ABSTRACT
Objective: Decompression surgery in patients with spinal cord injury (SCI) has a neuroprotective effect by alleviating secondary injury and improving neurological outcomes. N-Acetylcysteine (NAC), a drug approved by the United States Food and Drug Administration, has been shown to play neuroprotective roles via attenuation of apoptosis and inflammation. The purpose of the present study was to investigate the effects of early or late decompression surgery in combination with NAC administration on acute SCI, as well as investigate the underlying mechanisms of its actions. Methods: In this study, an acute SCI model was established in rats. The rats were treated with decompression surgery 24/48 h post-SCI in combination with or without NAC. Results: The results showed that decompression surgery in combination with NAC lead to a better outcome than decompression alone, as demonstrated by the higher Basso, Beattie, and Bresnahan scores. Histopathological examination demonstrated that early decompression surgery in combination with NAC exerted the best therapeutic effect on spinal cord recovery, which was further confirmed by the extent of inflammation and apoptosis. Additionally, we found that NAC might compensate for a lack of late surgery. Conclusions: Collectively, early decompression surgery and NAC could be a promising combination for the treatment of acute SCI, and its therapeutic effects may be associated with the regulation of inflammation and apoptosis.
Introduction
Spinal cord injury (SCI) is a global condition with high morbidity and disability rates caused by compression, contusion, traumatic accidents, or lacerations. Epidemiological data shows that in 2018, the incidence of traumatic SCI was 10.5 cases per 100,000 people worldwide [Citation1,Citation2]. Although significant efforts have been made to treat acute SCI, failure persists because of the high impact and complex pathophysiology of SCI [Citation3–6]. Recently, multiple reports have found that surgical decompression exerts neuroprotective effects in SCI patients by alleviating secondary injury and improving neurological outcomes [Citation7–11]. However, the influence of decompression timing on SCI outcomes remains unclear [Citation12,Citation13]. In addition, decompression alone cannot cure SCI, and it is necessary to improve SCI treatment by combining decompression with effective adjunctive therapies [Citation14]. Overall, the combination of decompression and neurosurgery is an important treatment tool [Citation15].
The pathophysiology of SCI involves both primary and secondary injuries. Secondary injury occurs in response to a progressive local cascade of tissue disruption, with changes in the microenvironment around the damaged spinal cord, such as inflammation, edema, microcirculation ischemia, and apoptosis. N-Acetylcysteine (NAC) is a safe and well-tolerated drug that has been approved by the United States Food and Drug Administration, which has been used in the treatment of numerous disorders, such as paracetamol intoxication [Citation16,Citation17]. NAC has shown neuroprotective effects and significantly attenuates the death of motor neurons after both adult and neonatal nerve injury [Citation18,Citation19]. Kamboj et al. found that NAC is involved in the inhibition of diabetic neuropathy through the attenuation of apoptosis and oxidative stress [Citation20]. The combination of NAC with allopurinol prevented ischemia/reperfusion injury in the spinal cord by inhibiting degeneration, edema, and inflammation of spinal cord tissues [Citation21]. In addition, NAC has shown a positive but limited influence on SCI [Citation22].
To date, the effect of decompression in combination with NAC on acute SCI has rarely been reported. In this study, an acute SCI model was constructed in rats to elucidate the underlying mechanisms of surgical decompression and NAC in acute SCI. The aim of this study was to determine the optimum timing for decompression surgery and reveal new insights into the use of decompression surgery in combination with NAC in the clinical treatment of SCI.
