IEEE Trans Biomed Eng. 1987;34:499–508.
- Naples GG, Mortimer JT, Scheiner A, et al. A spiral nerve cuff electrode for peripheral nerve stimulation. IEEE Trans Biomed Eng. 1988;35:905–916.
- Bryden AM, Nemunaitis GA, Kilgore KL. Advanced assessment of the upper limb in tetraplegia: a three-tiered approach to characterizing paralysis. Top Spinal Cord Inj Rehabil. 2018;24(3):206–216.
- Kayagil TA, Grimes JP, Grill WM. Mechanisms underlying reversal of motor unit activation order in electrically evoked contractions after spinal cord injury. Muscle Nerve. 2008 Feb;37(2):210–218.
- Bawa P, Binder MD, Ruenzel P, et al. Recruitment order of motoneurons in stretch reflexes is highly correlated with their axonal conduction velocity. J Neurophysiol. 1984 Sep;52(3):410–420.
- Scott TR, Haugland M. Command and control interfaces for advanced neuroprosthetic applications. Neuromodulation. 2001 Oct;4(4):165–175.
- Keith MW, Kilgore KL, Peckham PH, et al. Tendon transfers and functional electrical stimulation for reconstruction of hand function in spinal cord injury. J Hand Surg. 1996;21a:89–99.
- Kilgore KL, Bryden AM, Keith MW, et al. Evolution of neuroprosthetic approaches to restoration of upper limb function in spinal cord injury. Top Spinal Cord Inj Rehabil. 2018;24(3):252–264.
- Keith MW, Peljovich A. Surgical treatments to restore function control in spinal cord injury. Handb Clin Neurol. 2012;109:167–179. Review.
- van Zyl N, Hill B, Cooper C, et al., Expanding traditional tendon-based techniques with nerve transfers for the restoration of upper limb function in tetraplegia: a prospective case series. Lancet. 2019 Jul 4:pii: S0140-6736(19)31143–2. [Epub ahead of print]. DOI:10.1016/S0140-6736[19)31143-2.
- Keith MW, Peckham PH, Thrope GB, et al. Implantable functional neuromuscular stimulation in the tetraplegic hand. J Hand Surg. 1989;14A:524–530.
- Kilgore KL, Peckham PH, Thrope GB, et al. Synthesis of hand grasp using functional neuromuscular stimulation. IEEE Trans BME. 1989;BME-36(7):761–770.
- Johnson MW, Peckham PH. Evaluation of shoulder movement as a command control source. IEEE Trans Biomed Eng. 1990;37:876–885.
- Pancrazio JJ, Chen D, Fertig SJ, et al. Toward neurotechnology innovation: report from the 2005 neural interfaces workshop. Neuromodulation. 2006;9(1):1–7.
- Peckham PH, Keith MW, Kilgore KL, et al. Efficacy of an implanted neuroprosthesis for restoring hand grasp in tetraplegia: a multicenter study. Arch Phys Med Rehabil. 2001;82:1380–8.
- Wuolle KS, Van Doren CL, Thrope GB, et al. Development of a quantitative hand grasp and release test for patients with tetraplegia using a hand neuroprosthesis. J Hand Surg [Am]. 1994;19:209–218.
- Smith B, Tang Z, Johnson M, et al. An externally powered, multichannel, implantable stimulator-telemeter for control of paralyzed muscle. IEEE Trans Rehab Eng. 1998 April;45(4):463–465.
- Kilgore KL, Hoyen HA, Bryden AM, et al. An implanted upper extremity neuroprosthesis utilizing myoelectric control. J Hand Surg. 2008;33A:539–550.
- Memberg WD, Polasek KH, Hart RL, et al. An implanted neuroprosthesis for restoring arm and hand function in people with high level Tetraplegia. Arch Phys Med Rehabil. 2014;95:1201–1211.
- Bouton CE, Shaikhouni A, Annetta NV, et al. Restoring cortical control of functional movement in a human with quadriplegia. Nature. 2016 May 12;533(7602):247–250. Epub 2016 Apr 13.
- Ajiboye AB, Willett FR, Young DR, et al. Restoration of reaching and grasping movements through brain-controlled muscle stimulation in a person with tetraplegia: a proof-of-concept demonstration. Lancet. 2017 May 6;389(10081):1821–1830.
- Sinnott KA, Milburn P, McNaughton H. Factors associated with thoracic spinal cord injury, lesion level and rotator cuff disorders. Spinal Cord. 2000 Dec;38(12):748–753.
- Triolo RJ, Bailey SN, Miller ME, et al. Effects of stimulating hip and trunk muscles on seated stability, posture, and reach after spinal cord injury. Arch Phys Med Rehabil. 2013a;94:1766–1775.
- Triolo RJ, Lombardo LM, Nogan-Bailey S, et al. Effects of trunk stimulation on manual wheelchair propulsion mechanics after spinal cord injury. Arch Phys Med Rehabil. 2013b;94(10):1997–2005. PMID: 23628377, PMC4103696.
- Triolo RJ, Bailey SN, Foglyano K, et al. Long-term technical and clinical performance and user satisfaction of implanted neuroprostheses for upright mobility after paralysis: two to 14-year follow-up. Arch Phys Med Rehabil. 2018;99:289–298.
- Triolo RJ, Bailey SN, Miller ME, et al. Longitudinal performance of a surgically implanted neuroprosthesis for lower extremity exercise, standing, and transfers after spinal cord injury. Arch Phys Med Rehabil. 2012;93(5):896–904. PMID: 22541312, PMC4111081.
- Marsolais EB, Kobetic R. Implantation techniques and experience with percutaneous intramuscular electrodes in the lower extremities. J Rehabil Res Dev. 1986;23(3):1–8.
