Abstract
The search for new markers of diseases in human as well as veterinary medicine is ongoing. Recently, microRNAs (miRNAs or miRs) have emerged as potential new biomarkers. MiRNAs are short sequences of RNA (∼22 nucleotides) that regulate gene expression via their target messenger RNA (mRNA). Circulating miRNAs in blood can be used as novel diagnostic markers for diseases due to their evolutionary conservation and stability. As a consequence of their systemic and manifold effects on the gene expression in various target organs, the concept that miRNAs could function as hormones has been suggested. This review summarizes the biogenesis, maturation, and stability of miRNAs and discusses their use as potential biomarkers in equine medicine. To date, over 700 equine miRNAs are identified with distinct subsets of miRNAs differentially expressed in a tissue-specific manner. A physiological involvement of various miRNAs in the regulation of cell survival, steroidogenesis, and differentiation during follicle selection and ovulation in the monovular equine ovary has been demonstrated. Furthermore, miRNAs might be used as novel diagnostic markers for myopathies such as polysaccharide storage myopathy and recurrent exertional rhabdomyolysis as well as osteochondrosis. Preliminary data indicate that miRNAs in blood might play important roles in equine glucose metabolism pathway. Of note, breed differences have been reported regarding the normal equine miRNA signature. For disease prevention, it is of utmost importance to identify disease-associated biomarkers which help detect diseases before symptoms appear. As such, circulating miRNAs represent promising novel diagnostic markers in equine medicine.
1. Introduction
Beyond the ‘traditional’ functions such as gene storage, transport and protein synthesis, recent discoveries reveal that RNAs have important ‘new’ biological functions including RNA silencing and gene regulation. Such functions of noncoding RNAs are strongly coupled to the RNA structures and proper structure change, which naturally leads to the RNA folding problem including structure prediction and folding kinetics (Tan et al. Citation2015). In general, the sizes of the majority of noncoding RNA (ncRNA) species vary from 18 to 500 nucleotides, well below the size of the majority of messenger RNA (mRNA) species, and are therefore termed small ncRNAs (sncRNAs) (Zhang Citation2008).
The term ncRNA is commonly employed for RNA that does not encode a protein, but this does not mean that such RNAs do not contain information or have function (Zhang Citation2008). For example, ribosomal RNAs and transfer RNAs, which make up a large proportion of RNA based on amount, are two known sncRNAs that are critical for protein expression. Recently, two novel classes of sncRNA were discovered: microRNA (miRNA or miR) and small interfering RNA (siRNA) (Erdmann et al. Citation2001; Szymański et al. Citation2003; Mattick & Makunin Citation2006; Zhang Citation2008). MiRNAs and siRNAs have similar sizes (18–23 nucleotides) and share similar mechanisms regarding gene expression regulation. However, their biogenesis and origins are different (Cullen Citation2004; Kim Citation2005a, Citation2005b; Zhang Citation2008). SiRNAs are often of exogenous origin, and usually target sequences at the same locus or elsewhere in the genome for destruction (gene silencing). In contrast, miRNAs are endogenous. They are encoded within the genome and come from endogenous short hairpin precursors and usually target sequences at other loci. Therefore, miRNAs may be more important because they are endogenous regulators of gene expression (Zhang Citation2008).
MiRNAs are small (∼22 nucleotides) non-coding RNA molecules originally misinterpreted as non-sense or junk DNA. Emerging research suggests that these molecules have diverse regulatory roles in an array of molecular, cellular and physiological processes. MiRNAs are versatile and, because of their structure and shortness, highly stable molecules. Therefore, they are able to exist as intracellular or extracellular miRNAs (Nguyen et al. Citation2014).
This review summarizes the biogenesis, maturation, and stability of miRNAs and discusses their use as potential biomarkers in equine medicine.
2. MiRNAs
Genomic evidence reveals that gene expression in humans is precisely controlled in cell-type, tissue, time, and condition-specific manners. To understand the regulatory mechanisms of gene expression as completely as possible is therefore one of the most important issues in genomic medicine. Surprisingly, recent analyses of the human and animal genomes have demonstrated that the majority of RNA transcripts are sncRNAs, rather than large, protein coding mRNAs. Moreover, these sncRNAs may represent an important novel layer of regulation for gene expression. The most important breakthrough in this new area is the discovery of miRNAs. As a group, miRNAs may directly regulate approximately 30% of the genes in the human genome. In keeping with the nomenclature of RNomics, which is to study sncRNAs on the genomic scale, ‘microRNomics’ was coined by Zhang (Citation2008) to describe a novel subdiscipline of genomics that studies the identification, expression, biogenesis, structure, regulation of expression, targets, and biological functions of miRNAs on the genomic scale (Zhang Citation2008). Since the discovery of the founding members of the miRNA class, lin-4 and let-7 in Caenorhabditis elegans, over 2000 miRNA sequences have been described in vertebrates, flies, worms and plants, and even in viruses (Griffiths-Jones et al. Citation2006).
2.1. Structure
The highly conserved miRNAs form a class of small single stranded noncoding RNAs whose final product is a ∼22 nucleotides functional RNA molecule (Bartel Citation2004; Filipowicz et al. Citation2005; Sontheimer & Carthew Citation2005; Weiland et al. Citation2012) ().
Figure 1. The stem loop of eca-mir-140 (based on the structure as predicted by miRBase (Griffiths-Jones et al. Citation2006) and its sequence identified by Buechli et al. (Citation2013) with two mature miR sequences colored in red and blue.
