Abstract
A holistic perspective on changing rainfall-driven flood risk is provided for the late 20th and early 21st centuries. Economic losses from floods have greatly increased, principally driven by the expanding exposure of assets at risk. It has not been possible to attribute rain-generated peak streamflow trends to anthropogenic climate change over the past several decades. Projected increases in the frequency and intensity of heavy rainfall, based on climate models, should contribute to increases in precipitation-generated local flooding (e.g. flash flooding and urban flooding). This article assesses the literature included in the IPCC SREX report and new literature published since, and includes an assessment of changes in flood risk in seven of the regions considered in the recent IPCC SREX report—Africa, Asia, Central and South America, Europe, North America, Oceania and Polar regions. Also considering newer publications, this article is consistent with the recent IPCC SREX assessment finding that the impacts of climate change on flood characteristics are highly sensitive to the detailed nature of those changes and that presently we have only low confidence1 in numerical projections of changes in flood magnitude or frequency resulting from climate change.
Editor D. Koutsoyiannis
Citation Kundzewicz, Z.W., et al., 2013. Flood risk and climate change: global and regional perspectives. Hydrological Sciences Journal, 59 (1), 1–28.
Résumé
Cet article trace une perspective globale de l’évolution des risques d’inondation d’origine pluviale pour la fin du 20ème et le début du 21ème siècle. Les pertes économiques dues aux inondations ont fortement augmenté, principalement en raison de l’exposition croissante des actifs à risque. Il n’a pas été possible d’attribuer les tendances de débits de pointe au changement climatique d’origine anthropique au cours des dernières décennies. Les augmentations prévues de la fréquence et de l’intensité des précipitations extrêmes, basées sur des modèles climatiques, devraient contribuer à une augmentation des inondations locales (par exemple, des crues éclairs et des inondations en milieu urbain) provoquées par les pluies. Nous avons évalué la littérature incluse dans le rapport SREX du GIEC et celle qui a été publiée depuis, afin d’estimer l’évolution des risques d’inondation dans les sept régions considérées dans le rapport récent du SREX du GIEC, à savoir l’Afrique, l’Asie, l’Amérique centrale et du Sud, l’Europe, l’Amérique du Nord, l’Océanie et les régions polaires. Tenant compte des publications les plus récentes, le présent article rejoint la récente évaluation SREX du GIEC selon laquelle les impacts du changement climatique sur les caractéristiques des crues sont très sensibles aux détails de ces changements, et qu’à l’heure actuelle nous ne pouvons avoir qu’une confiance limitée dans les projections numériques de l’évolution de l’amplitude ou de la fréquence des inondations résultant du changement climatique.
1 INTRODUCTION
The Special Report on “Managing the Risks of Extreme Events and Disasters to Advance Climate Change Adaptation” of the Intergovernmental Panel on Climate Change (IPCC), here abbreviated to SREX report (Field et al. Citation2012), critically assessed the recent scientific literature on climate change and the impacts from extreme events. The report was the product of a multi-national and multi-disciplinary authorship from Working Groups I and II of the IPCC, assisted by a large pool of academic and government experts involved in the multi-stage review process. A very wide range of information, opinions and hypotheses were assessed and a prioritization of topics established, with respect to their importance, likelihood and confidence. Most authors of the present article were also authors of one of two chapters of the SREX report, namely, Chapter 3, “Changes in climate extremes and their impacts on the natural physical environment” (Seneviratne et al. Citation2012, hereafter referred to as S12), and Chapter 4, “Changes in impacts of climate extremes: human systems and ecosystems” (Handmer et al. Citation2012, hereafter referred to as H12). The present article provides a holistic perspective on the key conclusions of the SREX report concerning changing flood risk, extended, where appropriate, with references from even more recent literature.Footnote1Footnote2 However, it should be noted that, although most authors of the current article were authors of the SREX report, this article is NOT an IPCC report. While we believe our conclusions are congruent with the IPCC report, the views expressed in this article are those of the authors only and do not represent, in any way, an IPCC report or supplement. This article also includes complementary information and materials, which supplement and refine the conclusions from the SREX report.
In the glossary of the IPCC SREX report, floods are defined as: “the overflowing of the normal confines of a stream or other body of water or the accumulation of water over areas that are not normally submerged. Floods include river (fluvial) floods, flash floods, urban floods, pluvial floods, sewer floods, coastal floods, and glacial lake outburst floods.” These various classes of floods are generated by different mechanisms. The present article does not address coastal flooding caused by storm surges.
As noted by Bates et al. (Citation2008), floods are affected by various characteristics of the climatic system, most notably precipitation (intensity, duration, amount, timing, phase—rain or snow), but also temperature patterns (responsible for such phenomena as soil freezing, snow and ice melt and ice jam formation). Floods are also affected by drainage basin conditions, such as pre-existing water levels in rivers, the snow and ice cover, the soil character and status (permeability, soil moisture content and its vertical distribution), the rate of urbanization, and the presence of dikes, dams and reservoirs. Close to sea level, river flooding may be concurrent with storm surge or extreme tide events (Brakenridge et al. Citation2013).
Intense and long-lasting rain is the most common cause of river (fluvial) floods in large river basins, but high-latitude regions are subject to snowmelt floods (sometimes enhanced by rain or ice jams). Floods in small basins may be generated by short-duration, highly intense rainfall. The relationship between the amount of rainfall and flood response in a catchment can be complex. Antecedent conditions can determine the response to an input of rainfall, while the flood response is often controlled by where in the catchment the rain falls. Other natural factors that may induce floods include landslides, reduced channel conveyance and the sudden failure of inhibiting structures, such as the collapse of landslide dams, ice jams or glaciers blocking glacial lakes. Infrequently, the catastrophic failure of an artificial dam can be the direct cause of intense downstream flooding. Within a flooding event, the extent of inundation can be influenced by dike breaches and the blockage of bridges and culverts by debris (Kundzewicz et al. Citation2012).
While most large floods are rare events, at some sites, extensive inundations, following the definition of flood in the IPCC SREX glossary (Field et al. Citation2012), are “climatological normal” occurrences, for example, occurring every spring during snowmelt (Kundzewicz Citation2012), or during an annual monsoon.
The antecedent conditions of a river basin predetermine flooding potential (e.g. S12). Should storage be limited because groundwater levels are elevated and soil moisture is at maximum capacity, then even moderate amounts of rain can generate a large flood. However, the development of a very dry, crusted soil after a prolonged period without rain, or after a wildfire associated with severe drought conditions, can also rapidly convert rainfall to runoff (because the runoff coefficient is higher), resulting in a flash flood.
Flood-associated damage is a function not only of the depth, speed and persistence of the water, but also of the dissolved and suspended load that the flood waters carry, including when the flood becomes a debris flow. Sediment and solid and liquid material, including raw sewage and a wide range of pollutants, can affect the flooded area, often posing a serious health hazard (Kundzewicz et al. Citation2012).
Floods have been singled out from the SREX report as they cause very large amounts of material damage and casualties worldwide, and may raise the broad interest of many readers of Hydrological Sciences Journal. This issue is very timely and important since, these days, many a large flood is attributed by some to climate change.
2 FLOODS TODAY
Large and damaging floods occur every year. Heavy floods visited Pakistan, India and China in the summer of 2010, Colombia from October to December 2010 and Australia during the austral summer 2010/11. The maximum estimated annual damage caused by river floods in one country was recorded in China in 2010, where a total loss of US$51 billion was reported. In 2010, there were nearly 2000 immediate fatalities from monsoonal flooding in Pakistan (Syvitski and Brakenridge Citation2013).
In 2011, severe floods were reported in Mozambique, Namibia, South Africa and Uganda in Africa; Brazil, Columbia, Mexico and the United States in the Americas; and Cambodia, China, India, Korea, Pakistan, the Philippines and Thailand in Asia, with fatalities in each flood exceeding 50 (over 1000 in the Phillippines and Colombia) and high material damage, in particular in the developed countries from the list above (Kundzewicz et al. Citation2012). In 2012, “killer floods,” inducing more than 50 fatalities each, occurred in Madagascar, Niger and Nigeria in Africa; Bangladesh, China, India, North and South Korea, the Phillippines and Russia in Asia; and Argentina, the United States and Haiti in the Americas.
The international loss databases with global coverage such as EM-DAT, NatCatSERVICE and Sigma (maintained by the Centre for the Epidemiology of Disasters, Munich Re and Swiss Re, respectively, see the following websites: http://www.emdat.be/, https://www.munichre.com/touch/naturalhazards/en/natcatservice/default.aspx, http://www.swissre.com/sigma/) show an increase in reported flood disasters and flood losses through time. However, part of this increase may be attributed to improvements in reporting (Peduzzi et al. Citation2009, Citation2012), population increase and urbanization in flood-prone areas, increase of property value and degraded awareness about natural risks (due to less natural lifestyle). For the period 1980–2011, reported flood losses (adjusted for inflation) have increased from an average of US$7 billion per year in the 1980s to some US$24 billion per year in the period 2001–2011, of which an average of 9% was insured (data from Munich Re, NatCatSERVICE, September 2012). Over this same period, the average number of fatalities has been in thousands per year, with the highest flood mortalities in South and Southeast Asia.
The map in indicates the location of 3713 flood events during 1985–2010 recorded in the public global database maintained by G.R. Brakenridge at the Dartmouth Flood Observatory (http://floodobservatory.colorado.edu). The map illustrates a clear link between flood reporting and concentrations of population. In addition, regions with few reported floods include the great deserts and high mountains of western North America, southern South America, northern Africa, central Asia and Australia. It is not known if areas of Amazonia actually lack large floods or if instead the online-accessible reporting is sparse; the same holds true for portions of Russia.
Fig. 1 Geographic centres of large floods in the Dartmouth Flood Observatory archive. The map illustrates 3713 events over the period 1985–2010.
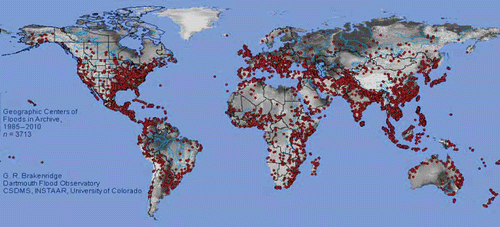
Both the absolute and relative exposures to river flood risk vary considerably between countries ((a) and (b)). In some countries, population and assets are concentrated in flood-prone areas. The highest relative share of population and percentage of economy exposed to floods is found in Cambodia, Bangladesh and Vietnam (Peduzzi et al. Citation2009). Bangladesh is the country with the highest number of people exposed to floods, both in absolute and in relative terms, while it holds ranks four and two in total assets exposed, in absolute and in relative terms, respectively. Approximately 9% of Japan’s land area is flood-prone, but it contains 41% of Japan’s population and 65% of the national assets (IWR Citation2011). Thanks to flood defences, Japan does not show up in , listing countries with highest exposure, except for the total absolute assets (rank 7).
Fig. 2 Exposure to floods: (left) number of people exposed to floods (per year) in terms of absolute numbers and relative proportions; (right) total assets and GDP exposed to floods (per year), absolute and relative. Source: Peduzzi et al. (Citation2009).
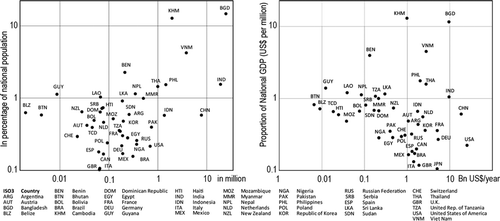
3 ESTIMATING COSTS
The costs imposed by floods on humans, societies and ecosystems can be subdivided into impact or damage costs (or simply losses) and subsequent risk reduction and adaptation costs (e.g. Meyer et al. Citation2013). Conceptually, comparing costs of adaptation with damages before and after adaptation can help in assessing the economic efficiency of adaptation (Parry et al. Citation2009). As assessed by H12, one can attribute costs to the physical, human and socio-environmental impacts of floods—on the lives and health of directly affected persons: all types of tangible and intangible assets, including private dwellings, and agricultural, commercial and industrial stocks and facilities; infrastructure (e.g. transport facilities such as roads, railways, bridges and ports, energy and water supply lines, and telecommunications); public facilities (e.g. hospitals, schools); and natural resources and the environment. Indirect impact costs arise due to the disruption of the flow of goods and services (and therefore economic activity) because of a disaster and are sometimes termed consequential or secondary impacts, as the losses typically flow from the direct impact of a climate event (ECLAC Citation2003, World Bank Citation2010). Indirect damages may be caused by the direct damage to physical infrastructure or sources of livelihoods, or because reconstruction pulls resources away from production. Indirect damages include additional costs incurred because of the need to use alternative and potentially inferior means of production and/or distribution of normal goods and services (Cavallo and Noy Citation2009). Health impacts are typically seen as intangible and may be direct or indirect depending on the circumstances. Increasingly, health impacts are measured through the cost of medical treatment and loss of productive time. This is shifting the impacts from intangible to tangible, although the “market” requirement for tangible may not be satisfied.
Heavy precipitation and field flooding in agriculture delay spring planting, increase soil compaction and cause crop losses through anoxia and root diseases. For ecosystems, flooding can be both a blessing (e.g. an advantageous “flood pulse” bringing an abundance of water and fertile solids) and a curse, leading to erosion and temporary or even permanent loss of habitat (as with the desert rodents studied by Thibault and Brown Citation2008). Before the construction of the High Aswan Dam, Nile floods sustained agriculture in Lower Egypt. Inundations replenished wetlands and aquifers, brought fertile sediments to cultivated areas and enhanced ecosystem services.
Floods are not bad for everyone: flood risks and benefits are distributed very unevenly across societies. The public sector, households and small businesses bear most of the risk, whereas some elements of the private sector benefit from land development in floodplains or reconstruction after floods (see Handmer Citation2008).
Handmer et al. (Citation2012; H12) assessed that direct, tangible impacts are comparatively easy to measure, but costing approaches are not necessarily standardized and assessments are often incomplete, which can make it difficult to aggregate and compare results across the literature. In some countries, flood impact assessment has long been standardized, for example in Britain and parts of the United States. Intangible losses can be estimated using various valuation techniques (see H12 for a review and list of relevant literature). Estimates of impacts that account for tangibles and intangibles are expected to be much larger than those that consider tangible impacts only (Handmer et al. Citation2002, Parry et al. Citation2009).