Materials and Methods
Animals and SCI model construction
A total of 150 female Sprague-Dawley rats weighing 200 ± 10 g (aged 6–7 weeks) were supplied by Liaoning Changsheng Biotechnology Co., Ltd. All rats were adaptively maintained for one week before surgery in a temperature-regulated room (22–25 °C) on a 12 h light/dark cycle with food and water available ad libitum. The SCI model was constructed in rats according to methods previously reported [Citation23]. Rats subjected to SCI were randomly divided into four groups: decompression surgery 8 h post-SCI (DEC-8), decompression surgery 48 h post-SCI (DEC-48), decompression surgery 8 h post-SCI in combination with NAC (DEC-8+ NAC), and decompression surgery 48 h post-SCI in combination with NAC (DEC-48+ NAC). The rats in the DEC-8 and DEC-48 groups were only treated with decompression surgery at 8 h and 48 h post-SCI, respectively. The rats in the DEC-8+ NAC and DEC-48+ NAC groups were treated with decompression surgery 8 h and 48 h post-SCI, respectively, followed by intraperitoneal injection of NAC (50 mg/kg) [Citation24] for four weeks, once every two days. After treatment, the rats were maintained in a temperature-regulated room (22–25 °C) on a 12 h light/dark cycle with food and water available ad libitum for 3 or 60 days before being euthanized to obtain spinal cord tissues. Portions of the spinal cord tissues were maintained at −80 °C, and the remainder were fixed in 4 % paraformaldehyde for histological analysis.
Behavioral analyses
The motor behavior of rats was evaluated every three days using the Basso, Beattie, and Bresnahan (BBB) locomotor rating scale (n = 10 per group). The rats were allowed to move freely in an open field and were scored using criteria such as ability to support weight, physical coordination, extent of joint movement, and stepping behavior of hind limbs. Rats in the control group did not show any impairment in motor behavior. Functional scores ranging from 0 (without any hind limb movement) to 21 (normal movement) were assigned to two individuals. Scoring was performed for a period of 4 min following the guidelines of the Spinal Cord Injury Project (WM Keck Center for Collaborative Neuroscience, Rutgers State University of NJ, USA). The mean scores for each group were calculated.
Histological analysis
Nissl staining was performed to evaluate neuronal survival. Spinal cord sections (4 μm in thickness) were rehydrated using a graded series of ethanol and double-distilled water and stained with cresyl violet (Bioswamp, Wuhan, China) at 56 °C for 1 h. After washing with water and differentiation in 95 % ethanol for 1 min, the slides were dehydrated in 100 % ethyl alcohol, immersed in xylene, and mounted with neutral balsam. The sections were observed under an optical microscope (Leica, Wetzlar, Germany).
Luxol fast blue (LFB) staining was performed to observe the nerve myelin sheath. The sections were rehydrated using a graded series of ethanol and immersed in 0.1 % LFB staining solution for 3 h at 65 °C, followed by alternate differentiation for 20 min in 70 % ethanol and 0.05 % lithium carbonate. The slides were dehydrated in 100 % ethanol, immersed in xylene, mounted with neutral balsam, and visualized under an optical microscope (Leica).
For the immunohistochemical analysis of neurofilaments (NF)-200, F4/80, and the glial fibrillary acidic protein (GFAP), the sections were rehydrated using a graded series of ethanol (100 %, 95 %, 85 %, 75 %) and double-distilled water. The sections were immersed in 0.01 M sodium citrate buffer at 125 °C at 103 kPa for 15 min for antigen retrieval. After blocking in 3 % H2O2 for 10 min, the sections were incubated with primary antibodies against NF-200 (Proteintech, Wuhan, China), F4/80 (ThermoFisher, Shanghai, China), and GFAP (Proteintech) overnight at 4 °C. This was followed by incubation with MaxVision TM HRP-Polymer anti-Mouse/Rabbit IHC secondary antibody (Bioswamp) for 30 min at room temperature. Thereafter, the slides were stained with diaminobenzidine (Bioswamp) and counterstained with hematoxylin (Bioswamp). Image analysis was performed using the Leica MD1000 apparatus.
Terminal deoxynucleotidyl transferase dUTP nick end labeling (TUNEL) staining was performed to evaluate apoptosis, according to the manufacturer’s instructions. After dewaxing with xylene and hydration in a graded series of ethanol (100 %, 95 %, 85 %, 75 %), the sections were incubated in proteinase K solution for 20 min at room temperature, followed by incubation with 50 µL of TUNEL reaction mixture in a dark, humid chamber at 37 °C for 1 h. The sections were then incubated with 50 μl of converter-POD in the dark at 37 °C for 1 h and diaminobenzidine for 10 min. Nuclei were counterstained with hematoxylin. Image analysis was performed using the Leica MD1000 apparatus.