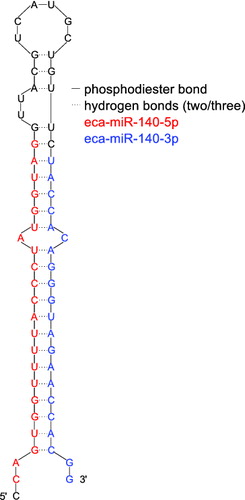
2.2. Function
A growing body of exciting evidence suggests that miRNAs are important regulators of cell differentiation, proliferation/growth, mobility and apoptosis (Zhang Citation2008). A single miRNA can target hundreds of mRNAs and this mechanism is physiologically involved in virtually all biologic processes. On the other hand, aberrant miRNA expression is involved in the initiation of many diseases, including cancer. Nonetheless, the initial categorization of miRNAs as oncogenes or tumor-suppressor genes based on their levels of expression in tumors versus normal tissues has proven to be inaccurate, as experiments have shown that many function dually as both oncogenes and tumor-suppressor genes depending on the cancer type and cellular context. In line with these findings, it seems that the role of a given miRNA is dependent on cancer type and target specificity and, therefore, it is important to elucidate the role of aberrantly expressed miRNAs in each type of cancer (Saunders et al. Citation2007; Cortez et al. Citation2011). MiRNAs play important roles in the regulation of target genes by binding to complementary regions of messenger transcripts to repress their translation or regulate degradation (Bartel Citation2004; Filipowicz et al. Citation2005; Sontheimer & Carthew Citation2005). Early studies in the worm Caenorhabditis elegans and recent studies in mammalian cell cultures suggest that prevention of protein translation can be achieved by (1) deadenylation of the poly(A) tail of the mRNA, (2) competition of the argonaute protein-RNA-induced silencing complex with translation initiation factors and cap structure, (3) blocking translation elongation, (4) promoting premature dissociation of ribosomes, and (5) degradation of the nascent polypeptide chain (Eulalio et al. Citation2008; Bhatt et al. Citation2011).
Recently, numerous studies have documented the importance of miRNAs as an essential cornerstone of the genetic system. Although RNA is usually considered an unstable molecule because of the ubiquitous ribonucleases, miRNAs in contrast have been shown to circulate in the bloodstream and other body fluids in a stable, cell-free form. Importantly, extracellular miRNAs can be aberrantly present in plasma, serum, and other body fluids during the pathogenesis of many disorders and, thus, are promising noninvasive disease biomarkers. However, the origin and biological function of extracellular miRNA remains incompletely understood (Liang et al. Citation2014). Furthermore, dysregulated miRNA expression in cancer cells and damaged tissues from different disorders implicates a functional role of miRNA in disease development (Weiland et al. Citation2012). Along with the cancer-associated genomic regions (CAGRs) harboring miRNA genes and epigenetic changes, several studies have indicated that single nucleotide polymorphisms (SNPs) in both miRNA genes and miRNA-target genes also increase the predisposition to specific types of cancers. Although SNPs are comparatively rare in miRNA genes, they can affect miRNA function in pri-miRNA transcription, pri-miRNA and pre-miRNA processing, and miRNA binding sites (Cortez et al. Citation2011). Moreover, some SNPs located outside miRNA genes can affect miRNA expression (miRNA expression quantitative trait loci [miRNA eQTLs]) and therefore contribute to disease pathogenesis (Gamazon et al. Citation2012).
2.3. Biogenesis and maturation
MiRNAs are transcribed in the nucleus as pri-miRNA and then processed by Drosha, a Class 2 RNase III enzyme, into pre-miRNA via the miRNA or canonical pathway. Of note, intronic pre-miRNA hairpins of the mirtron pathway (also known as microprocessor-independent or noncanonical pathway) are formed without Drosha processing. Pre-miRNA molecules are transported from the nucleus to the cytoplasm by exportin 5. Upon entering the cytoplasm, they are recognized by Dicer. Dicer modulates pre-miRNA and generates double-stranded-RNAs called miRNA/miRNA*, which are recognized by the RNA-induced silencing complex (RISC) and converted into the separate (mature) guide strand miRNA and the passenger strand (miRNA*) molecules by argonaute proteins (Ago) unwinding it. The passenger strand is usually degradated. The RISC carries the mature miRNA molecule to complementary miRNA target sites within the mRNA molecule, where it affects gene expression by miRNA:mRNA sequence complementarity. A consequence of perfect complementarity between miRNA:mRNA molecules is mRNA cleavage and degradation. Imperfect alignment represses gene translation. The mature miRNA interacts with the three prime untranslated region (3′-UTR) of the target mRNA although targeting can also occur in 5′-UTR and open reading frame (ORF). However, endogenous ORF targeting seems to be less frequent and effective than 3′-UTR targeting but still more frequent than 5′-UTR targeting (Matranga et al. Citation2005; Rand et al. Citation2005; Berezikov et al. Citation2007; Winter et al. Citation2009; Bartel Citation2009; Cortez et al. Citation2011; Bronze-da-Rocha Citation2014). A third pathway for the production of miRNAs, the Dicer-independent pathway, was recently identified in zebrafish and mammals (Huang & Lin Citation2012).
Importantly, exosomes represent a newly discovered mechanism by which donor cells can communicate and influence the gene expression of recipient cells (Cortez et al. Citation2011). Exosomes are vesicles of endocytic origin released by many cells. These vesicles can mediate communication between cells by facilitating processes such as antigen presentation. It has been shown that exosomes contain both mRNA and miRNA, which can be delivered to another cell, and can be functional in this new location. This RNA is called ‘exosomal shuttle RNA’ (esRNA) (Valadi et al. Citation2007).
2.4. Nomenclature
Annotating mammalian genomes for ncRNAs is nontrivial since far from all ncRNAs are known and the computational models are resource demanding. Currently, the human genome holds the best mammalian ncRNA annotation, a result of numerous efforts by several groups (Anthon et al. Citation2014). In general, miRNAs are assigned sequential numerical identifiers. The database uses abbreviated 3 or 4 letter prefixes to designate the species, such that identifiers take the form hsa-miR-101 (in Homo sapiens). The mature sequences are designated ‘miR’ in the database, whereas the precursor hairpins are labelled ‘mir’. The gene names are intended to convey limited information about functional relationships between mature miRNAs. For example, hsa-miR-101 in human and mmu-miR-101 in mouse are orthologous. Paralogous sequences whose mature miRNAs differ at only one or two positions are given lettered suffixes. For example, mmu-miR-10a and mmu-miR-10b in the mouse. Distinct hairpin loci that give rise to identical mature miRNAs have numbered suffixes (e.g. dme-mir-281-1 and dme-mir-281-2 in Drosophila melanogaster). It should be noted that plant and viral naming schemes differ subtly (Griffiths-Jones et al. Citation2006). Furthermore, miRNAs with nearly identical sequences except for one or two nucleotides are annotated with an additional lower case letter. For example, miR-123a would be closely related to miR-123b, whereas those of mir-6-1 and mir-6-2 are identical. Two different mature miRNA sequences which were excised from opposite arms of the same hairpin precursor are currently named as miR-17-5p (5′ arm) and miR-17-3p (3′ arm). When relative expression levels are known, an asterisk following the name indicates a miRNA expressed at low levels relative to the miRNA in the opposite arm of a hairpin. For example, miR-123 and miR-123* would share a pre-miRNA hairpin, but more miR-123 would be found. The ‘mir’ is followed by a dash and a number, the latter often indicating order of naming. For example, mir-318 was named and discovered earlier than mir-319. Pre-miRNAs that lead to 100% identical mature miRNAs but that are located at different places in the genome are indicated with an additional dash-number suffix. For example, the pre-miRNAs hsa-mir-194-1 and hsa-mir-194-2 lead to an identical mature miRNA (hsa-miR-194) but are located in different regions of the genome (Ambros et al. Citation2003; Griffiths-Jones et al. Citation2006). The so-called seed region is defined as nucleotides 2–7 from 5′ end of mature miRNA sequences (Gitlin et al. Citation2005).