Typically, excluded impacts include loss of human lives, cultural heritage, ecosystem services and indirect effects (impacts on the flows of trade and finance that make up economies). However, economic activity may simply be displaced. For some countries, informal or undocumented activity can make up more than half a nation’s economy, but this is rarely included in loss estimates. These aspects are very important for developing consistent databases (see Kron et al. Citation2012).
4 CHANGES IN FLOOD RISK OVER TIME
4.1 Changes in heavy precipitation, river flow and floods
The SREX report assessed that it is likely that there have been statistically significant increases in the number of heavy precipitation events in more regions than there have been statistically significant decreases, but also that there are strong regional and sub-regional variations in these trends. This assessment is somewhat weaker than that contained in the previous Fourth assessment report of the IPCC (AR4; Trenberth et al. Citation2007; see also Nicholls and Seneviratne Citation2013). Overall, as highlighted in Alexander et al. (Citation2006) and assessed in the SREX report (S12), observed changes in precipitation extremes have been found to be much less spatially coherent and less statistically significant compared to observed changes in temperature extremes, despite the identification of global or large-scale signals (e.g. Zhang et al. Citation2007). Thus, global-scale changes have been found not to map directly into regional-scale changes, where changes of opposite signal can even be found in the same region.
Regarding the attribution of observed changes in heavy precipitation, the SREX report assessed that there is medium confidence that anthropogenic influence has contributed to changes in extreme precipitation at the global scale, on the basis of, for instance Min et al. (Citation2008, Citation2011). However, the report also noted that the relevant scientific literature is too sparse to attribute changes in extreme precipitation on a seasonal or regional scale (S12).
As highlighted in the SREX report (S12), the influence of anthropogenic climate change has been detected in some variables that contribute to the hydrological cycle in affecting floods, including mean precipitation (Zhang et al. Citation2007), heavy precipitation and snowpack (Barnett et al. Citation2008), though a direct statistical link between anthropogenic climate change and trends in the magnitude/frequency of floods has not been established. In climates where seasonal snow storage and melting play a significant role in annual runoff, the hydrological regime is affected by changes in temperature, and there is abundant evidence for changes in the timing (earlier occurrence) of spring peak flows in snowmelt- and glacier-fed rivers (high confidence). However, not all such areas are experiencing changes in the magnitude of peak flow.
Hence, the SREX report (S12) corroborates Rosenzweig et al. (Citation2007) and Bates et al. (Citation2008) in stating that no gauge-based evidence has been identified for a clear climate-driven, globally widespread, observed change in the magnitude/frequency of river floods during the last decades. There is thus low confidence regarding the magnitude/frequency and even the sign of these changes.
This low confidence directly reflects the limited evidence in many regions. Available instrumental records of floods at stream gauge stations are often sparse in space and short or interrupted in time. Moreover, changes in land use and river engineering further hamper the identification of climate-driven trends. No doubt, long-term records can tell us much about persistence and natural variability. However, despite needing the long-term record for all sorts of reasons, we should not assume that this long-term record is the best predictor of the future. Perhaps the more recent record is. Hence, one should be extremely careful with projecting past trends forward in time.
Even without a change (such as gradual trend), there are extended departures of flood records from long-term average conditions. Among their possible sources are quasi-periodic unforced oscillations of the ocean–atmosphere system. For instance, the probability of flooding in particular regions is related to El Niño or La Niña phases of the ENSO (El Niño Southern Oscillation) cycle (e.g. Wells Citation1990) or the AMO (Atlantic Multi-decadal Oscillation) phase (e.g. Bouwer et al. Citation2008). There is a long-term persistence of flooding that could be viewed in the sense of Hurst (see Koutsoyiannis Citation2011) and Mandelbrot’s Noah effect, referring to the biblical pharaoh’s dream, and clustering of wet and dry years, creating flood-rich and flood-poor episodes. For instance, on the Danube in Vienna, grouping of five of the six largest floods in the 19th century was observed in the last two decades of the century, illustrating pronounced clustering of extreme events (Blöschl and Montanari Citation2010). Climate models do not adequately represent long-term persistence that is observed in real data, and the effect is typically ignored in climate studies (Koutsoyiannis and Montanari Citation2007). The implications are not fully understood or recognized. Acceptance of the long-term persistence hypothesis would lead to a dramatic increase in uncertainty in statistical estimation. This thwarts the trend detection.
Because the length of river gauge record is limited, there is no information about the largest events that occurred in the past, that is beyond the recent experience based on instrumental records, as well as about return periods of extreme floods and their tendency for clustering. Hence, acquiring information on pre-instrumental events is a very welcome way of extending river records. This underpins the importance of palaeohydrology or palaeoflood hydrology, using information encapsulated in geophysical archives and historical hydrology, dealing mainly with documentary evidence about hydrological events (Brázdil and Kundzewicz Citation2006, Brázdil et al. Citation2006). The optimal merging of instrumental, historical and palaeo-information is of considerable importance for understanding flood hazard and significance of multi-decadal climate variability of flood incidence.
4.2 Observed trends in costs of flooding
There is persuasive evidence that the costs of extreme weather events, with flooding as a major contributor, have been exhibiting a significant upward trend (UNISDR Citation2011). The number of reported hydrological events (floods and landslides) worldwide associated with major losses has considerably increased in the last three decades () at a rate greater than the number of reported geophysical events. This difference in the rate between geophysical/seismic events and hydrological events underpins that vulnerability and exposure may not develop in a similar manner over time (Bouwer Citation2011).
Fig. 3 Relative number of hydrological and geophysical events 1980–2012. Base: value of trend lines for absolute numbers in 1980. Source: Munich Re NatCatSERVICE, June 2013.
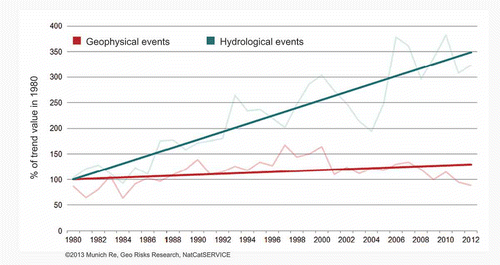
It is acknowledged that reporting on hydro-meteorological disasters has improved significantly because of a denser satellite network, the Internet and international media, whereas earthquakes were recorded globally from terrestrial stations (Peduzzi et al. Citation2012). These improvements have introduced a bias in information access through time, which needs to be addressed in trend analysis (Peduzzi et al. Citation2012). Although many loss records exhibit biases, the record since 1980, presented here in , is more robust for both types of events, as it only contains large events, and events since 1980 have been well documented. However, a portion of this difference may be related to changes in weather patterns and rainfall characteristics. Yet, there are indications that population and assets exposed to floods have increased more rapidly than overall population or economic growth (Bouwer et al. Citation2007, Di Baldassare et al. Citation2010, Bouwer Citation2011, Jongman et al. Citation2012).
The SREX report assessed that there is high confidence, based on high agreement and medium evidence, that economic losses from weather- and climate-related disasters have increased (see references in H12). Increases in total and insured losses from flood disasters are illustrated in . To determine whether trends in such losses can be attributed to climate change, losses over time need to be controlled for changes in the value of what is exposed and its inherent vulnerability. When this is done, normalizing loss records for changes over time in exposure and wealth, most studies using longitudinal disaster loss data attribute the observed increase in losses over time to increasing exposure of people and assets in at-risk areas (Miller et al. Citation2008, Bouwer Citation2011). Social, demographic, economic and political trends may also be important in shaping vulnerability to impacts (Pielke et al. Citation2005, Bouwer et al. Citation2007). There is medium evidence and high agreement that “long-term trends in normalized losses have not been attributed to natural or anthropogenic climate change” (see references in H12).
Fig. 4 Total and insured losses from flood disasters 1980–2012 (in 2012 values). Source: Munich Re NatCatSERVICE, June 2013.
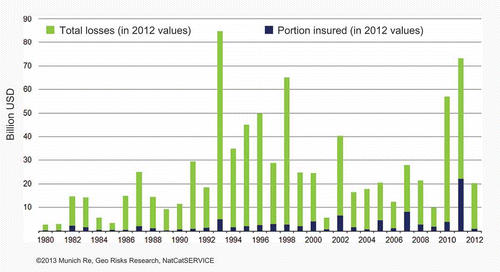
Results from studies of a range of regions did not show a flood signal related to anthropogenic climate change (Pielke and Downton Citation2000, Downton et al. Citation2005, Barredo Citation2009, Hilker et al. Citation2009, Neumayer and Barthel Citation2011, Barredo et al. Citation2012). Although this is the general picture, a few studies observed a partial relationship between recent increases in flood losses and (short-term) changes in intense rainfall events (Fengqing et al. Citation2005, Chang et al. Citation2009, Barthel and Neumayer Citation2012), and some studies suggest an increase in damages related to flash floods and a changing incidence in extreme precipitation (Changnon Citation2001). However, a Swiss study of normalized losses from flash floods and landslides failed to identify any significant trends (Hilker et al. Citation2009).
4.3 Reasons for a perceived increase in flood risk
There are several factors that may explain a perceived increase in flood risk: improved and expanded reporting of disasters, increased exposure of population and assets, and higher frequency and/or intensity of the hazard (Kundzewicz et al. Citation2012). Media play a role—the news coverage is much better, worldwide, than in the past and tends to be focused on the negative side of things (Kundzewicz Citation2011). Some call it a “CNN effect.” Anthropogenic changes in actual flood risk are themselves driven by changes in climatic, hydrological/terrestrial and socio-economic systems (Kundzewicz and Schellnhuber Citation2004). Drivers of changes in flood risk are illustrated, in schematic form, in . Risk reduction activities reduce the hazard and the potential loss (by affecting exposure and vulnerability).
Fig. 5 Anthropogenic drivers of changes in flood risk. Note that risk reduction activities can reduce the hazard and the potential loss (by affecting exposure and vulnerability). After Bouwer (Citation2013), modified.
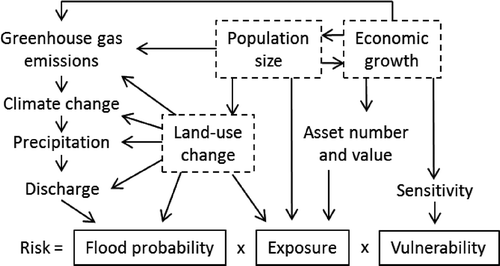
4.3.1 Factors related to the climate system
Among the principal climate-system factors that determine flood risk are the water-containing capacity (and water vapour content) of the atmosphere (Koutsoyiannis Citation2012) and the characteristics of intense precipitation, including its amount and distribution in space and time as affected by large-scale circulation patterns. However, these facts have sometimes created confusion, being presented in an oversimplified manner, while the related processes are very complex. At the land surface, such climate-related variables as evapotranspiration and snowmelt, the phase of precipitation, the sequence of temperature (important for freeze and thaw of snow cover, river or lake ice) and the preconditioning of surface hydrological variables (soil moisture content, groundwater and surface water levels) can all play a significant role.
A change in the climate could physically alter many of the factors affecting floods, for example, precipitation (volume and timing, proportions of precipitation falling as snow and rain), snow cover, antecedent soil moisture content, surface water levels, sea level, glacial lake conditions and vegetation, and thus may change the characteristics of floods.
Seneviratne et al. (Citation2012; S12) assesses that it “is likely that there have been statistically significant increases in the number of heavy precipitation events (e.g. 95th percentile of precipitation totals of all days with precipitation) in more regions than there have been statistically significant decreases, but there are strong regional and subregional variations in the trends.” Even if 95th percentile does not represent an extreme event, taking a higher, say, 99th percentile, we would have to deal with a much smaller sample size; hence, there is a trade-off between the percentile and the sample size.
4.3.2 Factors related to terrestrial/hydrological systems
The characteristics of terrestrial/hydrological systems play a pivotal part in driving flood risk. The most pertinent are catchment size, geology, landscape, topography and soils; the latter are often strongly modified by human intervention. River discharge is an integrated result of processes in the drainage basin—from precipitation to runoff.
Land-use and land-cover changes also affect floods, as do engineering developments such as dikes and reservoirs that regulate flow processes. Alterations in catchment surface characteristics (e.g. land cover), flood-plain storage and the river network can all modify the physical characteristics of river floods. Increased urbanization has led to soil sealing and growth of impermeable surfaces, reducing the accommodation space for flood waters. The reduction of forest and wetland coverage is also reducing the role of these ecosystems in buffering flood events (Bradshaw et al. Citation2007). In urban areas, the value of the runoff coefficient (portion of precipitation that enters a stream) is high, while the water-storage capacity (as in flood plains and wetlands) is low, in contrast to rural (and especially forested) areas. Hence, urban and rural catchments of the same size and topography will react differently to the same amount of precipitation. The peak discharge in the urban area is usually much higher, and the time-to-peak is shorter, than in the rural areas (Kundzewicz et al. Citation2012). Assessment of the multiple drivers of flood risk in Shanghai (Wu et al. Citation2012) showed that rapid urban expansion, infilling of natural drainage networks, changes in precipitation intensities and runoff coefficients have largely influenced flood risk.
Processes of urbanization also lead to increased occupation of flood plains and, often, inadequate drainage planning (H12). These urbanization issues are universal, but often at their worst in informal settlements, where there will be no investment in drainage solutions, and flooding regularly disrupts livelihoods and undermines local food security. A further concern for low- and middle-income cities, particularly in developing countries, is that floods become contaminated with human waste (Hardoy et al. Citation2001), leading to higher rates of infectious disease, such as cholera, cryptosporidiosis and typhoid fever (Kovats and Akhtar Citation2008) occurring after floods.
However, the river stage and thus the risk of flooding also depend on engineered alterations to river courses and depths and artificial flood containment, such as dikes built to protect settlements. Reservoirs, whether developed specifically for flood protection, or intended also to provide water storage, can substantially reduce short-duration flood waves, but during major flood events, their positive effects decrease and may even turn negative.