- Triolo RJ, Liu MQ, Kobetic R, et al. Selectivity of intramuscular stimulating electrodes in the lower extremities. J Rehabil Res Dev. 2000;38(5):533–544.
- Davis JA, Triolo RJ, Uhlir JP, et al. Surgical technique for installing an 8-channel neuroprosthesis for standing. Clini Orthop Rel Res. 2001a;385:237–252.
- Davis JA Jr., Triolo RJ, Uhlir J, et al. Preliminary performance of a surgically implanted neuroprosthesis for standing and transfers–where do we stand? J Rehabil Res Dev. 2001b;38:609–617.
- Kukke S, Triolo RJ. The effects of trunk stimulation on bimanual seated workspace. IEEE Trans Neural Syst Biomed Eng. 2004;12(2):117–185.
- Glenn WW, Brouillette RT, Dentz B, et al. Fundamental considerations in pacing of the diaphragm for chronic ventilatory insufficiency: a multi-center study. Pacing Clin Electrophysiol. 1988 Nov;11(11 Pt 2):2121–2127.
- Khong P, Lazzaro A, Mobbs R. Phrenic nerve stimulation: the Australian experience. J Clin Neurosci. 2010 Feb;17(2):205–208. Epub 2010 Jan 6.
- Romero FJ, Gambarrutta C, Garcia-Forcada A, et al. Long-term evaluation of phrenic nerve pacing for respiratory failure due to high cervical spinal cord injury. Spinal Cord. 2012 Dec;50(12):895–898. Epub 2012 Jul 10.
- Elefteriades JA, Quin JA, Hogan JF, et al. Long-term follow-up of pacing of the conditioned diaphragm in quadriplegia. Pacing Clin Electrophysiol. 2002 Jun;25(6):897–906.
- Glenn WW, Phelps ML, Elefteriades JA, et al. Twenty years of experience in phrenic nerve stimulation to pace the diaphragm. Pacing Clin Electrophysiol. 1986 Nov;9(6):780–784.
- Le Pimpec-Barthes F, Legras A, Arame A, et al. Diaphragm pacing: the state of the art. J Thorac Dis. 2016 Apr;8(Suppl 4):S376–86. Review.
- DiMarco AF, Onders RP, Ignagni A, et al. Phrenic nerve pacing via intramuscular diaphragm electrodes in tetraplegic subjects. Chest. 2005;127:671–678.
- Onders RP, Elmo M, Khansarinia S, et al. Complete worldwide operative experience in laparoscopic diaphragm pacing: results and differences in spinal cord injured patients and amyotrophic lateral sclerosis patients. Surg Endosc. 2009 Jul;23(7):1433–1440. Epub 2008 Dec 6.
- DiMarco AF, Kowalski KE, Geertman RT, et al. Lower thoracic spinal cord stimulation to restore cough in patients with spinal cord injury: results of a National Institutes of Health-sponsored clinical trial. Part II: clinical outcomes. Arch Phys Med Rehabil. 2009b;90:726–732.
- DiMarco AF, Kowalski KE, Geertman RT, et al. Lower thoracic spinal cord stimulation to restore cough in patients with spinal cord injury: results of a National Institutes of Health-sponsored clinical trial. Part I: methodology and effectiveness of expiratory muscle activation. Arch Phys Med Rehabil. 2009a;90:717–725.
- DiMarco AF, Geertman RT, Tabbaa K, et al. Case report: minimally invasive method to activate the expiratory muscles to restore cough. J Spinal Cord Med. 2018 Sep;41(5):562–566. Epub 2017 Oct 11.
- Brindley GS. The first 500 patients with sacral anterior root stimulator implants: general description. Paraplegia. 1994 Dec;32(12):795–805.
- Creasey GH, Grill JH, Korsten M, et al. An implantable neuroprosthesis for restoring bladder and bowel control to patients with spinal cord injuries: A multi-center trial. Arch Phys Med Rehab. 2001;82:1512–1519.
- Creasey GH, Dahlberg JE. Economic consequences of an implanted neuroprosthesis for bladder and bowel management. Arch Phys Med Rehabil. 2001 Nov;82(11):1520–1525.
- Lee Y-H, Kim S-H, Kim JM, et al. The effect of semiconditional dorsal penile nerve electrical stimulation on capacity and compliance of the bladder with deformity in spinal cord injury patients: a pilot study. Spinal Cord. 2012;50(4):289–293.
- Lee Y-H, Creasey GH. Self-controlled dorsal penile nerve stimulation to inhibit bladder hyperreflexia in incomplete spinal cord injury: a case report. Arch Phys Med Rehabil. 2002;83(2):273–277.
- Bourbeau DJ, Creasey GH, Sidik S, et al. Genital nerve stimulation increases bladder capacity after SCI: a meta-analysis. J Spinal Cord Med. 2018;41:426-434.
- Bourbeau DJ, Gustafson KJ, Brose SW. At-home genital nerve stimulation for individuals with SCI and neurogenic Detrusor Overactivity: A Pilot Feasibility Study. J Spinal Cord Med. 2018 January 1–11. DOI:10.1080/10790268.2017.1422881.
- Ho CH, Triolo RJ, Elias AL, et al. Functional Electrical Stimulation and Spinal Cord Injury. Phys Med Rehabil Clin N Am. 2014;25(3):631–654.
- Peckham PH, Kilgore KL. Challenges and opportunities in restoring function after paralysis. IEEE Trans BME. 2013;60(3):602–609.
- Creasey G, Kilgore KL, Brown-Triolo DL, et al. Reduction of costs of disability using neuroprostheses. Assistive Technol. 2000;12:67–75.