2.5. The potential role of miRNAs in diagnostics
The discovery of miRNAs as a new class of regulators of gene expression has triggered an explosion of research activities. A number of miRNAs have been found to be present in plasma and other body fluids of humans and mice in surprisingly high concentrations. This observation was unexpected in two respects: first, the fact that these molecules are present at all extracellularly at significant concentrations and second, that these molecules appear to be stable outside of the cell. In light of this it has been suggested that the biological function of miRNAs may also extend outside of the cell and mediate cell-cell communication (Wang et al. Citation2010). In accord, the concept that miRNAs could function as hormones has been advocated (Cortez et al. Citation2011).
The great interest in miRNAs reflects their central role in gene-expression regulation and the implication of miRNA-specific aberrant expression in the pathogenesis of cancer, cardiac, immune-related and other diseases. Another avenue of current research is the study of circulating miRNAs in serum, plasma, and other body fluids. Since deregulated miRNA expression is an early event in tumorigenesis, measuring circulating miRNA levels may also be useful for early cancer detection, which can contribute greatly to the success of treatment (Cortez et al. Citation2011). The noninvasive nature of sampling circulating miRNA, compared to biopsy specimen collection for instance, as well as their sensitivity and specificity as markers of certain diseases have encouraged the pursuit of miRNA biomarker research. As a result, approximately 100 circulating miRNAs have been identified as biomarkers for different disorders, and their number is growing (Chen et al. Citation2008; Chim et al. Citation2008; Cortez et al. Citation2011, Weiland et al. Citation2012). Specifically, variations in the level of miRNA expression have been linked with the development, progression, and spread of cancer to distant organs, indicating that these tiny molecules may play a role in accurate and early diagnosis, and also as prognostic determinants. Furthermore, modulating their activity promises opportunities for developing and designing novel cancer therapeutics. Recent studies indicate miRNA detection in a wide variety of human biologic specimens including fine-needle aspirates, tissues, blood, serum, and other body fluids, such as tears, breast milk, bronchial lavage, colostrum, and seminal, amniotic, pleural, peritoneal, and cerebro-spinal fluids making them clinically useful biomarkers of disease for early detection, prognosis, and for designing personalized therapies (Weber et al. Citation2010; Cortez et al. Citation2011; Sethi et al. Citation2013).
2.6. The potential role of miRNAs as therapeutics
An important avenue for future research is the development of therapies based on miRNAs to influence their effects on gene expression, which, as detailed above, acts at the posttranscriptional level by inhibiting translation or inducing degradation of the target mRNAs (Bronze-da-Rocha Citation2014) and can control several pathways including developmental timing, haematopoiesis, organogenesis, apoptosis, cell proliferation and possibly even tumorigenesis (Kim Citation2005a, Citation2005b). A promising approach is to target disease-related miRNAs using anti-miRNA oligos (miRNA inhibitors) to knock down overexpressed miRNAs either in their mature or precursor form (Zhang Citation2008). A potential advantage of miRNA therapy compared to conventional therapies is that they target multiple genes (Krek et al. Citation2005; Sayed & Abdellatif Citation2011; Bronze-da-Rocha Citation2014) involved in the same pathway and not only a single protein (Caroli et al. Citation2013). MiRNAs can be modulated by oligonucleotides composed of high-affinity nucleotide mimics, designated miR-mimics, and single-stranded antisense oligonucleotides, termed antimiRs or antagomirs (Bronze-da-Rocha Citation2014).
The miR-mimics are used with the aim of increasing a specific miRNA level when there is a downregulation of a miRNA in a certain disease state. Mimics are artificial small nucleotide sequences, similar to pre-miRNAs, which are recognized by the miRNA biogenesis machinery or loaded into the RISC, acting as the endogenous miRNA of interest and blocking gene expression (Galasso et al. Citation2010; Fasanaro et al. Citation2010; Oliveira-Carvalho et al. Citation2013; Caroli et al. Citation2013; Bronze-da-Rocha Citation2014). In contrast, antagomirs are employed when pathological conditions result from the upregulation of a specific miRNA. Antagomirs are modified antisense oligonucleotides (morpholinos) artificially synthesized that thoroughly target the mature miRNA sequence, thereby increasing the amount of mRNA target of that specific miRNA (Weiler et al. Citation2006; Galasso et al. Citation2010; Fasanaro et al. Citation2010; Bronze-da-Rocha Citation2014). The antimiR can exert its function at different levels of miRNA biogenesis by interfering with pri-miRNAs and with the export of pre-miRNA (Caroli et al. Citation2013).
Further possibilities of inhibiting miRNA function are achieved by mechanisms termed sponges, masking and erasers. Sponges prevent the binding of the miRNA to its target. Masking uses oligonucleotides that modulate a specific miRNA target, since they display a perfect complementarity to the target miR. Erasers are also oligonucleotides with tandem repeats of a specific sequence of the complementary antisense sequence of the miRNA (Galasso et al. Citation2010; Fasanaro et al. Citation2010; Oliveira-Carvalho et al. Citation2013; Caroli et al. Citation2013; Bronze-da-Rocha Citation2014).
2.7. Sampling and assessment of miRNAs
It has been demonstrated that miRNAs were present in serum and plasma of humans and other animals such as mice, rats, bovine fetuses, calves, and horses (Chen et al. Citation2008). The levels of miRNAs in serum turned out to be stable, reproducible, and consistent among individuals of the same species (Chen et al. Citation2008).