Costly elements of road infrastructure, such as bridges, culverts and embankments (for roads and railways), are vulnerable to erosional damage in heavy precipitation and floods. Even the structures used to control flood events may aggravate the risk and the resulting damage when an extreme flood occurs. Along the Indus, during the 2010 Pakistan flooding, close to 2000 fatalities occurred and approximately 20 million people were displaced, with the devastating impacts attributed in part to anthropogenically reduced river water and sediment conveyance capacity of the river channel to dam/barrage-related backwater effects and to multiple failures of irrigation system levees. The numerous failures extended from upstream areas on Indus tributaries, where some record discharges did occur, to downstream Indus reaches and the delta, where peak discharges were by no means extreme (Syvitski and Brakenridge Citation2013). Where levees hold, downstream flood peaks are higher than would otherwise have been the case; where they fail, local flood damage can be catastrophic. In coastal areas and behind levees along inland rivers, levee structures meant to protect against flooding have sometimes instead worsened flood damage by not allowing the free discharge of flood water to the sea (e.g. in Thailand in 2011) or overbank water return to the main channel (e.g. in the Pakistan 2010 event).
Seneviratne et al. (Citation2012) assessed that there is limited to medium evidence available to assess climate-driven observed changes in the magnitude and frequency of floods at regional scales. However, the available instrumental records of floods at gauge stations are limited in space and time and because of the confounding effects of changes in land use and engineering. Furthermore, there is low agreement in this evidence and thus overall low confidence at the global scale regarding even the sign of these changes. Changing flood risk is especially problematic in semi-arid regions where spate occurrence and magnitudes can be highly erratic.
4.3.3 Factors related to socio-economic systems
Changes in population size and development, and level of protection, strongly influence changes in exposure to flood hazards.
Over time, population has increased in most flood-prone areas, and the accumulation of assets has increased exposure to loss. There are indications that exposed population and assets have increased more rapidly than overall population or economic growth (Bouwer et al. Citation2007, Bouwer Citation2011) because of increasing concentration in flood-prone areas.
At the same time, improved flood protection and flood management measures have reduced losses in many flood-prone areas, particularly in high-income areas. Investment in flood warning and evacuation procedures may reduce the number of flood fatalities, while having less effect on the levels of material damage.
Based on data from 1970 to 2010, the number of fatalities caused by floods has decreased globally, despite an increase in exposure. This decrease is mostly attributable to fast-paced urbanization in China. Worldwide, economic losses continue to rise bcause of a significant increase in the exposure in flood plains fuelled by rapid economic growth. Also, even in China, the lethal flooding in parts of Beijing in the summer of 2012, which claimed ~80 lives, indicates that urbanization itself can increase flood impacts locally.
4.3.4 Difficulty in identification of climate signals
Assessing the causes of changes in flood hazard is complex (S12, H12); they may be related to both climatic and non-climatic factors, which can be difficult to distinguish in the instrumental record. In particular, those rivers that threaten towns and cities are typically not in their natural state, making it difficult to separate changes in the flow data related to climate from ongoing alterations in land-use change (including urbanization) and altered river regulation. These effects in combination are difficult to quantify, and, for instance, in the case of land cover changes, even the sign of imposed hydrological changes is partly uncertain (e.g. Gerten et al. Citation2008, Pitman et al. Citation2009, Teuling et al. Citation2010, Oliveira et al. Citation2011). Land-use effects on flood risk can only be convincingly demonstrated through model-based analysis; there are simply too many confounding factors for empirically-based attribution except at very local, plot or experimental scales.
Finally, flood frequency analysis deals with discharge records, as commonly obtained via river stage (level) conversions to discharge at gauging stations via a rating curve. Where channel aggradation (ordegradation) is occurring, however, the stage/discharge relation is not stable, and flooding may increase (or decrease), with higher (or lower) river levels occurring with the same flood discharge. Indeed, many sites worldwide make regular revisions of the rating curve to account for the channel changes, but rating curve estimates are particularly problematic for semi-arid wadi systems, where flows may be short-lived and high-magnitude, and the channel cross-section is changing during each flood event. As regards flooding and climate change, however, the focus is on whether meteorology-derived flood peak discharges or flood water volumes are changing.
5 A GLOBAL PERSPECTIVE ON FUTURE FLOOD RISK
5.1 Introduction
Future changes in flood risk are expected to be driven by a combination of potential changes in climate (especially precipitation), catchment conditions and exposure to loss. Interpretation of changes has been the focus of the new Panta Rhei decade of the International Association of Hydrological Sciences (IAHS) (Montanari et al. Citation2013). Eloquent, philosophical discussion of multi-scale changes, backing the Panta Rhei notion, was given by Koutsoyiannis (Citation2013), including climacograms spanning many orders of magnitude of time units.
This section considers first how precipitation may change in the future, then reviews how river flows and flood frequencies may change, before examining potential changes in exposure to flooding and future flood costs.
5.2 Flood-generating precipitation
The assessment of projected changes in heavy precipitation events is of key relevance when attributing related variations in flood risk, as discussed in some depth in S12. The following paragraphs provide a summary of this material.
The SREX report (S12) concluded that it is likely that the frequency of heavy precipitation or the proportion of total rainfall from intense events will increase in the 21st century over many areas of the globe, and that this is particularly the case in the high latitudes and tropical regions and in winter in the northern mid-latitudes (S12). The report also concluded that heavy rainfalls associated with tropical cyclones are likely to increase with continued warming and that there is medium confidence that, in some regions, increases in heavy precipitation will occur despite projected decreases in total precipitation. The stronger CO2 emissions scenarios (A1B and A2) lead to stronger projected increases in the probability (i.e. decreases in the return period) of events considered extreme with respect to the end of 20th century climate (), as well as higher percentage increases in the absolute magnitude of heavy precipitation events with a 20-year return period. The SREX assessment (S12) is comparable with the assessment from the AR4 (Christensen et al. Citation2007), though slightly weaker (“many areas” instead of “most areas” and “likely” instead of “very likely”; see also Nicholls and Seneviratne Citation2013 for details). The weaker SREX assessment reflects post-AR4 studies, indicating large uncertainties in the projection of precipitation extremes associated with uncertainties in models, downscaling techniques and natural variability (Nicholls and Seneviratne Citation2013). The current generation of GCMs do not even preserve mass in the global water balance (see Liepert and Previdi Citation2012), so one should be cautious about projected changes in precipitation extremes at smaller-than-global scales (see Anagnostopoulos et al. Citation2010). Selection of daily precipitation as an important measure, yet not “the” measure, is backed by broad experience in various regions. For instance, in Japan, daily precipitation is a good index for flood defence planning for river basins from a few to 10 000 km2.
Fig. 6 Projected return period (in years) of late-20th century 20-year return values of annual maximum 24-h precipitation rates. The bar plots (see legend for more information) show results for regionally averaged projections for two time horizons, 2046–2065 and 2081–2100, as compared to the late-20th century, and for three different SRES emissions scenarios (B1, A1B and A2). Results are based on 14 general circulation models (GCMs) contributing to the Coupled Model Intercomparison Project (CMIP3). See S12 for defined extent of regions. The “Globe” analysis (inset box) displays the projected return period (in years) of late-20th century 20-year return values of annual maximum daily precipitation rates computed using all land grid points. Source: S12. Adapted from the analysis of Kharin et al. (Citation2007).
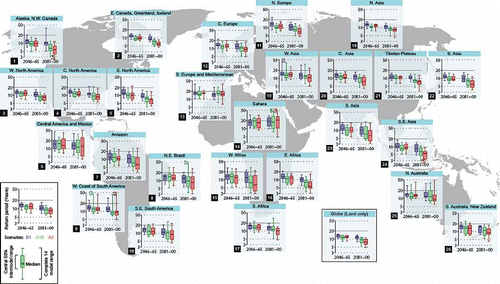
displays the projected return periods for late-20th century “20-year return values” of annual maximum daily precipitation rates in the mid-21st century (on the left of each plot) and in the late-21st century (on the right of each plot) under three different emissions scenarios, SRES B1, A1B and A2. The late-20th century data set was obtained from simulations of the 20th century climate (20C3M) validated with four reanalysis data sets, and return values were estimated from a generalized extreme value distribution fitted at every grid point to samples of precipitation extremes (Kharin et al. Citation2007). Although there are exceptions, the analyses display an overall tendency for a decrease in return periods. Medians fall below the late-20th century return period for all regions except the Sahara (Region 14). The 50% central (inter-quartile) range (coloured boxes in each plot) for the end-of-21st century projections generally falls below the late-20th century return period, except for Southern Europe and the Mediterranean (Region 13), Central America and Mexico (Region 6), and the Sahara (Region 14). For such regions as Alaska/NW Canada and SE Asia, there is the strongest decrease of the median for 2081–2100 (nearly fourfold—from a return period of 20 years to slightly over 5 years, for A2). For the Sahara region (14), the median increases slightly for A2, whereas whiskers range from 5 to 56 years (i.e. some models indicate that the 20-year heavy precipitation in the control period may become about four times more frequent, but other models project that it may become nearly three times less frequent in 2081–2100). The uncertainty is very high in that region, even regarding the direction of change, let alone its magnitude. Hence, this analysis illustrates the strong regional dependency of the projected changes, with overall larger changes in the high-latitude and tropical regions and overall larger uncertainty in drier regions such as the Sahara, Central America and Mexico, as well as Southern Europe and the Mediterranean.
With respect to projected future changes in heavy precipitation events, the SREX report (S12) assessed that there is medium confidence (based on physical reasoning) that projected increases in heavy rainfall would contribute to increases in local flooding in some catchments or regions, and thus these regional variations in projections of changes in heavy precipitation should play an important role in changing flood occurrence at the regional scale.
Stephens et al. (Citation2010) report little skill in precipitation calculated at individual grid cells, using the expression “dreary state of precipitation in global models.” Models produce more frequent and less intense precipitation. Problems of representation of precipitation in climate models from the viewpoint of hydrology are discussed by Koutsoyiannis et al. (Citation2008, Citation2011) and Kundzewicz and Stakhiv (Citation2010).
5.3 River flows and floods
As highlighted in the SREX report (S12), the number of regional- or continental-scale studies of projected changes in river floods is still very limited. Recently, however, a few studies for Europe (Lehner et al. Citation2006, Dankers and Feyen Citation2008) and a global study (Hirabayashi et al. Citation2008) have projected expected changes in the frequency and/or magnitude of floods in the 21st century at a large scale and employing daily river discharges calculated from regional climate model (RCM) or global climate model (GCM) outputs linked with hydrological models. There are relatively few projections of flood changes at the scale of river basins in the scientific literature; most of them apply to Europe and North America (see review in S12).
Hirabayashi et al. (Citation2013) evaluated the spatially distributed flood risk over the globe for the end of the 21st century, based on outputs of 11 climate models and a global river routing model. For an ensemble of projections under a high-concentration scenario (RCP8.5), an event corresponding to a 20th century 100-year flood is projected to be exceeded more frequently in 42% and less frequently in 18% of the land grid cells. Using the inter-model median, a large increase in flood hazard was projected in South Asia and Southeast Asia, as well as in parts of Africa and South America, but a decrease in flood hazard was also projected in certain areas.
5.4 Exposure to flood hazard
In terms of exposure to flooding, about 800 million people worldwide (i.e. over 11% of the global population) are currently living in flood-prone areas and about 70 million of those people (i.e. 1% of the global population) are, on average, exposed to floods each year (UNISDR Citation2011). Even at constant hazard, the population living in flood-prone areas would grow to 940 million, while those exposed to flooding yearly would grow to 86 million per year, based on demographic change alone (Peduzzi et al. Citation2011). Given the lack of satisfactory data sets on past flood events and the uncertainty associated with projected trends in future flood frequencies and magnitudes, it is difficult to estimate future flood impacts. However, using projected population increase in the flood-prone areas, it is possible to look at trends in the number of exposed people (UNISDR Citation2011). shows that population growth will continue to increase exposure to floods. Human exposure is defined as the intersection between hazard probability at a given severity and a population distribution model (Landscan). It can be expressed as the population living in flood-prone areas. However, for comparison purposes, this is provided here as the average absolute number of people exposed per year (annualized figures) or the percentage of the total population exposed per year. Economic exposure is the intersection between hazard probability at a given severity and a distribution model for gross domestic product (GDP). Similarly, economic exposure can be expressed as the value of the assets located in the flood-prone areas, but this is expressed here as the average GDP exposed per year. Note that GDP is considered in terms of revenue and, thus, is only a proxy to the total value of assets exposed. One flood event can destroy several decades worth of GDP.
Fig. 7 Population exposed to floods each year assuming constant hazard, in thousands of people per year (left) and in percentage of total population (right). Annualized values in both absolute and relative terms are used. Areas north of 60° N and south of 60° S, as well as catchments smaller than 1000 km2 (typically small islands) have not been modelled. Data from Peduzzi et al. (Citation2011). Notation: Asia 1 includes all countries north to south from Kazakhstan to Sri Lanka and west to east from the Syrian Arab Republic to Myanmar (therein India and the Arabian Peninsula). Asia 2 includes all countries north to south from Russia (east of Ural and south of 60° N) to Indonesia and west to east from Russia and China to Japan, Philippines and Papua New Guinea.
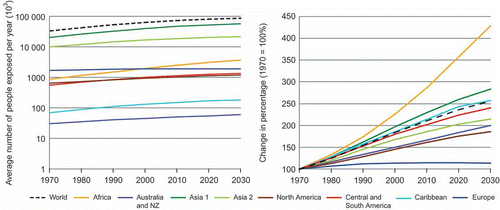
At the global scale, a few studies have estimated the numbers of people affected by increases (or decreases) in flood hazard. Kleinen and Petschel-Held (Citation2007) calculated the percentage of the global population living in river basins in which the return period of the current 50-year flood event becomes shorter, for three climate models and a range of warming scenarios. For an increase in global mean temperature of 2°C above late-20th century temperatures, they found that between 5 and 27% of the world’s population would live in river basins where the current 50-year return period flood occurs at least twice as frequently. Hirabayashi and Kanae (Citation2009) used a different metric, counting each year the number of people living in grid cells where the flood peak exceeded the (current) 100-year magnitude, using runoff as simulated by a high-resolution climate model fed through a river routing model. They projected increases in the number of people affected by substantial flooding. Finally, Hirabayashi et al. (Citation2013) asserted that the global exposure to floods would increase, depending on the degree of warming, but that inter-annual variability of exposure is strong.