Enzyme-linked immunosorbent assay
Enzyme-linked immunosorbent assay (ELISA) was conducted to measure the levels of interleukin (IL)-1μ, IL-6, chemokine (C-C motif) ligand 2 (CCL2), CCL3, CCL4, chemokine (C-X-C motif) ligand 1 (CXCL1), CXCL2, tumor necrosis factor (TNF)-α, cyclooxygenase 2 (COX-2), and inducible nitric oxide synthase (iNOS) in spinal cord tissues, according to the kit manufacturer’s instructions (Bioswamp).
Flow cytometry of inflammatory cell infiltration
A mononuclear suspension of 1 × 107 cells was collected from the spinal cord and stained with 2 μl of fluorescence-conjugated CD11b (BD, Shanghai, China) and CD45 (BD Biosciences) for 30 min at 4 °C. The cells were then stained with fluorescein-conjugated goat anti-rabbit IgG (H + L) highly cross-adsorbed secondary antibody (Invitrogen) for 30 min at 4 °C and subjected to flow cytometry (ACEA Biosciences, San Diego, CA, USA).
Western blot
Total proteins in the spinal cord were homogenized in radioimmunoprecipitation assay lysis buffer and centrifuged at 12,000 × g at 4 °C for 15 min. The supernatants were collected, and the protein concentration was quantified using a bicinchoninic acid kit (Bioswamp). Proteins (20 μg) were separated by 12 % sodium dodecyl sulfate-polyacrylamide gel electrophoresis and transferred onto polyvinylidene fluoride membranes (Millipore, Massachusetts, USA). The membranes were blocked with 5 % skimmed milk overnight at 4 °C. Next, the membranes were incubated with primary antibodies against cleaved caspase-3 (Abcam, Cambridge, UK) and GAPDH (Bioswamp) for 1 h at room temperature, followed by incubation with horseradish peroxidase-labeled goat anti-rabbit IgG secondary antibody (Bioswamp) for 1 h at 25°C. After treatment with an enhanced chemiluminescence substrate buffer (Millipore), the membranes were visualized using a Tanon-5200 apparatus (Tanon, Shanghai, China).
Statistical analysis
Statistical analysis was performed using SPSS software version 19.0, and the data are presented as the mean ± standard deviation. Differences between two groups were analyzed using the Student’s t-test, and those between more than two groups were analyzed using one-way analysis of variance followed by least significant difference. Statistical significance was set at p < 0.05.
Results
NAC promotes locomotor ability of rats with SCI after decompression surgery
Locomotor ability was evaluated using the BBB motor rating scale [Citation25], and the results are shown in . The rats in the control group showed a BBB score of approximately 21, indicating no impairment of motor behavior. Compared with the DEC groups, the BBB scores in the corresponding DEC+NAC combination treatment groups increased. The results demonstrate that NAC treatment improved motor recovery in rats with SCI after decompression surgery.
NAC alleviates central nerve damage in rats with SCI after decompression surgery
Sixty days after decompression surgery with or without NAC, the extent of spinal cord damage in rats was assessed. LFB staining was performed to observe the nerve myelin sheath. Nissl staining was performed to evaluate the neuronal survival. GFAP was used as an indication of glial scar formation and astrocyte activity. Immunohistochemical staining with NF-200 was performed to evaluate the number of nerve fibers. Compared with control rats, the area of the nerve myelin sheath and the number of NF-200-positive cells and Nissl bodies were both decreased in rats with SCI, whereas the number of GFAP-positive astrocytes was increased (). Compared with decompression 48 h post-SCI, 8 h post-SCI decompression led to an increase in the area of myelination and the number of NF-200-positive cells and Nissl bodies, which were further increased by combination treatment of decompression 8 h post-SCI and NAC. However, compared with decompression 48 h post-SCI, 8 h post-SCI decompression showed a decrease in the number of GFAP-positive astrocytes, which was further decreased by combined treatment with decompression 8 h post-SCI and NAC. These results demonstrate that early decompression is a superior option for acute SCI treatment compared with late decompression. In addition, the combination of early decompression with NAC was superior to decompression alone.