Principally, there are three methods for identifying miRNAs: the cloning approach, next-generation sequencing approach and computational approach. At present, the computational approach (or so called in silico) can be divided into three types: ab initio prediction based on sequence and structure features, comparative genomic approach based on evolutionary conservation and the integrated approach (Zhou et al. Citation2009). Available miRNA databases, e.g. miRBase, help researchers to look for sequence, secondary structure, and putative targets of miRNA annotated in more than 200 genomes (Griffiths-Jones et al. Citation2006). However, in silico predictions of mRNA:miRNA interactions do not take into account the specific transcriptomic status of the biological system and are biased by false positives (Meyer et al. Citation2014). RNA quality control is a crucial step in guaranteeing integer nondegraded RNA and receiving meaningful results in gene expression profiling experiments, using microarray, Reverse-Transcription quantitative Polymerase Chain Reaction (RT-qPCR), or Next-Generation-Sequencing (NGS) by RNA-Seq or small-RNA Seq. Therefore, assessment of RNA integrity and purity is very essential prior to gene expression analysis of sample RNA to ensure the accuracy of any downstream applications (Kirchner et al. Citation2014). The most common strategy to evaluate the RNA quality is the assesment of RNA integrity number (RIN) that is partially based on the characteristics of ribsomal RNA (rRNA)(Schroeder et al. Citation2006). Whereas the quality of miRNA derived from a sample can be assesed by the measurement of total RNA, the miRNA derived from biofluids is not trivial, as the presence of rRNA is rather limited to cells. Several techniques are currently available for establishing miRNA signatures or profiles in body fluids, such as miRNA microarrays (Lodes et al. Citation2009), quantitative real-time PCR (qRT-PCR) (Lawrie et al. Citation2008; Cortez et al. Citation2011), and deep sequencing (NGS) (Chen et al. Citation2008). Of note, for PCR based-techniques is that heparinized blood contains an inhibitor of Taq polymerase (Peltier & Latham Citation2008). However, increasing next-generation sequencing is becoming the gold-standard method for assessment of miRNA signatures.
The diagnostic and prognostic potential of miRNAs thus relies partly on their high stability and resistance to storage handling. It has been consistently shown that serum miRNAs remain stable after being subjected to severe conditions that would normally degrade most RNAs, such as boiling, very low or high pH levels, extended storage, and 10 freeze–thaw cycles (Chen et al. Citation2008). In addition, recent studies have demonstrated that miRNAs are preserved in archived 10-year-old human serum samples (Zhu et al. Citation2009), and in unrefrigerated dried serum blots, which may be a more convenient and safer way to save, transport, and store serum and other body fluids, such as saliva and urine, for miRNA assays (Patnaik et al. Citation2010).
This stability can be partially explained by the discovery of lipoprotein complexes, including small membrane vesicles of endocytic origin called exosomes or microvesicles (30–100 nm), containing miRNAs (Valadi et al. Citation2007), mRNAs (El-Hefnawy et al. Citation2004; Smalheiser Citation2007), and proteins (Théry et al. Citation2002; Cortez et al. Citation2011). Although the presence of miRNAs in exosomes or high-density lipoproteins (HDL) could explain their stability in serum, other possibilities include protection by chemical modifications or association with protein complexes. In this regard, recent findings showed that the RNA-binding protein nucleophosmin 1 (NPM1) may have a role in the exportation, packaging, and protection of extracellular miRNAs (Wang et al. Citation2010). Furthermore, recent studies demonstrated that potentially 90% of the plasma and serum miRNAs are not encapsulated by vesicles, but cofractionated with protein complexes (Cortez et al. Citation2011).
3. Equine miRNAs and their potential role in equine medicine
A total of 407 novel equine miRNA genes including 354 mature miRNAs have been identified based on an improved integrated computational approach using previously reported algorithms. Of these, 75 miRNAs were grouped into 32 families based on seed sequence identity. MiRNA genes tend to be present as clusters in some chromosomes, and 146 miRNA genes accounted for 36% of the total were observed as part of polycistronic transcripts referring to each encoding several different polypeptides (Zhou et al. Citation2009). In addition, 329 novel miRNAs in normal Thoroughbred horse tissues including skeletal muscle, colon and liver were identified based on next-generation sequencing technology as well as a total of 201 families including 292 known miRNAs. Distinct subsets of miRNAs were differentially expressed in a tissue-specific manner: 36 miRNAs in muscle samples, 99 miRNAs in colon samples, and 31 miRNAs in liver samples. The miRNA genes were distributed across all the chromosomes except chromosomes 29 and 31 in the horse reference genome (Kim et al. Citation2014). These two chromosomes have one of the smallest numbers of genes annotated in horse reference genome [http://www.ensembl.org] (Flicek et al. Citation2014). In some chromosomes, multiple miRNAs were clustered and considered to be polycistronic transcripts. A base composition analysis showed that equine miRNAs had a higher frequency of A+U than G+C. Furthermore, U tended to be more frequent at the 5' end of miRNA sequences (Kim et al. Citation2014).
3.1. Evolutionary conservation
Phylogenetic analysis of miRNA precursors indicated that 85% of human disease pre-miRNAs were highly conserved in animals, showing less than 5% nucleotide substitution rates over evolutionary time. As an example, conservation of (human) hsa-miR-143-3p associated with type 2 diabetes has been demonstrated and targets AKT1 gene which is highly conserved in pig, horse and dog. Functional analysis of AKT1 gene using gene ontology showed that it is involved in glucose homeostasis, positive regulation of glucose import, positive regulation of glycogen biosynthetic process, glucose transport and response to food (Buza et al. Citation2014). Furthermore, multiple sequence alignments of the gene cluster encoding miR-433/127 in mammalian species showed that the precursors of miR-433 and of miR-127 exhibited 95% and 100% similarity, respectively, in human, chimpanzee, horse, dog, monkey, rat, cow, and mouse indicating a conserved gene structure and transcriptional regulation of miR-433 and miR-127 in mammals (Song & Wang Citation2009). Of note, regarding miR-675-5p/3p the canonical miRNA was more conserved than the passenger strand (Guo et al. Citation2014).
Non-conserved miRNAs also seem to play roles in diseases, e.g. human miR-95 was shown to affect autophagy in lysosomal storage disorder (Frankel et al. Citation2014).