5.5 Future costs of flooding
Several studies have addressed future expected economic losses from river floods, most of which are focused on Europe (Bouwer Citation2013) (see ). These studies combine a range of approaches for arriving at estimates of future losses. They account for changes in hazard on the basis of projected climate change and use loss model estimates. They all project an increase in economic flood losses, albeit to different extents. Furthermore, it is important to note that some studies also assessed the combined effects owing to both exposure (related to on-going socio-economic changes) and anthropogenic climate change resulting from greenhouse gas emissions.
Table 1 Projected increase in disaster losses in 2040 under projected climate change and exposure change, relative to 2000, from available impact studies including median estimates.
These studies generally show that the combined effect of climate change and changes in exposure is higher, but also that exposure (increase in the number of assets and their value) is of similar magnitude to changes in climate over this time period. At the same time, these numbers must be considered with care, as uncertainties in these projections are high, the studies use different methods and approaches (Bouwer Citation2013), and many local factors are included in the projections that prevent extrapolation to other regions or the global level. Nevertheless, they provide an indication of future flood risk trends and thereby can support the assessment of future risks.
Economic, including insured, flood disaster losses are higher in developed countries, while relative fatality rates and economic losses expressed as a proportion of GDP are higher in developing countries (high confidence).
6 REGIONAL PERSPECTIVES
The SREX report also assessed changes in flood risk in seven regions: Africa, Asia, Central and South America, Europe, North America, Oceania and the Polar regions, drawing from H12 and S12. Europe and North America have very good data and analyses compared with the other regions, where uncertainties are stronger.
6.1 Africa
The periods of extreme rainfall and recurrent floods appear to correlate with the El Niño phase of ENSO events (e.g. 1982–1983, 1997–1998 and 2006–2007) and generate significant economic and human losses. In 2000, floods in Mozambique, particularly along the valleys of the rivers Limpopo, Save and Zambezi, resulted in 700 reported deaths and about half a million homeless. The floods had a devastating effect on livelihoods, destroying agricultural crops, disrupting electricity supplies and demolishing basic infrastructure (Osman-Elasha et al. Citation2006).
There is low to medium confidence in regional trends in heavy precipitation in Africa due to the scarcity of both previous studies and observational data (see Conway et al. (Citation2009) reviewing the evidence for hydrological change in sub-Saharan Africa), as well as reflecting the lack of consistency in those patterns reported in some regions.
Di Baldassarre et al. (Citation2010) found no evidence that the magnitude of African floods has increased during the 20th century. Yet, projections by Hirabayashi et al. (Citation2008) indicate an expected increase in the risk of floods in tropical Africa.
Heavy precipitation may induce landslides and debris flows in tropical mountain regions, with potential impacts for human settlements. In the arid and semi-arid areas of countries in the Horn of Africa, extreme rainfall events are often associated with a higher risk of the vector and epidemic diseases of malaria, dengue fever, cholera, Rift Valley fever and hantavirus pulmonary syndrome (Anyamba et al. Citation2006, McMichael et al. Citation2006).
However, floods can be highly beneficial in African drylands (e.g. the Sahara and Namib deserts), since the floodwaters infiltrate and recharge alluvial aquifers along ephemeral river pathways, extending water availability to dry seasons and drought years (Morin et al. Citation2009, Benito et al. Citation2010) and supporting riparian systems and human communities.
6.2 Asia
Globally, the most devastating flood disasters, killing thousands of people, continue to occur in Asia. The geographical distribution of flood risk is heavily concentrated in Bangladesh and the two countries with the greatest population—China and India—causing high human and material losses (Bouwer et al. Citation2007, Dash et al. Citation2007, Shen et al. Citation2008, Douglas Citation2009).
Bangladesh is perhaps the most flood-prone country, globally. As noted in the final report for the Ministry of Environment and Forest (Citation2005) of the People’s Republic of Bangladesh, flooding in Bangladesh is a normal, frequently recurrent phenomenon and includes flash floods from the overflowing of upland rivers; rain floods due to poor drainage; monsoon floods in the flood plains of major rivers; and coastal floods following storm surge. In a normal year, river overflows and drainage congestions cause the inundation of 20–25% of the country’s area, while 10-, 50- and 100-year floods are projected to flood 37, 52 and 60% of the total country’s area, respectively. On three occasions in 12 years, in 1987, 1988 and 1998, floods inundated more than 60% of the country, demonstrating that what used to be considered a “100-year flood” may already have become more frequent. The 1998 flood alone led to 1100 deaths, caused inundation of nearly 100 000 km2, left 30 million people homeless and substantially damaged infrastructure (H12).
Severe flooding occurred in Mumbai, India, in July 2005, after 944 mm of rain fell in a 24-hour period (Kshirsagar et al. Citation2006). The consequent flooding affected households, even in more affluent neighbourhoods. Poor urban drainage systems in many parts of India tend to become blocked with debris. Ranger et al. (Citation2011) analysed risk from heavy rainfall in the city of Mumbai and concluded that total losses (direct plus indirect) for a 1-in-100 year event could treble by the 2080s (SRES A2 scenario) compared with the present (increasing from US$700 million to US$2305 million) and that adaptation could help reduce future damages.
Rapid economic development has not only significantly increased the exposure in flood plains, but also influenced investment in flood-risk management infrastructure. There have been increases in flood impacts associated with changes in surrounding environments. Flooding has increased over the past few decades in the Poyang Lake, South China, because of levee construction protecting a large rural population. These levees reduce the area formerly available for floodwater storage, resulting in higher lake stages during the summer flood season and the potential for catastrophic levee failures (Shankman et al. Citation2006).
Heavy rainfall and flooding also affect environmental health in urban areas, because surface water can be quickly contaminated. In Dhaka, Bangladesh, the severe flood in 1998 was associated with an increase in diarrhoea among those from a lower socio-economic group and not using tap water (Hashizume et al. Citation2008).
A record-breaking, destructive flood occurred in Thailand (), where the 2011 monsoon season was exceptionally heavy and led to extensive and long-lasting flooding in the Chao Phraya and Mekong river basins. Flooding was exacerbated by the rapid expansion of urban areas into flood plains. The flooding lasted from July 2011 to mid-January 2012 and was the costliest natural disaster in the country’s history, with direct damages estimated at US$45 billion. As a result, Thailand’s annual 2011 economic growth was slashed to 1.5% from a pre-flood estimate of 3.5–4%. In part, the severity of this event was due to failure of flood control structures and systems that had effectively alleviated the damage from smaller events in the past. The 2011 flood in Thailand caused the most expensive insurance loss ever, worldwide, caused by a flood, with the total liability estimated at ~US$15 billion.
Fig. 8 Flooding in Southeast Asia as mapped by MODIS satellite imaging between 2 November and 4 December, 2011 (red); light red: previous flooding during 2011. The coastal city of Bangkok is shown near the left margin, centre, and is where an unprecedented US$15 billion total insurance loss is estimated.
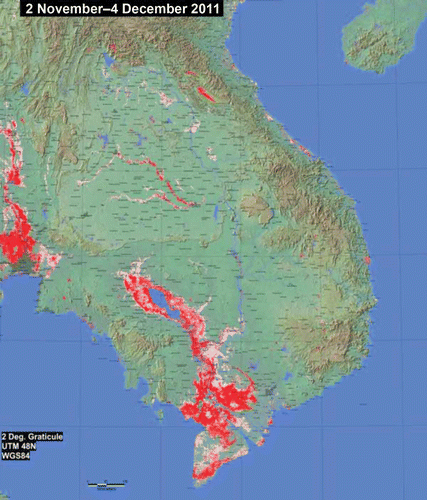
Seasonal accelerated cycles of ice-melting have affected the stability of glacial lakes in the Himalayas, increasing the potential risk of glacial lake outburst floods (GLOF) in the region (Ives et al. Citation2010). A small but not statistically significant increase of GLOF events was observed in the Himalayas since 1940 until the end of the 20th century (Richardson and Reynolds Citation2000), with a greater risk to water infrastructures and socio-economic damage on riverine settlements (Rosenzweig et al. Citation2007).
There is low to medium confidence in trends in recorded heavy precipitation in Asia. No systematic spatially coherent trends in the frequency and duration of extreme precipitation events have been found over much of Asia, although statistically significant trends were observed at sub-regional scales (for review, see S12).
Different flooding trends have been detected and projected in various catchments, but the evidence for broader regional trends is limited.
In the Asian monsoon region, heavy precipitation is projected to increase, notably in Bangladesh and in the Yangtze River basin, because of the intensified convergence of water vapour flux in summer. Based on a Japanese GCM, heavy precipitation is projected to increase substantially in South Asia (Kamiguchi et al. Citation2006). Projections by Hirabayashi et al. (Citation2008) show an increase in the risk of floods in most humid-affected Asian monsoon regions. However, GCMs generally cannot adequately reproduce observed links between SST anomalies and the strength of the South Asian monsoon (see Annamalai et al. Citation2007).
6.3 Central and South America
Extreme rainfall episodes have caused disasters in parts of South America, with hundreds to thousands of fatalities in mudslides and landslides, as typified, for example, by the December 1999 incident in Venezuela (Lyon Citation2003). However, there is low to medium confidence in observed and in projected changes in heavy precipitation in the region.
In the Amazon region in South America, the July 2009 flood set record highs in the 106 years of data for the Rio Negro at the Manaus gauge site (Marengo et al. Citation2012). Recent increases have also been reported in flood frequency of some other river basins in South America (Camilloni and Barros Citation2003, Barros et al. Citation2004).
Outbreaks of malaria were associated with changes in habitat after the 1991 floods in Costa Rica’s Atlantic region (Saenz et al. Citation1995).
In the Andes, glacier retreat in the Patagonian ice fields has caused repeated outburst floods, although there remains a debate as to whether this reflects a direct relationship with increased global temperatures (Dussaillant et al. Citation2010).
As assessed in the SREX report (S12), spatially varying trends in extreme rainfall events have been observed in Central and South America (low to medium confidence). This reflects an increased frequency of heavy events in many areas, but also a decreased frequency of heavy events in some areas for Central America and northern South America. For the western coast of South America, there has been a decrease in extreme rainfall in many areas, but an increase in a few areas.
Simulations with a single RCM (PRECIS) under IPCC emissions scenarios SRES A2 and B2 project an increase in the intensity of extreme precipitation events over most of southeastern South America and western Amazonia in 2071–2100, whereas in Northeast Brazil and eastern Amazonia, smaller or no changes are projected (Marengo et al. Citation2009).
6.4 Europe
Despite much investment in flood protection, flooding remains a serious problem throughout the continent. Large parts of Europe have been hit by major floods in recent decades, with multiple fatalities and average multi-billion (in US$ or Euro) material damages each year (Kundzewicz et al. Citation2012). Economic losses from flood hazards in Europe have increased considerably over previous decades (Lugeri et al. Citation2010), driven principally by the increasing exposure of people and economic assets (Barredo Citation2009).
The SREX report assessed that there is medium confidence in trends for heavy precipitation in Europe, observed to date, because of partly inconsistent signals across studies and regions, especially in summer. Increasing trends in high percentiles of daily winter precipitation were found (Moberg et al. Citation2006), confirmed by several more detailed country-based studies (see S12, Hattermann et al. Citation2013). In southern Europe and the Mediterranean region, there is low confidence in the trends, with opposite signals in the observed records (Benito and Machado Citation2012). A recent study (Zolina et al. Citation2010) has indicated that there has been an increase by about 15–20% in the persistence of wet spells over most of Europe over the last 60 years, which was not associated with an increase of the total number of wet days.
There are many national flood studies in Europe (see reviews by S12 and 14 national and regional chapters in the book edited by Kundzewicz Citation2012), but geographically organized patterns of climate-driven changes in the flood magnitude/frequency cannot be detected. Yet, an increase in the number of large floods in Europe in 1985–2010 has been demonstrated by Kundzewicz et al. (Citation2013).
Using daily statistics from various models for European sub-regions under the IPCC SRES A2 scenario, Boberg et al. (Citation2009, Citation2010) projected a clear increase in the contribution to total precipitation from more intense events. For 2071–2100, projected European precipitation extremes in high-resolution studies tend to increase in northern Europe, especially during winter.
However, the projected increase in frequency and intensity of heavy precipitation over large parts of Europe may increase the probability of floods, and in particular flash floods, which pose the highest risk of fatality (EEA Citation2004). If climatic projections are correct, a notable—and beneficial—decrease in the probability of floods that generally corresponds to lower flood peaks is expected for northeastern Europe in the late 21st century, because of a reduction in snow accumulation (Dankers and Feyen Citation2008, Hirabayashi et al. Citation2008).
The SREX report (H12) assessed combinations of estimates of current and future damage potential (as represented by a relationship between flood damage and flood magnitude) with estimates of current and future flood frequency curves to determine damages. A wide range in projected average annual damage was found in the UK Foresight Future Flooding and Coastal Defence project (Evans et al. Citation2004, Hall et al. Citation2005), which calculated average annual damage in 2080 of £1.5 billion, £5 billion and £21 billion under similar climate scenarios but different socio-economic futures (current average annual damage was estimated at £1 billion). The Foresight project represented the effect of climate change on flood frequency by altering the shape of the flood frequency curve using precipitation outputs from climate models and rainfall–runoff models for a sample of UK catchments.
A more recent UK study (Ramsbottom et al. Citation2012, data tables FL6b and FL7b, Appendix 8) stated that river flood damages for England and Wales (in 2010 prices, with consideration of climate change only; without change in exposure) are projected to increase from a baseline of £0.8 billion (1961–1990 climate, 2008 population) to the following levels in 2050: £0.85 billion (+6.3%), £1.9 billion (+138%), and £3 billion (+275%), for low, medium and high climate change, respectively, under assumption of a year-2008 population.