NAC attenuates inflammation in rats with SCI after decompression surgery
Three days after decompression surgery with or without NAC, the spinal cord tissues of post-SCI rats were collected to evaluate their inflammatory response. Immunohistochemical staining with F4/80, a marker of macrophages, was performed to detect the presence of macrophages, and cells labeled for CD11b and CD45 were quantified using flow cytometry. As shown in , the number of F4/80-positive cells and the percentage of CD11b+ and CD45+ cells was increased in rats with SCI. Compared with decompression 48 h post-SCI, 8 h post-SCI decompression resulted in a decrease in the number of F4/80-positive cells and the percentage of CD11b- and CD45-labeled cells, which was further decreased by combination treatment with NAC.
Figure 3. NAC attenuates inflammatory cell infiltration on the third day of treatment after decompression surgery in rats with SCI. Immunohistochemical analysis of F4/80 and detection of CD11b- and CD45-labeled cells. Data presented as mean ± SD, n = 3, *p < 0.05. Scale bar = 50 μm.
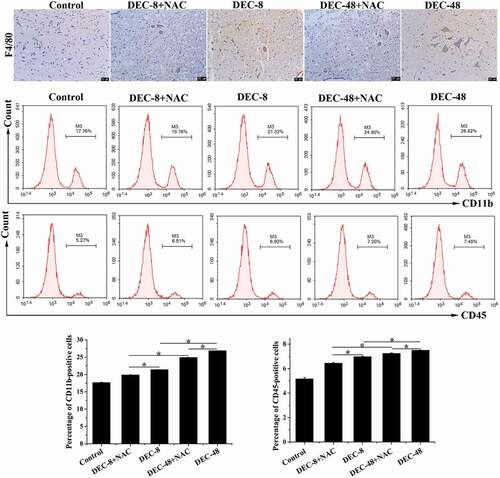
Additionally, the levels of pro-inflammatory cytokines and chemokines were measured (). Compared with decompression 48 h post-SCI, 8 h post-SCI decompression induced a decrease in the expression of IL-1β, IL-6, TNF-α, CXCL1, CXCL1, CCL2, CCL3, CCL4, iNOS, and COX-2, which was further decreased by combination treatment with NAC.
NAC attenuates apoptosis in rats with SCI after decompression surgery
TUNEL staining demonstrated that no apoptotic cells were present in the control rats (). Apoptotic cells were identified in rats subjected to SCI. Compared with the two decompression groups, the number of apoptotic cells was decreased with the combined treatment of decompression and NAC. Western blotting was performed to assess the expression of the pro-apoptotic protein caspase-3. The results indicated that, compared with decompression 48 h post-SCI, the 8 h post-SCI decompression group showed a decrease in the expression of cleaved caspase-3, which was further decreased by combination treatment with NAC.
Figure 5. NAC attenuates apoptosis in rats with SCI treatment for 2 months after decompression surgery. (A) TUNEL staining and (B) protein expression of cleaved caspase-3 in injured spinal cords. Data presented as mean ± SD, n = 3, *p < 0.05. Scale bar = 50 μm.
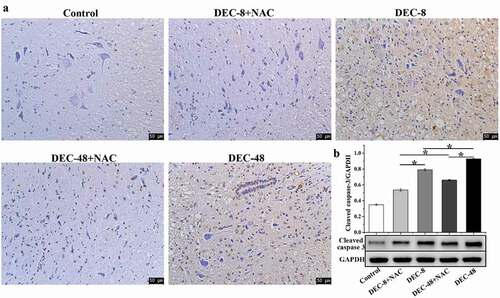
These data suggest that early decompression combined with NAC protected rats from SCI by suppressing apoptosis.
Discussion
Despite numerous preclinical studies investigating the pathophysiology of acute SCI and potential treatments to improve the outcome of SCI, the need for targeted therapeutic options that reach clinical practice is still evident.