Multiple sequence analysis indicated that over 60% of known horse mature miRNAs were perfectly matched to human disease-associated miRNAs, followed by dog (50%) (Buza et al. Citation2014). In addition, sequencing the cDNA of the equine ACTN3 gene, which is overexpressed in skeletal muscles with fast fibers of type IIb, revealed 8 SNPs, 6 in the coding and 2 in the 3' non-coding regions with no amino acid change and not affecting potential miRNA targets. The equine in silico promoter sequence revealed a structure with two regions similar to those of other mammalian species (Mata et al. Citation2012).
3.2. Emerging understanding of equine miRNAs in physiological and pathological states
3.2.1. Breeding
Polymorphisms within miRNA genes present a source of novel biomarkers for phenotypic traits in animal breeding. The analysis of 16 genomes including four livestock species: pig, horse, cattle and chicken revealed 65 polymorphisms located within mature miRNA regions in these four species, including 28% within the seed region in cattle and chicken. As a result, the analysis of polymorphic miRNA genes and prioritization of potential regulatory polymorphisms can serve the animal science community to efficiently select miRNA SNPs and/or miRNA eQTLs for further quantitative and molecular genetic evaluations of their phenotypic effects and causal associations with livestock production traits (Jevsinek Skok et al. Citation2013).
3.2.2. Fertility
Proper cell communication within the ovarian follicle is critical for the growth and maturation of a healthy oocyte that can be fertilized and develop into an embryo. Cell communication within the follicle involves many signaling molecules and is affected by maternal age. Recent studies indicate that cell communication can be mediated through secretion and uptake of small membrane-enclosed vesicles. The presence of vesicles resembling microvesicles and exosomes containing miRNAs has been shown in equine ovarian follicular fluid. Furthermore, miRNAs in microvesicle and exosome preparations were also present within surrounding granulosa and cumulus cells indicating cell communication within the equine ovarian follicle via microvesicles and exosomes. Of interest, miRNAs present in exosomes from ovarian follicular fluid varied with the age of the mare, and a number of different miRNAs were detected in young versus old mare follicular fluid (da Silveira et al. Citation2012). Relatively little is known about the physiological roles of miRNAs during follicular development in horses. Relative to ovulatory follicles, anovulatory follicles had higher mean levels of miR-21, miR-23b, miR-378, and miR-202 (Donadeu & Schauer Citation2013). A physiological involvement of miR-21, miR-145, miR-224, miR-378, miR-132, and miR-212 in the regulation of cell survival, steroidogenesis, and differentiation during follicle selection and ovulation in the monovular equine ovary has been suggested (Schauer et al. Citation2013).
Regarding male horses, non-coding polymorphisms within phospholipase C, zeta 1 gene (PLCz1) were identified as conferring stallion fertility and PLCz1 has thus been proposed as candidate locus for male fertility in Hanoverian Warmblood horses (Schrimpf et al. Citation2014).
3.2.3. Osteochondrosis
Equine epiphyseal cartilage and subchondral bone miRNome were defined based on next-generation sequencing, including about 300 new miRNAs. Differentially expressed miRNAs were identified between bone and cartilage from 3 healthy and 3 osteochondrosis Anglo-Arabian foals, as well as after an experimental mechanical load. In cartilage, functional annotation of their predicted targets suggests a role in the maintenance of cartilage integrity through the control of cell cycle and differentiation, energy production and metabolism as well as extracellular matrix structure and dynamics. In bone, miRNA predicited targets were associated with osteoblast and osteoclast differentiation, through the regulation of energy production, vesicle transport and some growth factor signaling pathways (Desjardin et al. Citation2014).
Among these miRNAs, miRNA-140 has been identified as a cartilage-specific miRNA with several targets involved in cartilage development and homeostasis. MiR-140 is highly expressed in normal equine articular cartilage and equine cord blood-derived mesenchymal stromal cells (eCB-MSCs) express significantly higher levels of MiR-140 after 14 days of chondrogenic differentiation. Furthermore, miR-140 expression closely paralleled that of the cartilage-specific transcription factor Sox9, suggesting that miR-140 may be under the transcriptional regulation of Sox9 in these cells. In addition, it has been stated that miR-140 may act, in part, through the regulation of the chemokine (CXC motif) ligand 12 (CXCL12) and a disintegrin and metalloproteinase with thrombosponin motifs 5 (ADAMTS5) (Buechli et al. Citation2013).
3.2.4. Metabolism
Physical exercise induces gene expression changes that trigger glucose metabolism pathways in organisms. Lactate dehydrogenase (LDH) and glycogen synthase 1 (GYS1) in the blood of three retired Thoroughbred racehorses were down-regulated, whereas eca-miR-33a and miR-17 were up-regulated, after exercise. In addition, eca-miR-33a and miR-17 inhibited LDHA and GYS1 expression via binding to the 3′ UTR sequences of each gene indicating that eca-miR-33a and miR-17 play important roles in glucose metabolism pathway in horses (Gim et al. Citation2014).
3.2.5. Myopathy
Because of the central function of miRNAs in the proliferation and differentiation of the myoblasts demonstrated in mouse and man, it is assumed that they could be present in equine muscles and their expression profile may be related to the muscle status. A specific miRNA profile in the gluteus medius muscle was related to both recurrent exertional rhabdomyolysis (RER) and polysaccharide storage myopathy (PSSM). A higher expression of mir-1, 133, 23a, 30b, 195 and 339 was detected in RER and a higher expression of mir-195 in PSSM. The miRNA signature was different between French Trotters and Norman Cobs for mir-181, 188 and 206. The RER miRNA profile was more specific and contrasted than the PSSM profile (Barrey et al. Citation2010).
3.2.6. Recurrent airway obstruction
Recurrent airway obstruction (RAO) in horses is an asthma-like condition that commonly affects horses worldwide (Robinson Citation2001). In asthmatic human patients differences in miRNA expression have been shown in bronchoalveolar lavage fluid exosomes, airway T cells, and serum (Panganiban et al. Citation2012; Eissa Citation2013; Simpson et al. Citation2014). As a consequence, miRNA profiles may reveal new insight in our understanding of the mechanism of equine RAO. As the disease develops in mature horses, identification of early biomarkers, e.g. miRNAs could improve the diagnostics in early stages of the disease, before the symptoms appear. Of note, some therapeutic effect of antimiR-126 antagomir has already been reported in the mouse model of asthma (Mattes et al. Citation2009).