The EU-funded Projection of Economic impacts of climate change in Sectors of the European Union based on bottom-up Analysis (PESETA) project (Ciscar Citation2009, Feyen et al. Citation2009) used a hydrological model to simulate river flows, flooded areas and flood-frequency curves from climate scenarios derived from RCMs, but—in contrast to the UK Foresight project—assumed no change in economic development and in population in flood-prone areas. There are strong regional variations in impact, with particularly large projected increases in both the number of people flooded and economic damages (over 200%) in Central and Eastern Europe, while in parts of northeastern Europe, average annual flood damages decrease. Maaskant et al. (Citation2009) addressed future loss of life from flooding and projected up to a fourfold increase in potential flood victims in The Netherlands by the year 2040, when population growth is also accounted for.
Apart from newly developed urban areas, linear infrastructure, such as roads, railroads and underground rails with inadequate drainage, will probably suffer more flood damage (Defra Citation2004, Arkell and Darch Citation2006, ASC Citation2012). Increased runoff volumes may increase risk of dam failure with high environmental and socio-economic damages (Rico et al. Citation2008). In glaciated areas of Europe, glacial lake outburst floods, although infrequent, have the potential to produce immense socio-economic and environmental impacts, even at long distances downstream from the hazard source area (Haeberli et al. Citation2001, Huggel et al. Citation2004).
6.5 North America
There have been a number of disastrous floods in the last two decades. In 1993, approximately 3.3 million ha of American Midwest soybean and corn fields were flooded, leading to a 50% decrease in corn yields in Iowa, Minnesota and Missouri and a 20–30% decrease in three other states (Changnon Citation1996). Flood impacts also included significant damage to transportation infrastructure. Flooding in the Mississippi Valley continues to constitute a risk to the central USA economy. In May 2011, the cities of Baton Rouge and New Orleans, and numerous oil refineries and chemical plants, narrowly escaped catastrophic inundation by the Lower Mississippi. The combined Missouri–Mississippi basin includes large areas, as well, where spring snowmelt can cause severe local flooding and contribute to an eventual high peak far downstream.
As assessed in the SREX report (S12), there has been a likely increase in heavy precipitation in many areas of North America since 1950, with projections suggesting further increases in heavy precipitation in some regions.
Recent studies on past and current changes of precipitation extremes in North America, some of which are included in the recent assessment of the USA Climate Change Science Program (CCSP) report (Kunkel et al. Citation2008), have reported an increasing trend over the last half century. On the basis of station data from Canada, the United States and Mexico, Peterson et al. (Citation2008) reported that heavy precipitation, as well as the average amount of precipitation falling on days with precipitation, has been increasing in the study interval of 1950–2004. For the contiguous United States, DeGaetano (Citation2009) showed a 20% reduction in the return period for extreme precipitation of different return levels over 1950–2007; Gleason et al. (Citation2008) reported an increasing trend in the area experiencing a much above-normal proportion of heavy daily precipitation from 1950 to 2006 and Pryor et al. (Citation2009) provided evidence of increases in the intensity of precipitation events above the 95th percentile during the 20th century, with a larger magnitude increase at the end of the century. Overall (S12), the evidence indicates a likely increase in observed heavy precipitation in many regions of North America, despite statistically nonsignificant trends and some decreases in some sub-regions.
In the United States and Canada during the 20th century and in the early 21st century, there is no compelling evidence for climate-driven changes in the magnitude/frequency of floods (Lins and Slack Citation1999, McCabe and Wolock Citation2002, Cunderlik and Ouarda Citation2009, Villarini et al. Citation2009). A study in the United States (Choi and Fisher Citation2003) constructed regression relationships between annual flood loss and socio-economic and climate drivers, concluding that a 1% increase in average annual precipitation would, other things being equal, lead to an increase in annual national flood loss of around 6.5%. However, the conclusions are highly dependent on the methodology and the spatial scale of analysis.
Wang and Zhang (Citation2008) investigated possible changes in North American extreme winter precipitation comparing 1949–1999 with 2050–2099. Their results suggested a strong increase in extreme precipitation over the south and central United States, but decreases over the Canadian prairies. Also, Gutowski et al. (Citation2008) showed that, for the Upper Mississippi River Basin region during October–March, the intensity of extreme precipitation is projected to increase.
Flooding and heavy precipitation events have a variety of significant human health impacts (Ebi et al. Citation2008). Heavy precipitation events are strongly correlated with the outbreak of waterborne illnesses in the United States, and 51% of waterborne disease outbreaks were preceded by precipitation events in the top decile (Curriero et al. Citation2001). In addition, heavy precipitation events in North America have been linked to outbreaks of vector-borne diseases, such as hantavirus and plague (Engelthaler et al. Citation1999, Parmenter et al. Citation1999, Hjelle and Glass Citation2000). There is a risk of extreme floods causing major toxic chemical spills into flooded areas.
6.6 Oceania
The SREX report assessed that there has likely been a decrease in heavy precipitation in many parts of southern Australia and New Zealand. However, there were statistically significant increases in the proportion of annual/seasonal rainfall stemming from heavy rain days between two periods, 1911–2008 and 1957–2008, in northwest Australia (Gallant and Karoly Citation2010). Extreme summer rainfall over the northwest of the Swan-Avon River basin in Western Australia increased over 1950–2003, while extreme winter rainfall over the southwest of the basin decreased (Aryal et al. Citation2009). In New Zealand, the trends are positive in the western North and South Islands and negative in the east of the country (Mullan et al. Citation2008).
Since the SREX report was prepared, there has been record-breaking flooding across parts of eastern Australia. Flooding in the states of Victoria and Queensland (including parts of the capital city, Brisbane) during the austral summer of 2010/11 resulted in major economic impacts and subsequent public enquiries (Wilby and Keenan Citation2012). Wide-ranging recommendations on most aspects of flood-risk management, including land-use planning to reduce exposure, have followed (e.g. Goode et al. Citation2011). There have been improvements in flood mapping and warnings. However, development pressures make implementation of other measures more challenging (Wenger et al. Citation2013).
As shown in , the return period of the 20th century 20-year return value of annual maximum 24-h precipitation is projected to decrease in the Oceania region in the future, but there is generally low to medium confidence in projections of heavy precipitation because of a lack of consistency between models (Alexander and Arblaster Citation2009). Floods are New Zealand’s most frequently experienced hazard (OCDESC Citation2007), affecting both agricultural and urban areas. Being long and narrow, New Zealand is characterized by small river catchments and, accordingly, shorter time-to-peak and shorter flood warning times, posing a difficult preparedness challenge. Projected increases in heavy precipitation are expected to cause greater erosion of land surfaces, more landslides and a decrease in the protection afforded by levees (Hennessy et al. Citation2007).
6.7 Polar regions
Polar regions in the IPCC classification cover high-latitude areas in both the Northern and Southern hemispheres, characterized by low population densities. From the mid-1960s to the beginning of the 1990s, winter runoff in the three largest rivers of Siberia (Yenisei, Lena and Ob), jointly contributing approximately 70% of the total river runoff to the Arctic Ocean, increased by 165 km3 (Savelieva et al. Citation2004). Rivers in Arctic Russia experience floods, but their frequency, stage and incidence are different across this large region, depending on flood formation conditions. Floods on the Siberian rivers can be produced by a high peak in the spring flood, by rare heavy rain, by a combination of snow and rain, as well as by ice jams, hanging dams and combinations of factors (Semyonov and Korshunov Citation2006).
Maximum river discharge was found to decrease from the mid-20th century through 1980 in Western Siberia and the Far East (except for the Yenisei and the Lena rivers). However, since the 1980s, maximum streamflow values began to increase over much of Russia (Semyonov and Korshunov Citation2006). Snowmelt and rain continue to be the most frequent causes of hazardous floods on the rivers in the Russian Arctic (85% of all hazardous floods in the past 15 years), while ice jams and wind tides make up 10% and 5% of the total number of hazardous floods, respectively. Pomeranets (Citation2005) suggests that the probability of catastrophic ice jam-related floods increased in the early 21st century.
Although changes in flood magnitude/frequency might be expected in regions where temperature change affects precipitation type (i.e. rain/snow separation), as well as snowmelt and ice cover, widespread evidence of such climate-driven changes in floods is not available. For example, there is no evidence of widespread common trends in the magnitude of floods based on the daily river discharge of 139 Russian gauge stations, though a significant shift of spring discharge to earlier dates has been found (Shiklomanov et al. Citation2007). However, for 19 large rivers encompassing the entire Arctic region over the period 1977–2007, Overeem and Syvitski (Citation2010) found a consistent increase in annual discharge over the entire region (+9.8%). Combined change in water outflux is significantly higher than previous reconstructions for the Canadian Arctic (+2% over 1964–2000) and Eurasia (+7% over 1936–1999). However, the period of 30 years used by Overeem and Syvitski (Citation2010) is short for evaluation of changes in flooding, as at such a time scale climate oscillations can be expected to be dominant.
7 ATTRIBUTION AND UNCERTAINTY ASPECTS
The SREX report concluded that there was a lack of studies identifying, in a persuasive way, an influence of anthropogenic climate change on rain-generated peak streamflow trends. Overall, there is low confidence (due to limited evidence) that anthropogenic climate change has already affected the magnitude/frequency of floods, though it has detectably influenced several components of the hydrological cycle, such as precipitation and snowmelt, which impact on floods. The assessment of causes behind changes in flooding behaviour is inherently complex and difficult.
Using 200 long-term (85–127 years of record) stream gauge flow series in the coterminous United States in locations with little or no regulation or urban development, Hirsch and Ryberg (Citation2012) did not find strong statistical evidence for flood magnitudes increasing with increasing global mean carbon dioxide concentration (GMCO2) in any of the four regions defined in this study. One region, the southwest, showed a statistically significant negative relationship between GMCO2 and flood magnitudes.
A recent model-based study by Pall et al. (Citation2011) showed that increasing global anthropogenic greenhouse-gas emissions could have increased the risk of rainfall-dominated flood occurrence in the flooding of some river basins in England and Wales, as observed in autumn (September–November) 2000 and as associated with a displacement in the North Atlantic jet stream. This study showed that there is now about 50% chance that an anthropogenic influence can be detected for UK extreme precipitation in winter, but the likelihood of the detection in other seasons is very small (Fowler and Wilby Citation2010).
Trenberth (Citation2012) postulated a link between record high sea-surface temperatures (SSTs) and subsequent occurrence of floods in affected adjacent areas. According to this postulate, the presence of record high SSTs might be related to floods in China, India and Pakistan in the summer of 2010, Queensland in Australia in the austral summer 2010/11 (Evans and Boyer-Souchet Citation2012), Colombia in October–December 2010, and the United States in spring 2011 (although the very strong La Niña event of 2010/11 may have played the major role in some of these events).
The SREX report (H12) assessed that the exposure of people and economic assets has been the major cause of long-term increases in economic losses from floods. Long-term trends in economic disaster losses, adjusted for wealth and population increases, have not been attributed to climate change. The attribution of economic disaster losses is subject to a number of limitations in studies to date, including data availability (most data are available for standard economic sectors in developed countries) and the processes used to normalize loss data over time. Published studies have used different approaches to normalization, which take account of changes in exposure of people and assets, but use only limited, if any, measures of changes in vulnerability (Bouwer Citation2011). Different approaches are also used to handle variations in the quality and completeness of data on impacts over time. Finding a trend or “signal” in a system characterized by large variability or “noise” is difficult and requires lengthy records (Kundzewicz et al. Citation2005). A general area of uncertainty in the studies concerns the impacts of floods on the livelihoods and people of informal settlements and economic sectors, especially in developing countries. Some one billion people live in settlements developed outside government planning and building systems (UNISDR Citation2011) and over half the economy in some developing countries is informal or undocumented (Schneider et al. Citation2010).
In many regions, the monitoring network is very sparse, resulting in considerable uncertainty in the estimates of extreme precipitation. There are also known biases in precipitation measurements, mostly leading to rain undercatch. As highlighted in the SREX report, little evidence of pre-instrumental changes in heavy precipitation is available to place recent variations in context (S12).
The SREX report (S12) assessed that the projection of precipitation extremes is associated with large uncertainties, contributed by climatic models and by natural variability of the climate. The underestimation of rainfall extremes by the models (often observed when models simulate historical data) may be related to the coarse spatial resolution used in the model simulations, suggesting that projections of future changes in rainfall extremes in response to anthropogenic global warming may also be underestimated.
All GCM projections of precipitation extremes must come with a heavy caveat, because of the fundamental limitations, such as, for example, omission of land-cover feedbacks, failure to conserve the global water balance, or poor representation of planetary-scale teleconnections effecting monsoon regions.
River discharge simulation under a changing climate scenario requires a set of GCM or RCM outputs (e.g. precipitation and surface air temperature) and a hydrological model (S12). Alternative hydrological models may yield quantitatively different river discharges, but they may not yield different signs of the trend if the same GCM/RCM outputs are used. So the ability of models to simulate floods, and in particular the signs of past and future trends, depends on the ability of the GCM/RCM to simulate precipitation changes. The ability of a GCM/RCM to simulate temperature is also important for river discharge simulations in snowmelt- and glacier-fed rivers. Worldwide instrumental records of floods at gauge stations are limited in spatial coverage and in time, and only a very limited number of gauge stations have data that span a time period of 100 years or more (Rodier and Roche Citation1984).
There is strong uncertainty in the projected changes in the magnitude and frequency of floods, while the GCM remains the largest source of uncertainties in hydrological projections for the forthcoming decades at the river-basin scale. Model skill in reproducing extreme storm events and trends in extreme storm events, given some change in forcing, is not yet persuasive. Uncertainties from emissions scenarios and downscaling methods are also relevant, but likely smaller in magnitude (Graham et al. Citation2007, Leander et al. Citation2008). However, the relative uncertainty mix depends on the future time horizon, as uncertainties from emissions scenarios assume greater importance further into the future. Various techniques of downscaling and/or bias-correction are applied to GCM/RCM outputs (e.g. precipitation and temperature) before hydrological simulations are conducted. We cannot judge which approach is the best, hence the selection of downscaling or bias correction is a source of uncertainty.
Furthermore, the choice of hydrological models was found to be relevant but less important (Taye et al. Citation2011). However, the relative importance of downscaling, bias-correction and the choice of hydrological models may depend on the selected region/catchment, the selected downscaling and bias-correction methods and the selected hydrological models (Wilby et al. Citation2008).