Current clinical methods in acute SCI therapy include surgical decompression, stabilization, and aggressive intensive care measures, followed by blood pressure augmentation to alleviate secondary damage [Citation26–28]. Accumulating research has demonstrated that early surgical decompression treatment (<24 h post-SCI) improves neurological outcomes [Citation29]. One study included a total of 313 patients, of which 182 underwent early surgery and 131 underwent late surgery (≥24 h post-SCI), and those treated with early surgery showed a greater rate of improvement of ≥2 on the abbreviated injury scale grades (odds ratio [OR]: 2.57; 95 % confidence interval [CI]: 1.11, 5.97) at a 6-month follow-up [Citation30]. A systematic search of 422 patients with complete cervical SCI revealed that their improvement was greater after early decompression surgery than after late surgery (early: 22.6 %, 95 % CI: 16.6–28.7 %; late: 10.4 %, 95 % CI: 5.6–15.8 %; OR 2.6 [95 % CI: 1.4–5.1]) [Citation8]. In this study, the results indicated that, compared to late decompression surgery (48 h post-SCI), early decompression surgery (8 h post-SCI) attenuated SCI-induced nerve damage, inflammatory response, and apoptosis, as demonstrated by the increase in nerve myelin sheath area, NF-200-positive cells, and Nissl bodies and a decrease in GFAP-positive astrocytes, TUNEL-positive cells, pro-inflammatory cytokines, and pro-apoptotic protein expression.
Secondary injury caused by inflammation, oxidative stress, and apoptosis after SCI is one of the key targets in SCI treatment [Citation31], occurring seconds after primary injury and continuing for several weeks [Citation32]. Neuroinflammation mediated by the host immune response and molecules such as chemokines and inflammatory cytokines promote neurodegenerative processes within the injured spinal cord [Citation33]. Numerous basic and clinical studies have demonstrated that apoptosis plays a vital role in determining neurological outcomes after SCI [Citation34]. The mechanism of decompression surgery in relieving SCI involves the inhibition of the inflammatory response [Citation35], which is consistent with the present study.
NAC, a natural antioxidant found in onions, has been utilized as a drug since the 1960s and is listed on the World Health Organization Model List of Essential Medicines as an antidote against poisoning [Citation36]. There are various proposed functions of NAC in medicine that remain under clinical or preclinical investigation. NAC has been used in the treatment of numerous disorders, including cardiac ischemia-reperfusion injury [Citation37], chronic obstructive pulmonary disease [Citation38], psychiatric disorders [Citation39], and inflammatory bowel disease [Citation40]. In addition, evidence has demonstrated the immunomodulatory activity of NAC, which improved immune response in ways such as leukocyte chemotaxis and decreasing the levels of TNF-α, IL-1β, and leukocyte infiltration in inflamed tissues [Citation41,Citation42]. NAC showed anti-inflammatory activity by regulating nuclear factor-kappa B activation and the subsequent production of inflammation-related factors and chemokines [Citation43–45], whereas Khashayar et al. experimentally demonstrated that inhibition of inflammatory factors improved motor activity and neuropathic pain in SCI rats [Citation46]. Additionally, previous experiments have suggested that NAC is involved in the modulation of cell cycle progression and apoptosis. It has been shown that reducing caspase-3 expression promotes functional recovery in SCI rats [Citation47–49]. Hu et al. [Citation50] found that NAC attenuated fluoride-induced apoptosis via the inhibition of Ire1α-JNK, which is associated with the blockage of caspase-3 cleavage. Li et al. [Citation51] revealed that NAC produced neuroprotective effects by inhibiting the expression of caspase-3, thereby inhibiting apoptosis. In this study, compared to decompression surgery alone, surgery with NAC exhibited a significant neuroprotective effect, as demonstrated by the alleviation of inflammation and apoptosis through the regulation of their corresponding factors. Decompression surgery in combination with NAC treatment decreased the number of macrophages and leukocytes in SCI rats, as indicated by the decrease in the number of F4/80-positive cells and the percentage of CD11b- and CD45-labeled cells. In other words, decompression surgery in combination with NAC treatment decreased inflammatory cell infiltration compared to decompression surgery alone in SCI rats. We were surprised to find no significant difference between MOD-DEC-8 and MOD-DEC-48+ NAC in some outcomes, such as BBB score, HE staining, TUNEL staining, and Nissl staining, and we speculated whether NAC could compensate for late decompression surgery. However, in the present study, we did not investigate the effect of NAC in rats with SCI prior to decompression surgery after SCI. This will be the focus of future studies to further demonstrate the effect of NAC on SCI.
Conclusion
In conclusion, early decompression surgery in combination with NAC is a superior option for SCI treatment compared to decompression surgery alone or late decompression surgery in combination with NAC. Combined early decompression and NAC showed neuroprotective effects through the inhibition of inflammatory responses and apoptosis in SCI rats. The present findings may provide new insights into SCI intervention.