4. Conclusions and outlook
The most important means of identifying diseases before symptoms appear is through the discovery of disease-associated biomarkers. Recently, miRNAs have become highly useful biomarkers of infectious, genetic, metabolic and neoplastic diseases in humans, but they have not been well studied in domestic animals. It is probable that many of the animal homologs of human disease-associated miRNAs may be involved in domestic animal diseases (Buza et al. Citation2014). As outlined above the available information of miRNAs in equine physiologic states and diseases is still very limited. However, the potential and translational impact of miRNAs as prognostic biomarkers and therapeutic targets has stimulated tremendous interest in miRNAs and resulted in research efforts in a broad range of human diseases, including cardiovascular (Kunz et al. Citation2014; Maegdefessel Citation2014), endocrine and metabolic disorders (Price et al. Citation2014), neoplasms (Yin et al. Citation2015; Zhao et al. Citation2015) as well as allergic diseases (Lv et al. Citation2014; Simpson et al. Citation2014; Tay et al. Citation2014; Haj-Salem et al. Citation2015). The consequential substantial gain in knowledge is in contrast to the current lack of information in the equine species. Most of these disorders have not or only sparcely been investigated for miRNA signatures in the horse, but are likely to become emerging fields of miRNomics in equine medicine.
Disclosure statement
No potential conflict of interest was reported by the authors.
Additional information
Funding
References
- Ambros V, Bartel B, Bartel DP, Burge CB, Carrington JC, Chen X, Dreyfuss G, Eddy SR, Griffiths-Jones S, Marshall M, et al. 2003. A uniform system for microRNA annotation. RNA. 9:277–279.
- Anthon C, Tafer H, Havgaard JH, Thomsen B, Hedegaard J, Seemann SE, Pundhir S, Kehr S, Bartschat S, Nielsen M, et al. 2014. Structured RNAs and synteny regions in the pig genome. BMC Genomics. 15:459.
- Barrey E, Bonnamy B, Barrey EJ, Mata X, Chaffaux S, Guerin G. 2010. Muscular microRNA expressions in healthy and myopathic horses suffering from polysaccharide storage myopathy or recurrent exertional rhabdomyolysis. Equine Vet J Suppl. 303–310.
- Bartel DP. 2009. MicroRNAs: target recognition and regulatory functions. Cell. 136:215–233.
- Bartel DP. 2004. MicroRNAs: genomics, biogenesis, mechanism, and function. Cell. 116:281–297.
- Berezikov E, Chung WJ, Willis J, Cuppen E, Lai EC. 2007. Mammalian mirtron genes. Mol Cell. 28:328–336.
- Bhatt K, Mi QS, Dong Z. 2011. microRNAs in kidneys: biogenesis, regulation, and pathophysiological roles. Am J Physiol Renal Physiol. 300:F602–F610.
- Bronze-da-Rocha E. 2014. MicroRNAs expression profiles in cardiovascular diseases. Biomed Res Int. doi:10.1155/2014/985408.
- Buechli ME, Lamarre J, Koch TG. 2013. MicroRNA-140 expression during chondrogenic differentiation of equine cord blood-derived mesenchymal stromal cells. Stem Cells Dev. 22:1288–1296.
- Buza T, Arick M 2nd, Wang H, Peterson DG. 2014. Computational prediction of disease microRNAs in domestic animals. BMC Res Notes. 7:403.
- Caroli A, Cardillo MT, Galea R, Biasucci LM. 2013. Potential therapeutic role of microRNAs in ischemic heart disease. J Cardiol. 61:315–320.
- Chen X, Ba Y, Ma L, Cai X, Yin Y, Wang K, Guo J, Zhang Y, Chen J, Guo X, et al. 2008. Characterization of microRNAs in serum: a novel class of biomarkers for diagnosis of cancer and other diseases. Cell Res. 18:997–1006.
- Chim SS, Shing TK, Hung EC, Leung TY, Lau TK, Chiu RW, Lo YM. 2008. Detection and characterization of placental microRNAs in maternal plasma. Clin Chem. 54(3):482–490.
- Cortez MA, Bueso-Ramos C, Ferdin J, Lopez-Berestein G, Sood AK, Calin GA. 2011. MicroRNAs in body fluids–the mix of hormones and biomarkers. Nat Rev Clin Oncol. 8:467–477.
- Cullen BR. 2004. Derivation and function of small interfering RNAs and microRNAs. Virus Res. 102:3–9.
- Desjardin C, Vaiman A, Mata X, Legendre R, Laubier J, Kennedy SP, Laloe D, Barrey E, Jacques C, Cribiu EP, Schibler L. 2014. Next-generation sequencing identifies equine cartilage and subchondral bone miRNAs and suggests their involvement in osteochondrosis physiopathology. BMC Genomics. 15:798.
- Donadeu FX, Schauer SN. 2013. Differential miRNA expression between equine ovulatory and anovulatory follicles. Domest Anim Endocrinol. 45:122–125.
- Eissa NT. 2013. The exosome in lung diseases: message in a bottle. J Allergy Clin Immunol. 131:904–905.
- El-Hefnawy T, Raja S, Kelly L, Bigbee WL, Kirkwood JM, Luketich JD, Godfrey TE. 2004. Characterization of amplifiable, circulating RNA in plasma and its potential as a tool for cancer diagnostics. Clin Chem. 50:564–573.
- Erdmann VA, Barciszewska MZ, Hochberg A, de Groot N, Barciszewski J. 2001. Regulatory RNAs. Cell Mol Life Sci. 58:960–977.
- Eulalio A, Huntzinger E, Izaurralde E. 2008. Getting to the root of miRNA-mediated gene silencing. Cell. 132:9–14.
- Fasanaro P, Greco S, Ivan M, Capogrossi MC, Martelli F. 2010. microRNA: emerging therapeutic targets in acute ischemic diseases. Pharmacol Ther. 125:92–104.
- Filipowicz W, Jaskiewicz L, Kolb FA, Pillai RS. 2005. Post-transcriptional gene silencing by siRNAs and miRNAs. Curr Opin Struct Biol. 15:331–341.
- Flicek P, Amode MR, Barrell D, Beal K, Billis K, Brent S, Carvalho-Silva D, Clapham P, Coates G, Fitzgerald S, et al. 2014. Ensembl 2014. Nucleic Acids Res. 42:D749–D755.