Upon reviewing the estimates to date, the costing of flood losses and estimation of adaptation costs is still preliminary, incomplete and subject to a number of assumptions, with the result that considerable uncertainty remains (Agrawala and Fankhauser Citation2008, Parry et al. Citation2009). This is largely due to uncertainties in projected climate change and associated damage estimates, as well as methodological shortcomings. Such costing is further limited by the interaction between numerous adaptation options and assumptions about future exposure and vulnerabilities, social preferences and technology, as well as levels of resilience in specific societies. Another major knowledge gap is changing flood risk within urban areas (under climate change), since most model studies refer to “natural” basins.
Potentially, remote sensing may offer a cost-effective solution for monitoring precipitation, but for now, these records are short and the issue of exploring trends when there is a major change in measurement method is a very serious one. Satellite sensors provide a synoptic and global coverage. The collection and archives of data can be automated, thus providing a rapid update for monitoring, with little human intervention in relation to the area covered. In remote locations, it is often the only source of information. Satellite sensors, however, cannot replace ground meteorological stations. First, the ground stations are needed for calibrating the sensors; second, ground stations provide a much longer period of records, which is needed for climate change studies; hence, their network should be maintained. Remote sensing has the potential to be increasingly important as the method for interpretation of flood levels and inundated areas.
8 CONCLUDING REMARKS
Based on the evidence recently assessed in the SREX report (S12), one can assess at present that it is likely that there have been statistically significant increases in the number of heavy precipitation events (e.g. 95th percentile of 24-h precipitation totals of all days with precipitation) in more regions than there have been statistically significant decreases, but there are strong regional and sub-regional variations in the trends, both between and within regions. Based on cumulative evidence, there is additionally medium confidence that anthropogenic influence has contributed to the intensification of heavy precipitation at the global scale, though attribution at the regional scale is not feasible at present. Projected changes from both global and regional studies indicate that it is likely that the frequency of heavy precipitation, or the proportion of total rainfall from heavy falls, will increase in the 21st century over many areas of the globe, especially in the high-latitude and tropical regions and northern mid-latitudes in winter. Heavy precipitation is projected to increase in some (but not all) regions with projected decreases of total precipitation (medium confidence).
Despite the diagnosed extreme-precipitation-based signal, and its possible link to changes in flood patterns, no gauge-based evidence had been found for a climate-driven, globally widespread change in the magnitude/frequency of floods during the last decades. As assessed in the SREX report, projected temperature and precipitation changes imply changes in floods, although overall there is low confidence in projections of changes in fluvial floods, due to limited evidence and because the causes of regional changes are complex. There is medium confidence (based on physical reasoning) that projected increases in heavy rainfall would contribute to increases in rain-generated local flooding in some areas. Earlier spring peak flows in snowmelt- and glacier-fed rivers are very likely, but there is low confidence (due to limited evidence) in their projected magnitude.
There are not many projections of flood magnitude/frequency changes at regional and continental scales. Projections at the river-basin scale are also not abundant, especially for regions outside Europe and North America. In addition, considerable uncertainty remains in the projections of changes in flood magnitude and frequency. Therefore, there is low confidence in future changes in flood magnitude and frequency derived from river discharge simulations.
Kundzewicz et al. (Citation2007, Citation2008) and Bates et al. (Citation2008) argued that climate change (i.e. observed increase in precipitation intensity and other observed climate changes) might already have had an impact on floods and that physical reasoning suggests that projected increases in heavy rainfall in some catchments or regions would contribute to increases in rain-generated local floods (medium confidence). They argued that more frequent heavy precipitation events projected over most regions would affect the risk of rain-generated floods (e.g. flash flooding and urban flooding).
Changes in precipitation totals, frequency and intensity, along with snow cover, snowmelt and soil moisture, are all relevant for causing changes in floods. However, confidence in the magnitude of change of one of these components alone (and—over some regions—even in the sign of change) may not be sufficient to confidently project changes in flood occurrence.
According to UNISDR (Citation2011), the global mortality risk from floods increased from 1980 to 2000, but is now stabilizing and even reducing, because of a compensation of the increase in exposure by a significant decrease in vulnerability. However, the global trend is largely influenced by developments in China (in particular around vertical urbanization). If China is removed from the data set, the mortality risk is increasing (UNISDR Citation2011). Economic losses from floods have increased considerably (H12), but with large spatial and inter-annual variability (high confidence, based on high agreement, medium evidence). Global flood disaster losses reported over the last few decades reflect mainly monetized direct damages to assets. Trends in the vulnerability of what is exposed are variable by location, and the global picture is strongly influenced by China, where vulnerability to floods has decreased over the past decade. Current studies indicate that increasing exposure of population and assets, and not anthropogenic climate change, is responsible for the past increase in flood losses. While early warning systems can successfully reduce mortality risk through evacuation of the population, crops and infrastructures remain in place, and hence the significant increase in infrastructures has led to a drastic increase in economic risk (Bouwer Citation2011, UNISDR Citation2011). Studies that project future flood losses and casualties indicate that, when no adaptation is undertaken, future anthropogenic climate change is likely to lead to increasing flood losses, alongside the increase in exposure linked to ongoing economic development (H12). Where rapid urbanization brings inadequately engineered in-city drainage infrastructure as well, its effect can promote rather than decrease losses to both the economy and human lives. In general, human modifications of watersheds, changing their runoff characteristics (e.g. urbanization, deforestation, building of flood walls and dams, and artificial land drainage), lead to flood risk changes.
The impacts of changes in flood characteristics will be highly dependent on how climate changes in the future, and there is low confidence in specific projections of changes in flood magnitude or frequency. However, even in the absence of increased flood risk owing to increased heavy precipitation or other modifications of the climate system, flood risk will generally increase as exposure continues to rise. Hence, flood adaptation strategies need to jointly consider landscape changes that affect flood response, the location and protection of people and property at risk, as well as changes in flood risk due to changes in climate. All three are of critical importance to the future of flood hazards and economic losses due to flooding.
There are at least two ways of interpreting the notion of “early flood warning” (Kundzewicz Citation2013). The more common interpretation of the notion refers to a short-term flood preparedness system, where a flood warning contains specific timely information, based on a reliable forecast, that a high water level is expected at a particular location and time. A warning should be issued sufficiently early before the potential inundation to allow adequate preparation and to ensure that emergency actions, such as strengthening dikes or evacuation, can be undertaken, so that flood damage to people and property is reduced. The appropriate timeframe is affected by the catchment size relative to the vulnerable zones. The warning should be expressed in a way that persuades people to take appropriate action. The solutions lie in the area of improved weather and hydrological forecasting, better weather and hydrologic networks and better real-time risk communication.
The other interpretation of “early flood warning” is a statement that a high water level or discharge is likely to occur more (or less) frequently in a time scale of decades. Technically, this constitutes a “prediction” of a change in flood frequency compared to a reference period. An early warning of this type could, for example, predict that at a site of concern, the current 100-year flood (river flow exceeded once in 100 years on average in the reference period) may become a 50-year flood in a defined future time horizon. Despite the inherent uncertainty, such an early warning would be an important signal for decision-makers that the required level of protection is unlikely to be maintained in the future unless flood preparedness is improved.
The linkages between enhanced greenhouse forcing and flood phenomena are highly complex and, up to the present, it has not been possible to describe the connections well, either by empirical analysis or by the use of models. It is clear that current trends in human activity on the landscape continue to cause an increase in flood damages. Decreasing or reversing this trend will require substantial attention from governments, private citizens, scientists and engineers, and the actions needed to accomplish this are largely the same regardless of the nature of the greenhouse gas–flood linkage. If anything, the state of the science of regarding this linkage should cause decision-makers to take a more cautious approach to flood adaptation because of the added uncertainty that enhanced greenhouse forcing has introduced. There is such a furore of concern about the linkage between greenhouse forcing and floods that it causes society to lose focus on the things we already know for certain about floods and how to mitigate and adapt to them. Blaming climate change for flood losses makes flood losses a global issue that appears to be out of the control of regional or national institutions. The scientific community needs to emphasize that the problem of flood losses is mostly about what we do on or to the landscape and that will be the case for decades to come.
The climate change issue is very important to flooding, but we have low confidence about the science. What should the science community, engineers, citizens or governments do with that? The precautionary principle is a right choice. Doing the right things should not depend on waiting for the answers to the greenhouse forcing–flood issue. The continuation of empirical and model-based science and a “no regrets” strategy for limiting flood losses should be encouraged. However, work towards improvements in GCMs to bring us to a point where all of this is made clear is much needed, and this may take much time (Kundzewicz and Stakhiv Citation2010).
Although media reports of both floods and global flood damage are on the increase, there is still no Mauna-Loa-like record (see Vörösmarty Citation2002) that shows a global increase in flood frequency or magnitude.
Acknowledgements
The authors thank all the authors of the myriad references assessed in the IPCC Special Report on Managing the Risks of Extreme Events and Disasters to Advance Climate Change Adaptation. Much of the material draws from chapters 3 and 4 of this Special Report. The reader is referred to the original report (Field et al. Citation2012) for the extensive list of publications that were subject to assessment. Support of work on this article is gratefully acknowledged. Constructive and useful comments by three referees (two eponymous reviewers: Dr Robert Hirsch and Professor Robert Wilby, and one anonymous referee), as well as Professor Demetris Koutsoyiannis, HSJ Co-editor, are gratefully acknowledged. Some of these comments indeed helped the authors improve their article.
Funding
The contribution of ZWK has been supported by the project (PSPB no. 153/2010), FLOod RISk on the nothern foothills of the Tatra Mountains (FLORIST), supported by a grant from Switzerland through the Swiss Contribution to the enlarged European Union. This research was also supported by the Environment Research and Technology Development Fund (S-10) of the Ministry of the Environment, Japan.
Notes
1. Calibrated language is used herein to characterize the degree of certainty authors have in statements about the state of current scientific understanding, following the guidance used in the IPCC SREX (Mastrandrea et al. Citation2010). Author teams of IPCC assessments were charged with applying expert judgement, following from their comprehensive assessment of the literature. Confidence in the validity of a finding is based on the type, amount, quality and consistency of evidence (e.g. mechanistic understanding, theory, data, models and expert judgement) and the degree of agreement. Levels of confidence are expressed qualitatively through five qualifiers: very low, low, medium, high and very high. This guidance framework includes summary terms to describe the available evidence: limited, medium or robust, and the degree of agreement: low, medium or high. Where quantified measures of uncertainty in a finding can be expressed probabilistically (based on statistical analysis of observations or model results, or expert judgement), a finding can include calibrated likelihood language. The likelihood terms and their corresponding probability ranges are as follows: virtually certain, 99–100% probability; very likely, 90–100% probability; likely, 66–100% probability; about as likely as not, 33–66% probability; unlikely, 0–33% probability; very unlikely, 0–10% probability; and exceptionally unlikely, 0–1% probability. The great strength of the common use of calibrated language is that comparisons can be made across the findings of the IPCC SREX so that readers of the statements may understand the degree to which understanding is well-established or still reflects competing explanations. The use of calibrated uncertainty language is strongly intertwined with the value the assessment process adds to the abundant literature on which it is based.
2. To be included in the SREX report, references had to be accepted for publication by the cut-off date of 31 May 2011.
REFERENCES
- Agrawala, S. and Fankhauser, S., eds., 2008. Economic aspects of adaptation to climate change, costs, benefits and policy instruments. Paris: OECD.
- Alexander, L.V., et al., 2006. Global observed changes in daily climate extremes of temperature and precipitation. Journal of Geophysical Research—Atmospheres, 111, D05109.
- Alexander, L.V. and Arblaster, J.M., 2009. Assessing trends in observed and modelled climate extremes over Australia in relation to future projections. International Journal of Climatology, 29 (3), 417–435.
- Anagnostopoulos, G.G., et al., 2010. A comparison of local and aggregated climate model outputs with observed data. Hydrological Sciences Journal, 55 (7), 1094–1110.
- Annamalai, H., Hamilton, K., and Sperber, K.R., 2007. The South Asian summer monsoon and its relationship with ENSO in the IPCC AR4 simulations. Journal of Climate, 20, 1071–1092.
- Anyamba, A., et al. 2006. Developing global climate anomalies suggest potential disease risks for 2006–2007. International Journal of Health Geographics, 5, 60.
- Arkell, B.P. and Darch, G.J.C., 2006. Impact of climate change on London’s transport network. In: Proceedings of the Institution of Civil Engineers: municipal engineer, 159. London: ME4, 231–237.
- Aryal, S.K., et al., 2009. Characterizing and modeling temporal and spatial trends in rainfall extremes. Journal of Hydrometeorology, 10 (3), 241–253.
- ASC (Adaptation Sub-Committee), 2012. Climate change—is the UK preparing for flooding and water scarcity? Adaptation Sub-Committee Progress Report 2012.
- Barnett, T.P., et al., 2008. Human-induced changes in the hydrology of the western United States. Science, 319 (5866), 1080–1083.
- Barredo, J.I., 2009. Normalised flood losses in Europe: 1970–2006. Natural Hazards and Earth System Sciences, 9, 97–104.
- Barredo, J.I., Sauri, D., and Llasat, M.C., 2012. Assessing trends in insured losses from floods in Spain 1971–2008. Natural Hazards and Earth System Sciences, 12, 1723–1729.
- Barros, V., et al., 2004. The major discharge events in the Paraguay River. Journal of Hydrometeorology, 5 (6), 1161.
- Barthel, F. and Neumayer, E., 2012. A trend analysis of normalized insured damage from natural disasters. Climatic Change, 113 (2), 215–237.
- Bates, B.C., et al., 2008. Climate change and water. Technical paper of the Intergovernmental Panel on Climate Change. Geneva: IPCC Secretariat.
- Benito, G., et al., 2010. Management of alluvial aquifers in two southern African ephemeral rivers: implications for IWRM. Water Resources Management, 24, 641–667.
- Benito, G. and Machado, M.J., 2012. Floods in the Iberian Peninsula. In: Z.W. Kundzewicz, ed. Changes in flood risk in Europe. IAHS Special Publications 10. Wallingford: IAHS Press and CRC Press/Balkema, 372–383.