Author contributions
Xing Guo, Zhiyong Niu, and Junjie Song conceived the study. Xing Guo, Jindong He, Rongping Zhang, Tiechui Wang, Jinjin Chen, and Jinyu Wang performed the experiments. Xing Guo, Zihang Wang, Guan Chang, and Yubo Niu analyzed the data. Xing Guo drafted the manuscript. All authors have read and agreed to the published version of the manuscript.
Disclosure statement
The authors have no commercial or other associations that might pose a conflict of interest.
Additional information
Funding
References
- Kumar R, Lim J, Mekary RA, et al. Traumatic Spinal Injury: global Epidemiology and Worldwide Volume. World Neurosurg. 2018 May;113:e345–e363.
- Deng Q, Ma L, Chen T, et al. NF-κB 1-induced LINC00665 regulates inflammation and apoptosis of neurons caused by spinal cord injury by targeting miR-34a-5p. Neurol Res. 2021 May;43(5):418–427.
- Rowland JW, Hawryluk GW, Kwon B, et al. Current status of acute spinal cord injury pathophysiology and emerging therapies: promise on the horizon. Neurosurg Focus. 2008;25(5):E2.
- Curt A, Van Hedel HJ, Klaus D, et al. Recovery from a spinal cord injury: significance of compensation, neural plasticity, and repair. J Neurotrauma. 2008 Jun;25(6):677–685.
- Aarabi B, Akhtar-Danesh N, Chryssikos T, et al. Efficacy of Ultra-Early (< 12 h), Early (12-24 h), and Late (>24-138.5 h) Surgery with Magnetic Resonance Imaging-Confirmed Decompression in American Spinal Injury Association Impairment Scale Grades A, B, and C Cervical Spinal Cord Injury. J Neurotrauma. 2020;37(3):448–457.
- Yi Kang HD, Zhou H, Wei Z, et al. Epidemiology of worldwide spinal cord injury: a literature review. J Neurorestoratol. 2018;6:1–9.
- Ahuja CS, Wilson JR, Nori S, et al. Traumatic spinal cord injury. Nat Rev Dis Primers. 2017 Apr 27 3(1):17018.
- Ter Wengel PV, Witt Hamer PC D, Pauptit JC, et al. Early Surgical Decompression Improves Neurological Outcome after Complete Traumatic Cervical Spinal Cord Injury: a Meta-Analysis. J Neurotrauma. 2019 Mar 19 36(6):835–844.
- Du JP, Fan Y, Zhang JN, et al. Early versus delayed decompression for traumatic cervical spinal cord injury: application of the AOSpine subaxial cervical spinal injury classification system to guide surgical timing. Eur Spine J. 2019 Aug;28(8):1855–1863.
- Lee DY, Park YJ, Song SY, et al. The Importance of Early Surgical Decompression for Acute Traumatic Spinal Cord Injury. Clin Orthop Surg. 2018 Dec;10(4):448–454.
- Xie JB, Zhang X, Li QH, et al. Inhibition of inflammatory cytokines after early decompression may mediate recovery of neurological function in rats with spinal cord injury. Neural Regen Res. 2015 Feb;10(2):219–224.
- Wilson JR, Tetreault LA, Kwon BK, et al. Timing of Decompression in Patients With Acute Spinal Cord Injury: a Systematic Review. Global Spine J. 2017 Sep;7(3 Suppl):95S–115S.
- Furlan JC, Craven BC, Massicotte EM, et al. Early Versus Delayed Surgical Decompression of Spinal Cord after Traumatic Cervical Spinal Cord Injury: a Cost-Utility Analysis. World Neurosurg. 2016 Apr;88:166–174.
- Wang YL, Qi YN, Wang W, et al. Effects of decompression joint Governor Vessel electro-acupuncture on rats with acute upper cervical spinal cord injury. Neural Regen Res. 2018 Jul;13(7):1241–1246.
- Zhu F, Yao S, Ren Z, et al. Early durotomy with duroplasty for severe adult spinal cord injury without radiographic abnormality: a novel concept and method of surgical decompression. Eur Spine J. 2019 Oct;28(10):2275–2282.