- Frankel LB, Di Malta C, Wen J, Eskelinen EL, Ballabio A, Lund AH. 2014. A non-conserved miRNA regulates lysosomal function and impacts on a human lysosomal storage disorder. Nat Commun. 5:5840.
- Galasso M, Sana ME, Volinia S. 2010. Non-coding RNAs: a key to future personalized molecular therapy? Genome Med. 2:12.
- Gamazon ER, Ziliak D, Im HK, LaCroix B, Park DS, Cox NJ, Huang RS. 2012. Genetic architecture of microRNA expression: implications for the transcriptome and complex traits. Am J Hum Genet. 90:1046–1063.
- Gim JA, Ayarpadikannan S, Eo J, Kwon YJ, Choi Y, Lee HK, Park KD, Yang YM, Cho BW, Kim HS. 2014. Transcriptional expression changes of glucose metabolism genes after exercise in thoroughbred horses. Gene. 547:152–158.
- Gitlin L, Stone JK, Andino R. 2005. Poliovirus escape from RNA interference: short interfering RNA-target recognition and implications for therapeutic approaches. J Virol. 79:1027–1035.
- Griffiths-Jones S, Grocock RJ, van Dongen S, Bateman A, Enright AJ. 2006. miRBase: microRNA sequences, targets and gene nomenclature. Nucleic Acids Res. 34:D140–D144.
- Guo L, Zhao Y, Yang S, Zhang H, Wu Q, Chen F. 2014. An integrated evolutionary analysis of miRNA-lncRNA in mammals. Mol Biol Rep. 41:201–207.
- Haj-Salem I, Fakhfakh R, Bérubé JC, Jacques E, Plante S, Simard MJ, Bossé Y, Chakir J. 2015. MicroRNA-19a enhances proliferation of bronchial epithelial cells by targeting TGFβR2 gene in severe asthma. Allergy. 70:212–219.
- Huang XA, Lin H. 2012. The miRNA Regulation of Stem Cells. Wiley Interdiscip Rev Membr Transp Signal. 1:83–95.
- Jevsinek Skok D, Godnic I, Zorc M, Horvat S, Dovc P, Kovac M, Kunej T. 2013. Genome-wide in silico screening for microRNA genetic variability in livestock species. Anim Genet. 44:669–677.
- Kim MC, Lee SW, Ryu DY, Cui FJ, Bhak J, Kim Y. 2014. Identification and characterization of microRNAs in normal equine tissues by Next Generation Sequencing. PLoS One. 9:e93662.
- Kim VN. 2005a. MicroRNA biogenesis: coordinated cropping and dicing. Nat Rev Mol Cell Biol. 6:376–385.
- Kim VN. 2005b. Small RNAs: classification, biogenesis, and function. Mol Cells. 19:1–15.
- Kirchner B, Paul V, Riedmaier I, Pfaffl MW. 2014. mRNA and microRNA purity and integrity: the key to success in expression profiling. Methods Mol Biol. 1160:43–53.
- Krek A, Grün D, Poy MN, Wolf R, Rosenberg L, Epstein EJ, MacMenamin P, da Piedade I, Gunsalus KC, Stoffel M, Rajewsky N. 2005. Combinatorial microRNA target predictions. Nat Genet. 37:495–500.
- Kunz M, Xiao K, Liang C, Viereck J, Pachel C, Frantz S, Thum T, Dandekar T. 2014. Bioinformatics of cardiovascular miRNA biology. J Mol Cell Cardiol. doi:10.1016/j.yjmcc.2014.11.027.
- Lawrie CH, Gal S, Dunlop HM, Pushkaran B, Liggins AP, Pulford K, Banham AH, Pezzella F, Boultwood J, Wainscoat JS, et al. 2008. Detection of elevated levels of tumour-associated microRNAs in serum of patients with diffuse large B-cell lymphoma. Br J Haematol. 141:672–675.
- Liang H, Gong F, Zhang S, Zhang CY, Zen K, Chen X. 2014. The origin, function, and diagnostic potential of extracellular microRNAs in human body fluids. Wiley Interdiscip Rev RNA. 5:285–300.
- Lodes MJ, Caraballo M, Suciu D, Munro S, Kumar A, Anderson B. 2009. Detection of cancer with serum miRNAs on an oligonucleotide microarray. PLoS One. 4:e6229.
- Lv Y, Qi R, Xu J, Di Z, Zheng H, Huo W, Zhang L, Chen H, Gao X. 2014. Profiling of serum and urinary MicroRNAs in children with atopic dermatitis. PLoS One. 9:e115448.
- Maegdefessel L. 2014. The emerging role of microRNAs in cardiovascular disease. J Intern Med. 276:633–44.
- Mata X, Vaiman A, Ducasse A, Diribarne M, Schibler L, Guérin G. 2012. Genomic structure, polymorphism and expression of the horse alpha-actinin-3 gene. Gene. 491:20–24.
- Matranga C, Tomari Y, Shin C, Bartel DP, Zamore PD. 2005. Passenger-strand cleavage facilitates assembly of siRNA into Ago2-containing RNAi enzyme complexes. Cell. 123:607–620.
- Mattes J, Collison A, Plank M, Phipps S, Foster PS. 2009. Antagonism of microRNA-126 suppresses the effector function of TH2 cells and the development of allergic airways disease. Proc Natl Acad Sci U S A. 106:18704–18709.
- Mattick JS, Makunin IV. 2006. Non-coding RNA. Review. Hum Mol Genet. 15(1):R17–R29.
- Meyer SU, Stoecker K, Sass S, Theis FJ, Pfaffl MW. 2014. Posttranscriptional regulatory networks: from expression profiling to integrative analysis of mRNA and microRNA data. Methods Mol Biol. 1160:165–188.
- Nguyen TT, Brenu EW, Staines DR, Marshall-Gradisnik SM. 2014. MicroRNAs in the Intracellular Space, Regulation of Organelle Specific Pathways in Health and Disease. Microrna. 3(2):98–107.
- Oliveira-Carvalho V, da Silva MM, Guimarães GV, Bacal F, Bocchi EA. 2013. MicroRNAs: new players in heart failure. Mol Biol Rep. 40:2663–2670.