- Blöschl, G. and Montanari, A., 2010. Climate change impacts—throwing the dice? Hydrological Processes, 24 (3), 374–381. doi:10.1002/hyp.7574
- Boberg, F., et al., 2009. Improved confidence in climate change projections of precipitation evaluated using daily statistics from the PRUDENCE ensemble. Climate Dynamics, 32 (7–8), 1097–1106.
- Boberg, F., et al., 2010. Improved confidence in climate change projections of precipitation further evaluated using daily statistics from the ENSEMBLES models. Climate Dynamics, 35 (7–8), 1509–1520.
- Bouwer, L.M., 2011. Have disaster losses increased due to anthropogenic climate change? Bulletin of the American Meteorological Society, 92, 39–46.
- Bouwer, L.M., 2013. Projections of future extreme weather losses under changes in climate and exposure. Risk Analysis, 33 (5), 915–930.
- Bouwer, L.M., Bubeck, P., and Aerts, J.C.J.H., 2010. Changes in future flood risk due to climate and development in a Dutch polder area. Global Environmental Change, 20, 463–471.
- Bouwer, L.M., Vermaat, J.E., and Aerts, J.C.J.H., 2008. Regional sensitivities of mean and peak river discharge to climate variability in Europe. Journal of Geophysical Research—Atmospheres, 113, D19103.
- Bouwer, L.M., et al., 2007. Confronting disaster losses. Science, 318, 753.
- xBradshaw, C.J.A., et al., 2007. Global evidence that deforestation amplifies flood risk and severity in the developing world. Global Change Biology, 13, 1–17.
- Brakenridge, G.R., et al., 2013. Global mapping of storm surges, 2002–present and the assessment of coastal vulnerability. Natural Hazards, 66 (3), 1295–1312. doi:10.1007/s11069-012-0317-z
- Brázdil, R. and Kundzewicz, Z.W., 2006. Historical hydrology—editorial. Hydrological Sciences Journal, 51 (5), 733–738.
- Brázdil, R., Kundzewicz, Z.W., and Benito, G., 2006. Historical hydrology for studying flood risk in Europe. Hydrological Sciences Journal, 51 (5), 739–764.
- Camilloni, I.A. and Barros, V.R., 2003. Extreme discharge events in the Paraná River and their climate forcing. Journal of Hydrology, 278 (1–4), 94–106.
- Cavallo, E. and Noy, I., 2009. The economics of natural disasters: a survey [online]. RES Working Papers 4649, Inter-American Development Bank, Research Department. Available from: http://ideas.repec.org/p/idb/wpaper/4649.html [Accessed 22 November 2013].
- Chang, H., Franczyk, J., and Kim, C., 2009. What is responsible for increasing flood risks? The case of Gangwon Province, Korea. Natural Hazards, 48, 399–354.
- Changnon, S., ed., 1996. The great flood of 1993. Boulder, CO: Westview Press.
- Changnon, S.A., 2001. Damaging thunderstorm activity in the United States. Bulletin of the American Meteorological Society, 82, 597–608.
- Choi, O. and Fisher, A., 2003. The impacts of socioeconomic development and climate change on severe weather catastrophe losses: Mid-Atlantic Region (MAR) and the US. Climatic Change, 58 (1–2), 149–170.
- Christensen, J.H., et al., 2007. Regional climate projections. In: S. Solomon, et al., eds. Climate change 2007: the physical science basis. Contribution of Working Group I to the Fourth Assessment Report of the Intergovernmental Panel on Climate Change. Cambridge: Cambridge University Press, 847–940.
- Ciscar, J.C., ed., 2009. Climate change impacts in Europe. Final report of the PESETA research project. EUR 24093 EN–2009. Luxembourg: European Communities.
- Conway, D., et al., 2009. Rainfall and water resources variability in sub–Saharan Africa during the twentieth century. Journal of Hydrometeorology, 10 (1), 41–59.
- Cunderlik, J.M. and Ouarda, T.B.M.J., 2009. Trends in the timing and magnitude of floods in Canada. Journal of Hydrology, 375 (3–4), 471–480.
- Curriero, F.C., et al., 2001. The association between extreme precipitation and waterborne disease outbreaks in the United States, 1948–1994. American Journal of Public Health, 91 (8), 1194–1199. doi:10.2105/AJPH.91.8.1194
- Dankers, R. and Feyen, L., 2008. Climate change impact on flood hazard in Europe: an assessment based on high resolution climate simulations. Journal of Geophysical Research—Atmospheres, 113, D19105.
- Dash, S., et al., 2007. Some evidence of climate change in twentieth-century India. Climatic Change, 85, 299–321.
- Defra (Department for Environment, Food and Rural Affairs), 2004. Scientific and technical aspects of climate change, including impacts and adaptation and associated costs. London: Defra.
- DeGaetano, A.T., 2009. Time-dependent changes in extreme-precipitation return-period amounts in the Continental United States. Journal of Applied Meteorology and Climatology, 48, 2086–2099.
- Di Baldassare, G., et al., 2010. Flood fatalities in Africa: from diagnosis to mitigation. Geophysical Research Letters, 37, L22402.
- Douglas, I., 2009. Climate change, flooding and food security in south Asia. Food Security, 1 (2), 127–136.
- Downton, M.W., Miller, J.Z.B., and Pielke, R.A., 2005. Reanalysis of US National Weather Service flood loss database. Natural Hazards Review, 6, 13–22.
- Dussaillant, A., et al., 2010. Repeated glacial–lake outburst floods in Patagonia: an increasing hazard? Natural Hazards, 54 (2), 469–481.
- Ebi, K.L., et al., 2008. Effects of global change on human health. In: J.L. Gamble, ed. Analyses of the effects of global change on human health and welfare and human systems. Report by the US Climate Change Science Program and the Subcommittee on Global Change Research. Washington, DC: US Environmental Protection Agency, 2-1–2-78.
- ECLAC, 2003. Handbook for estimating the socio-economic and environmental effects of disaster [online]. Mexico City: ECLAC, LC/MEX/G.5. Available from: http://www.eclac.org/publicaciones/xml/4/12774/lcmexg5i_VOLUME_Ia.pdf [Accessed 22 November 2013].
- EEA (European Environment Agency), 2004. Impacts of Europe’s changing climate. An indicator-based assessment. Copenhagen: EEA Report no. 2/2004, European Environment Agency.
- Engelthaler, D.M., et al., 1999. Climatic and environmental patterns associated with hantavirus pulmonary syndrome, Four Corners region, United States. Emerging Infectious Diseases, 5 (1), 87–94.
- Evans, E.R. and Ashley, G.B.O., 2004. Foresight future flooding: scientific summary. Future risks and their drivers. London: Office of Science and Technology.
- Evans, J.P. and Boyer-Souchet, I., 2012. Local sea surface temperatures add to extreme precipitation in northeast Australia during La Niña. Geophysical Research Letters, 39, L10803. doi:10.1029/2012GL052014
- Fengqing, J., et al., 2005. Magnification of flood disasters and its relation to regional precipitation and local human activities since the 1980s in Xinxiang, Northwestern China. Natural Hazards, 36, 307–330.
- Feyen, L., Barredo, J.I., and Dankers, R., 2009. Implications of global warming and urban land use change on flooding in Europe. Water and urban development paradigms—towards an integration of engineering. In: J. Feyen, K. Shannon, and M. Neville, eds. Design and management approaches. Boca Raton, FL: CRC Press, 217–225.
- Field, C.B., et al., eds., 2012. Managing the risks of extreme events and disasters to advance climate change adaptation. Special Report of Working Groups I and II of the Intergovernmental Panel on Climate Change (IPCC). Cambridge: Cambridge University Press.
- Fowler, H.J. and Wilby, R.L., 2010. Detecting changes in seasonal precipitation extremes using regional climate model projections: Implications for managing fluvial flood risk. Water Resources Research, 46, W0525.
- Gallant, A.J.E. and Karoly, D.J., 2010. A combined climate extremes index for the Australian region. Journal of Climate, 23 (23), 6153–6165.
- Gerten, D., et al., 2008. Causes of change in 20th century global river discharge. Geophysical Research Letters, 35, L20405.
- Gleason, K.L., et al., 2008. A revised U.S. climate extremes index. Journal of Climate, 21 (10), 2124–2137.
- Goode, N., et al., 2011. Review of recent Australian disaster inquiries [online]. Monash University. Available from: http://www.em.gov.au/AboutAGD/Authorityandaccountability/Committeesandcouncils/Documents/Review%20of%20Recent%20Australian%20Disaster%20Inquiries%20-%20final%20report.PDF [Accessed 15 June 2013].
- Graham, L.P., Andreasson, J., and Carlsson, B., 2007. Assessing climate change impacts on hydrology from an ensemble of regional climate models, model scales and linking methods—a case study on the Lule River basin. Climatic Change, 81 (suppl. 1), 293–307.
- Gutowski, W.J., et al., 2008. Changes in extreme, cold–season synoptic precipitation events under global warming. Geophysical Research Letters, 35, L20710.
- Haeberli, W., et al., 2001. Prevention of outburst floods from periglacial lakes at Grubengletscher, Valais, Swiss Alps. Journal of Glaciology, 47, 111–122.
- Hall, J.W., Sayers, P.B., and Dawson, R.J., 2005. National-scale assessment of current and future flood risk in England and Wales. Natural Hazards, 36 (1–2), 147–164.
- Handmer, J., 2008. Risk creation, bearing and sharing on Australian floodplains. International Journal of Water Resources Development, 24, 527–540.
- Handmer, J., Reed, C., and Percovich, O., 2002. Disaster loss assessment guidelines. Brisbane: Department of Emergency Services, State of Queensland, 117 p.
- Handmer, J., et al., 2012. Changes in impacts of climate extremes: human systems and ecosystems. In: C.B. Field, et al., eds. Managing the risks of extreme events and disasters to advance climate change adaptation. Special Report of Working Groups I and II of the Intergovernmental Panel on Climate Change (IPCC). Cambridge: Cambridge University Press, 231–290.
- Hardoy, J.E., Mitlin, D., and Satterthwaite, D., 2001. Environmental problems in an urbanizing world: finding solutions for cities in Africa, Asia and Latin America. London: Earthscan.
- Hashizume, M., et al., 2008. Factors determining vulnerability to diarrhoea during and after severe floods in Bangladesh. Journal of Water Health, 6 (3), 323–332.
- Hattermann, F.F., et al., 2013. Climatological drivers of changes in flood hazard in Germany. Acta Geophysica, 61 (2), 463–477.
- Hennessy, K., et al., 2007. Australia and New Zealand. In: M.L. Parry, et al., eds. Climate change 2007: impacts, adaptation and vulnerability. Contribution of Working Group II to the Fourth Assessment Report of the Intergovernmental Panel on Climate Change. Cambridge: Cambridge University Press, 507–540.
- Hilker, N., Badoux, A., and Hegg, C., 2009. The Swiss flood and landslide damage database 1972–2007. Natural Hazards Earth System Sciences, 9, 913–925.
- Hirabayashi, Y., et al., 2008. Global projections of changing risks of floods and droughts in a changing climate. Hydrological Sciences Journal, 53 (4), 754–772.
- Hirabayashi, Y., et al., 2013. Global flood risk under climate change. Nature Climate Change, 3, 816–821. doi:10.1038/nclimate1911
- Hirabayashi, Y. and Kanae, S., 2009. First estimate of the future global population at risk of flooding. Hydrology Research Letters, 3, 6–9.
- Hirsch, R.M. and Ryberg, K.R., 2012. Has the magnitude of floods across the USA changed with global CO2 levels? Hydrological Sciences Journal, 57 (1), 1–9.
- Hjelle, B. and Glass, G.E., 2000. Outbreak of hantavirus Infection in the Four Corners Region of the United States in the Wake of the 1997–1998 El Niño–Southern Oscillation. The Journal of Infectious Diseases, 181 (5), 1569–1573.
- Hoes, O.A.C., 2007. Aanpak wateroverlast in polders op basis van risicobeheer. Thesis (PhD), Delft University of Technology, (in Dutch). Available from: http://repository.tudelft.nl/assets/uuid:69de94f9–385c–4c50–beff–8cc7b955beba/ceg_hoes_20070319.pdf
- xHuggel, C., et al., 2004. An assessment procedure for glacial hazards in the SwissAlps. Canadian Geotechnology Journal, 41, 1068–1083.
- Ives, J.D., Shrestha, R.B., and Mool, P.K., 2010. Formation of glacial lakes in the Hindu Kush–Himalayas and GLOF Risk Assessment. Kathmandu: ICIMOD.
- IWR (Institute for Water Resources, US Army Corps of Engineers), 2011. Flood risk management approaches as being practiced in Japan, Netherlands, United Kingdom, and United States. IWR Report no. 2011–R–08, 124 pp.
- Jongman, B., Ward, P.J., and Aerts, J.C.J.H., 2012. Global exposure to river and coastal flooding: long term trends and changes. Global Environmental Change, 22 (4), 823–835.
- Kamiguchi, K., et al., 2006. Changes in precipitation–based extremes indices due to global warming projected by a global 20-km-mesh atmospheric model. SOLA, 2, 64–67.
- Kharin, V., et al., 2007. Changes in temperature and precipitation extremes in the IPCC ensemble of global coupled model simulations. Journal of Climate, 20 (8), 1419–1444.
- Kleinen, T. and Petschel-Held, G., 2007. Integrated assessment of changes in flooding probabilities due to climate change. Climatic Change, 81 (3), 283–312.
- Koutsoyiannis, D., 2011. Hurst-Kolmogorov dynamics and uncertainty. Journal of the American Water Resources Association, 47 (3), 481–495.
- Koutsoyiannis, D., 2012. Reply to the Comment by T. López-Arias on “Clausius-Clapeyron equation and saturation vapour pressure: simple theory reconciled with practice”. European Journal of Physics, 33, L13–L14. doi:http://dx.doi.org/10.1088/0143-0807/33/3/L13
- Koutsoyiannis, D., 2013. Hydrology and change. Hydrological Sciences Journal, 58 (6), 1177–1197. doi:http://dx.doi.org/10.1080/02626667.2013.804626
- Koutsoyiannis, D., et al., 2008. On the credibility of climate predictions. Hydrological Sciences Journal, 53 (4), 671–684.