- Elbini Dhouib I, Jallouli M, Annabi A, et al. A minireview on N-acetylcysteine: an old drug with new approaches. Life Sci. 2016 Apr 15;151:359–363.
- Zhou Y, Wang HD, Zhou XM, et al. N-acetylcysteine amide provides neuroprotection via Nrf2-ARE pathway in a mouse model of traumatic brain injury. Drug Des Devel Ther. 2018;12:4117–4127.
- Welin D, Novikova LN, Wiberg M, et al. Effects of N-acetyl-cysteine on the survival and regeneration of sural sensory neurons in adult rats. Brain Res. 2009 Sep 1;1287:58–66.
- Catapano J, Zhang J, Scholl D, et al. N-Acetylcysteine Prevents Retrograde Motor Neuron Death after Neonatal Peripheral Nerve Injury. Plast Reconstr Surg. 2017 May;139(5):1105e–1115e.
- Kamboj SS, Vasishta RK, Sandhir R. N-acetylcysteine inhibits hyperglycemia-induced oxidative stress and apoptosis markers in diabetic neuropathy. J Neurochem. 2010 Jan;112(1):77–91.
- Erkut B, Onk OA. Effect of N-acetylcysteine and allopurinol combination to protect spinal cord ischemia/reperfusion injury induced by aortic cross-clamping in rat model. J Cardiothorac Surg. 2015 Jul 8; 10(1):95.
- Olakowska E, Marcol W, Wlaszczuk A, et al. The neuroprotective effect of N-acetylcysteine in spinal cord-injured rats. Adv Clin Exp Med. 2017 Dec;26(9):1329–1334.
- Huang WL, George KJ, Ibba V, et al. The characteristics of neuronal injury in a static compression model of spinal cord injury in adult rats. Eur J Neurosci. 2007 Jan;25(2):362–372.
- Al-Samhari MM, Al-Rasheed NM, Al-Rejaie S, et al. Possible involvement of the JAK/STAT signaling pathway in N-acetylcysteine-mediated antidepressant-like effects. Exp Biol Med (Maywood). 2016 Mar;241(5):509–518.
- Basso DM, Beattie MS, Bresnahan JC. A sensitive and reliable locomotor rating scale for open field testing in rats. J Neurotrauma. 1995 Feb;12(1):1–21.
- Badhiwala JH, Ahuja CS, Fehlings MG. Time is spine: a review of translational advances in spinal cord injury. J Neurosurg Spine. 2018 Dec 20; 30(1):1–18.
- Hadley MN, Walters BC. Introduction to the Guidelines for the Management of Acute Cervical Spine and Spinal Cord Injuries. Neurosurgery. 2013 Mar;72 Suppl 2(suppl_3):5–16.
- Fehlings MG, Tetreault LA, Wilson JR, et al. A Clinical Practice Guideline for the Management of Acute Spinal Cord Injury: introduction, Rationale, and Scope. Global Spine J. 2017 Sep;7(3 Suppl):84S–94S.
- Tanaka C, Tagami T, Kaneko J, et al. Early versus late surgery after cervical spinal cord injury: a Japanese nationwide trauma database study. J Orthop Surg Res. 2019 Sep 5 14(1):302.
- Karsy M, Hawryluk G. Modern Medical Management of Spinal Cord Injury. Curr Neurol Neurosci Rep. 2019 Jul 30; 19(9):65.
- Wang XJ, Shu GF, Xu XL, et al. Combinational protective therapy for spinal cord injury medicated by sialic acid-driven and polyethylene glycol based micelles. Biomaterials. 2019 Oct;217:119326.
- Wilson JR, Forgione N, Fehlings MG. Emerging therapies for acute traumatic spinal cord injury. CMAJ. 2013 Apr 2; 185(6):485–492.
- Stahel PF, VanderHeiden T, Finn MA. Management strategies for acute spinal cord injury: current options and future perspectives. Curr Opin Crit Care. 2012 Dec;18(6):651–660.
- Yu WR, Liu T, Kiehl TR, et al. Human neuropathological and animal model evidence supporting a role for Fas-mediated apoptosis and inflammation in cervical spondylotic myelopathy. Brain. 2011 May;134(Pt 5):1277–1292.