- Panganiban RPL, Pinkerton MH, Maru SY, Jefferson SJ, Roff AN, Ishmael FT. 2012. Differential microRNA epression in asthma and the role of miR-1248 in regulation of IL-5. Am J Clin Exp Immunol. 1:154–165.
- Patnaik SK, Mallick R, Yendamuri S. 2010. Detection of microRNAs in dried serum blots. Anal Biochem. 407:147–149.
- Peltier HJ, Latham GJ. 2008. Normalization of microRNA expression levels in quantitative RT-PCR assays: identification of suitable reference RNA targets in normal and cancerous human solid tissues. RNA. 14:844–852.
- Price NL, Ramírez CM, Fernández-Hernando C. 2014. Relevance of microRNA in metabolic diseases. Crit Rev Clin Lab Sci. 51:305–320.
- Rand TA, Petersen S, Du F, Wang X. 2005. Argonaute2 cleaves the anti-guide strand of siRNA during RISC activation. Cell. 123:621–629.
- Robinson NE. 2001. International Workshop on Equine Chronic Airway Disease. Michigan State University 16-18 June 2000. Equine Vet J. 33:5–19.
- Saunders MA, Liang H, Li WH. 2007. Human polymorphism at microRNAs and microRNA target sites. Proc Natl Acad Sci U S A. 104:3300–3305.
- Sayed D, Abdellatif M. 2011. MicroRNAs in development and disease. Physiol Rev. 91:827–887.
- Schauer SN, Sontakke SD, Watson ED, Esteves CL, Donadeu FX. 2013. Involvement of miRNAs in equine follicle development. Reproduction. 146:273–282.
- Schrimpf R, Dierks C, Martinsson G, Sieme H, Distl O. 2014. Genome-wide association study identifies phospholipase C zeta 1 (PLCz1) as a stallion fertility locus in Hanoverian warmblood horses. PLoS One. 9:e109675.
- Schroeder A, Mueller O, Stocker S, Salowsky R, Leiber M, Gassmann M, Lightfoot S, Menzel W, Granzow M, Ragg T. 2006. The RIN: an RNA integrity number for assigning integrity values to RNA measurements. BMC Mol Biol. 7:3.
- Sethi S, Ali S, Kong D, Philip PA, Sarkar FH. 2013. Clinical implication of microRNAs in molecular pathology. Clin Lab Med. 33:773–786.
- Silveira JC da, Veeramachaneni DN, Winger QA, Carnevale EM, Bouma GJ. 2012. Cell-secreted vesicles in equine ovarian follicular fluid contain miRNAs and proteins: a possible new form of cell communication within the ovarian follicle. Biol Reprod. 86:71.
- Simpson LJ, Patel S, Bhakta NR, Choy DF, Brightbill HD, Ren X, Wang Y, Pua HH, Baumjohann D, Montoya MM, et al. 2014. A microRNA upregulated in asthma airway T cells promotes TH2 cytokine production. Nat Immunol. 15:1162–1170.
- Smalheiser NR. 2007. Exosomal transfer of proteins and RNAs at synapses in the nervous system. Biol Direct. 2:35.
- Song G, Wang L. 2009. A conserved gene structure and expression regulation of miR-433 and miR-127 in mammals. PLoS One. 4:e7829.
- Sontheimer EJ, Carthew RW. 2005. Silence from within: endogenous siRNAs and miRNAs. Cell. 122:9–12.
- Szymański M, Barciszewska MZ, Zywicki M, Barciszewski J. 2003. Noncoding RNA transcripts. J Appl Genet. 44:1–19.
- Tan Z, Zhang W, Shi Y, Wang F. 2015. RNA folding: structure prediction, folding kinetics and ion electrostatics. Adv Exp Med Biol. 827:143–183.
- Tay HL, Plank M, Collison A, Mattes J, Kumar RK, Foster PS. 2014. MicroRNA: potential biomarkers and therapeutic targets for allergic asthma? Ann Med. 46:633–639.
- Théry C, Zitvogel L, Amigorena S. 2002. Exosomes: composition, biogenesis and function. Nat Rev Immunol. 2:569–579.
- Valadi H, Ekström K, Bossios A, Sjöstrand M, Lee JJ, Lötvall JO. 2007. Exosome-mediated transfer of mRNAs and microRNAs is a novel mechanism of genetic exchange between cells. Nat Cell Biol. 9:654–659.
- Wang K, Zhang S, Weber J, Baxter D, Galas DJ. 2010. Export of microRNAs and microRNA-protective protein by mammalian cells. Nucleic Acids Res. 38:7248–7259.
- Weber JA, Baxter DH, Zhang S, Huang DY, Huang KH, Lee MJ, Galas DJ, Wang K. 2010. The microRNA spectrum in 12 body fluids. Clin Chem. 56:1733–1741.
- Weiland M, Gao XH, Zhou L, Mi QS. 2012. Small RNAs have a large impact: circulating microRNAs as biomarkers for human diseases. RNA Biol. 9:850–859.
- Weiler J, Hunziker J, Hall J. 2006. Anti-miRNA oligonucleotides (AMOs): ammunition to target miRNAs implicated in human disease? Gene Ther. 13:496–502.
- Winter J, Jung S, Keller S, Gregory RI, Diederichs S. 2009. Many roads to maturity: microRNA biogenesis pathways and their regulation. Nat Cell Biol. 11:228–234.
- Yin J, Hou P, Wu Z, Wang T, Nie Y. 2015. Circulating miR-375 and miR-199a-3p as potential biomarkers for the diagnosis of hepatocellular carcinoma. Tumour Biol. [Epub ahead of print].
- Zhang C. 2008. MicroRNomics: a newly emerging approach for disease biology. Physiol Genomics. 33:139–147.
- Zhao Q, Ping LI, Junrong MA, Xijie YU. 2015. MicroRNAs in lung cancer and lung cancer bone metastases: biomarkers for early diagnosis and targets for treatment. Recent Pat Anticancer Drug Discov. [Epub ahead of print]
- Zhou M, Wang Q, Sun J, Li X, Xu L, Yang H, Shi H, Ning S, Chen L, Li Y, et al. 2009. In silico detection and characteristics of novel microRNA genes in the Equus caballus genome using an integrated ab initio and comparative genomic approach. Genomics. 94:125–131.
- Zhu W, Qin W, Atasoy U, Sauter ER. 2009. Circulating microRNAs in breast cancer and healthy subjects. BMC Res Notes. 2:89.