- Jiang Y, Wang F, Yu X, et al. A Comparative Study on Functional Recovery, Complications, and Changes in Inflammatory Factors in Patients with Thoracolumbar Spinal Fracture Complicated with Nerve Injury Treated by Anterior and Posterior Decompression. Med Sci Monit. 2019;25:1164–1168.
- Salamon S, Kramar B, Marolt TP, et al. Medical and Dietary Uses of N-Acetylcysteine. Antioxidants (Basel). 2019 Apr 28;8(5). https://doi.org/10.3390/antiox8050111.
- Baker WL, Anglade MW, Baker EL, et al. Use of N-acetylcysteine to reduce post-cardiothoracic surgery complications: a meta-analysis. Eur J Cardiothorac Surg. 2009 Mar;35(3):521–527.
- Sadowska AM, Manuel YKB, De Backer WA. Antioxidant and anti-inflammatory efficacy of NAC in the treatment of COPD: discordant in vitro and in vivo dose-effects: a review. Pulm Pharmacol Ther. 2007;20(1):9–22.
- Berk M, Malhi GS, Gray LJ, et al. The promise of N-acetylcysteine in neuropsychiatry. Trends Pharmacol Sci. 2013 Mar;34(3):167–177.
- Moura FA, de Andrade KQ, Dos Santos JCF, et al. Antioxidant therapy for treatment of inflammatory bowel disease: does it work? Redox Biol. 2015 Dec;6:617–639.
- Gao X, Lampraki EM, Al-Khalidi S, et al. N-acetylcysteine (NAC) ameliorates Epstein-Barr virus latent membrane protein 1 induced chronic inflammation. PLoS One. 2017;12(12):e0189167.
- Samuni Y, Goldstein S, Dean OM, et al. The chemistry and biological activities of N-acetylcysteine. Biochim Biophys Acta. 2013 Aug;1830(8):4117–4129.
- Csontos C, Rezman B, Foldi V, et al. Effect of N-acetylcysteine treatment on oxidative stress and inflammation after severe burn. Burns. 2012 May;38(3):428–437.
- Paterson RL, Galley HF, Webster NR. The effect of N-acetylcysteine on nuclear factor-kappa B activation, interleukin-6, interleukin-8, and intercellular adhesion molecule-1 expression in patients with sepsis. Crit Care Med. 2003 Nov;31(11):2574–2578.
- Kim WH, An HJ, Kim JY, et al. Apamin inhibits TNF-alpha- and IFN-gamma-induced inflammatory cytokines and chemokines via suppressions of NF-kappaB signaling pathway and STAT in human keratinocytes. Pharmacol Rep. 2017 Oct;69(5):1030–1035.
- Afshari K, Dehdashtian A, Haddad NS, et al. Sumatriptan improves the locomotor activity and neuropathic pain by modulating neuroinflammation in rat model of spinal cord injury. Neurol Res. 2021 Jan;43(1):29–39.
- Tao L, Liu X, Da W, et al. Pycnogenol achieves neuroprotective effects in rats with spinal cord injury by stabilizing the mitochondrial membrane potential. Neurol Res. 2020 Jul;42(7):597–604.
- Ji WC, Li M, Jiang WT, et al. Protective effect of brain-derived neurotrophic factor and neurotrophin-3 overexpression by adipose-derived stem cells combined with silk fibroin/chitosan scaffold in spinal cord injury. Neurol Res. 2020 May;42(5):361–371.
- Şaker D, Sencar L, Yılmaz DM, et al. Relationships between microRNA-20a and microRNA-125b expression and apoptosis and inflammation in experimental spinal cord injury. Neurol Res. 2019 Nov;41(11):991–1000.
- Hu Y, Wang Y, Yan T, et al. N-acetylcysteine alleviates fluoride-induced testicular apoptosis by modulating IRE1alpha/JNK signaling and nuclear Nrf2 activation. Reprod Toxicol. 2019 Mar;84:98–107.
- Li J, Meng Z, Zhang G, et al. N-acetylcysteine relieves oxidative stress and protects hippocampus of rat from radiation-induced apoptosis by inhibiting caspase-3. Biomed Pharmacother. 2015;70:1–6.