- Koutsoyiannis, D., et al., 2011. Scientific dialogue on climate: is it giving black eyes or opening closed eyes? Reply to “A black eye for the Hydrological Sciences Journal” by D. Huard. Hydrological Sciences Journal, 56 (7), 1334–1339.
- Koutsoyiannis, D. and Montanari, A., 2007. Statistical analysis of hydroclimatic time series: uncertainty and insights. Water Resources Research, 43 (5), W05429.
- Kovats, R.S. and Akhtar, R., 2008. Climate, climate change and human health in Asian cities. Environment and Urbanization, 20 (1), 165–175. doi:10.1177/0956247808089154
- Kron, W., et al., 2012. How to deal properly with a natural catastrophe database—analysis of flood losses. Natural Hazards and Earth System Sciences, 12, 535–550.
- Kshirsagar, N., Shinde, R., and Mehta, S., 2006. Floods in Mumbai: impact of public health service by hospital staff and medical students. Journal of Postgraduate Medicine, 52 (4), 312.
- Kundzewicz, Z.W., 2011. Nonstationarity in water resources—Central European perspective. Journal of the American Water Resources Association (JAWRA), 47 (3), 550–562. doi:10.1111/j.1752-1688.2011.00549.x
- Kundzewicz, Z.W., ed., 2012. Changes in flood risk in Europe. Wallingford: IAHS Press.
- Kundzewicz, Z.W., 2013. Floods: lessons about early warning systems. In: EEA (European Environment Agency), ed. Late lessons from early warnings: science, precaution, innovation. EEA Report no. 1/2013. Copenhagen: European Environment Agency, 347–368.
- Kundzewicz, Z.W., Pińskwar, I., and Brakenridge, G.R., 2013. Large floods in Europe, 1985–2009. Hydrological Sciences Journal, 58 (1), 1–7.
- Kundzewicz, Z.W. and Schellnhuber, H.-J., 2004. Floods in the IPCC TAR perspective. Natural Hazards, 31, 111–128.
- Kundzewicz, Z.W. and Stakhiv, E.Z., 2010. Are climate models “ready for prime time” in water resources management applications, or is more research needed? Editorial. Hydrological Sciences Journal, 55 (7), 1085–1089.
- Kundzewicz, Z.W., et al., 2005. Trend detection in river flow time-series: 1. Annual maximum flow. Hydrological Sciences Journal, 50 (5), 797–810.
- Kundzewicz, Z.W., et al., 2007. Freshwater resources and their management. In: M.L. Parry, et al., eds. Climate change 2007: impacts, adaptation and vulnerability. Contribution of Working Group II to the Fourth Assessment Report of the Intergovernmental Panel on Climate Change. Cambridge: Cambridge University Press.
- Kundzewicz, Z.W., et al., 2008. The implications of projected climate change for freshwater resources and their management. Hydrological Sciences Journal, 53 (1), 3–10.
- Kundzewicz, Z.W., et al., 2012. Flood risk in Europe—setting the stage. In: Z.W. Kundzewicz, ed. Changes in flood risk in Europe. Wallingford: IAHS Press, 11–26.
- Kunkel, K.E., et al., 2008. Observed changes in weather and climate extremes. In: T.R. Karl, et al., eds. Weather and climate extremes in a changing climate. Regions of focus: North America, Hawaii, Caribbean, and US Pacific Islands. Report by the US Climate Change Science Program and the Subcommittee on Global Change Research, Washington, DC, 222.
- Leander, R., et al., 2008. Estimated changes in flood quantiles of the river Meuse from resampling of regional climate model output. Journal of Hydrology, 351 (3–4), 331–343.
- Lehner, B., et al., 2006. Estimating the impact of global change on flood and drought risks in Europe: a continental, integrated analysis. Climatic Change, 75 (3), 273–299.
- Liepert, B.G. and Previdi, M., 2012. Inter-model variability and biases of the global water cycle in CMIP3 coupled climate models. Environmental Research Letters, 7. doi:10.1088/1748–9326/7/1/014006
- Lins, H.F. and Slack, J.R., 1999. Streamflow trends in the United States. Geophysical Research Letters, 26 (2), 227–230.
- Lugeri, N., et al., 2010. River flood risk and adaptation in Europe—assessment of the present status. Mitigation and Adaptation Strategies for Global Change, 15 (7), 621–639.
- Lyon, B., 2003. Enhanced seasonal rainfall in northern Venezuela and the extreme events of December 1999. Journal of Climate, 16, 2302–2306.
- Maaskant, B., Jonkman, S.N., and Bouwer, L.M., 2009. Future risk of flooding: an analysis of changes in potential loss of life in South Holland (The Netherlands). Environmental Science and Policy, 12, 157–169.
- Marengo, J., et al., 2012. Extreme climatic events in the Amazon basin. Theoretical and Applied Climatology, 107, 73–85. doi:10.1007/s00704-011-0465-1
- Marengo, J.A., et al., 2009. Future change of temperature and precipitation extremes in South America as derived from the PRECIS regional climate modelling system. International Journal of Climatology, 29 (15), 2241–2255.
- Mastrandrea, M.D., et al., 2010. Guidance note for lead authors of the IPCC Fifth Assessment Report on consistent treatment of uncertainties [online]. Intergovernmental Panel on Climate Change (IPCC). Available from: http://www.ipcc.ch [Accessed 18 October 2012].
- McCabe, G.J. and Wolock, D.M., 2002. A step increase in streamflow in the conterminous United States. Geophysical Research Letters, 29 (24), 2185.
- McMichael, A.J., Wooddruff, R.E., and Hales, S., 2006. Climate change and human health: present and future risks. Lancet, 367, 859–869.
- Meyer, V., et al., 2013. Assessing the costs of natural hazards: state of the art and knowledge gaps. Natural Hazards and Earth System Sciences, 13, 1351–1373.
- Miller, S., Muir-Wood, R., and Boissonnade, A., 2008. An exploration of trends in normalised weather–related catastrophe losses. In: H.F. Diaz and R.J. Murnane, eds. Climate extremes and society. Cambridge: Cambridge University Press, 225–247.
- Min, S.-K., et al., 2011. Human contribution to more intense precipitation extremes. Nature, 470 (7334), 378–381.
- Min, S.-K., Zhang, X., and Zwiers, F., 2008. Human-induced arctic moistening. Science, 320 (5875), 518–520.
- Ministry of Environment and Forest, 2005. National Adaptation Programme of Action (NAPA), Final Report. Dhaka: Government of the People’s Republic of Bangladesh.
- Moberg, A., et al., 2006. Indices for daily temperature and precipitation extremes in Europe analyzed for the period 1901–2000. Journal of Geophysical Research—Atmospheres, 111(D22), D22106.
- Montanari, A., et al., 2013. “Panta Rhei—everything flows”: change in hydrology and society—the IAHS Scientific Decade 2013–2022. Hydrological Sciences Journal, 58 (6), 1256–1275. doi:http://dx.doi.org/10.1080/02626667.2013.809088
- Morin, E., et al., 2009. Flood routing and alluvial aquifer recharge along the ephemeral arid Kuiseb River, Namibia. Journal of Hydrology, 368, 262–275.
- Mullan, B., et al., 2008. Climate change effects and impacts assessment: a guidance manual for local government in New Zealand. Wellington: Ministry for the Environment.
- Neumayer, E. and Barthel, F., 2011. Normalizing economic loss from natural disasters: a global analysis. Global Environmental Change, 21, 13–24.
- Nicholls, N. and Seneviratne, S.I., 2013. Comparing IPCC Assessments: how do the AR4 and SREX assessments of changes in extremes differ? Climatic Change. doi:10.1007/S10854-013-018-0
- OCDESC (Official Committee for Domestic and External Security Coordination), 2007. National hazardscape report. Wellington: Official Committee for Domestic and External Security Coordination, Department of the Prime Minister and Cabinet.
- Oliveira, P.J.C., et al., 2011. Vegetation-mediated impacts of trends in global radiation on land hydrology: a global sensitivity study. Global Change Biology, 17, 3453–3467.
- Osman-Elasha, B., et al., 2006. Impacts, vulnerability and adaptation to climate change in Africa. Background paper for the African Workshop on Adaptation Implementation of Decision 1/CP.10 of the UNFCCC Convention Accra, 21–23 September, Ghana.
- Overeem, I. and Syvitski, J.P.M., 2010. Shifting discharge peaks in Arctic Rivers, 1997–2007. Geografiska Annaler, 92 (2), 285–296.
- Pall, P., et al., 2011. Anthropogenic greenhouse gas contribution to flood risk in England and Wales in autumn 2000. Nature, 470 (7334), 382–385. doi:10.1038/nature09762
- Parmenter, R., et al., 1999. Incidence of plague associated with increased winter–spring precipitation in New Mexico. American Journal of Tropical Medicine and Hygiene, 61 (5), 814–821.
- Parry, M., et al., 2009. Assessing the costs of adaptation to climate change: a review of the UNFCCC and other recent estimates. London: International Institute for Environment and Development and Grantham Institute for Climate Change.
- Peduzzi, P., et al., 2009. Assessing global exposure and vulnerability towards natural hazards: the Disaster Risk Index. Natural Hazards and Earth System Sciences, 9, 1149–1159.
- Peduzzi, P., et al., 2011. Preview Global Risk Data Platform, [online], UNEP/GRID Geneva, UNISDR, World Bank. Available from: http://preview.grid.unep.ch/index.php?preview=tools&cat=1&lang=eng [Accessed 18 October 2012].
- Peduzzi, P., et al., 2012. Global trends in tropical cyclones risk. Nature Climate Change, 2 (4), 289–294.
- Peterson, T.C., et al., 2008. Why weather and climate extremes matter. In: T.R. Karl, et al., eds. Weather and climate extremes in a changing climate. Regions of focus: North America, Hawaii, Caribbean, and US Pacific Islands. Washington, DC: US Climate Change Science Program.
- Pielke Jr., R.A., et al., 2005. Clarifying the attribution of recent disaster losses: a response to Epstein and McCarthy. Bulletin of the American Meteorological Society, 86, 1481–1483.
- Pielke Jr., R.A. and Downton, M.W., 2000. Precipitation and damaging floods: trends in the United States, 1932–1997. Journal of Climate, 13 (20), 3625–3637.
- Pitman, A.J., et al., 2009. Uncertainties in climate responses to past land cover change: first results from the LUCID intercomparison study. Geophysical Research Letters, 36 (14), L14814.
- Pomeranets, K.S., 2005. Three centuries of floods in St Petersburg. St Petersburg: Iskusstvo.
- Pryor, S.C., et al., 2009. How spatially coherent and statistically robust are temporal changes in extreme precipitation in the contiguous USA? International Journal of Climatology, 29 (1), 31–45.
- Ramsbottom, D., Sayers, P., and Panzeri, M., 2012. Climate change risk assessment for the floods and coastal erosion sector [online]. UK Climate Change Risk Assessment. Defra, London. Available from: http://www.defra.gov.uk/environment/climate/government/risk-assessment/#sectors [Accessed 15 June 2013].
- Ranger, N., et al., 2011. An assessment of the potential impact of climate change on flood risk in Mumbai. Climatic Change, 104, 139–167.
- Richardson, S.D. and Reynolds, J.M., 2000. An overview of glacial hazards in the Himalayas. Quaternary International, 65, 31–47.
- Rico, M., et al., 2008. Reported tailings dam failures. A review of the European incidents in the worldwide context. Journal of Hazardous Materials, 152, 846–852.
- Rodier, J.A. and Roche, M., 1984. World catalogue of maximum observed floods. IAHS Publication No. 143. Wallingford: IAHS Press.
- Rosenzweig, C., et al., 2007. Assessment of observed changes and responses in natural and managed systems. In: M.L. Parry, et al., eds. Climate change 2007: impacts, adaptation and vulnerability. Contribution of Working Group II to the Fourth Assessment Report of the Intergovernmental Panel on Climate Change. Cambridge: Cambridge University Press.
- Saenz, R., et al., 1995. Post-disaster malaria in Costa Rica. Prehospital Disaster Medicine, 10 (3), 154–160.
- Savelieva, N.I., et al., 2004. Climate change in the northern Asia in the second half of the 20th century. Pacific Oceanography, 2 (1–2), 74–84.
- Schneider, F., et al., 2010. Shadow economies all over the world: New estimates for 162 countries from 1999 to 2007 [online]. The World Bank Development Research Group, Poverty and Inequality Team & Europe and Central Asia Region, Human Development Economics Unit. Available from: http://wwwwds.worldbank.org/external/default/WDSContentServer/IW3P/IB/2010/10/14/000158349_20101014160704/Rendered/PDF/WPS5356.pdf [Accessed 18 October 2012].
- Semyonov, V.A. and Korshunov, A.A., 2006. Floods on the Russian rivers in the late 20th century and the early 21st century. Issues of Geography and Geo-ecology, 5, 6–12.
- Seneviratne, S.I., et al., 2012. Changes in climate extremes and their impacts on the natural physical environment. In: C.B. Field, et al., eds. Managing the risks of extreme events and disasters to advance climate change adaptation. Special Report of Working Groups I and II of the Intergovernmental Panel on Climate Change (IPCC). Cambridge: Cambridge University Press.
- Shankman, D., et al., 2006. Flood frequency in China’s Poyang Lake Region: trends and teleconnections. International Journal of Climatology, 26, 1255–1266.
- Shen, C., et al., 2008. Characteristics of anomalous precipitation events over eastern China during the past five centuries. Climate Dynamics, 31 (4), 463–476.
- Shiklomanov, A.I., et al., 2007. Temporal and spatial variations in maximum river discharge from a new Russian data set. Journal of Geophysical Research—Biogeosciences, 112, G04S53. doi:10.1029/2006JG000352
- Stephens, G.L., et al., 2010. Dreary state of precipitation in global models. Journal of Geophysical Research, 115, D24211. doi:10.1029/2010JD014532
- Syvitski, J.P.M. and Brakenridge, G.R., 2013. Causation and avoidance of catastrophic flooding along the Indus River, Pakistan[online]. GSA Today, 23 (1). doi: 10.1130/GSATG165A.1. Available from: http://www.geosociety.org/gsatoday/archive/23/1/article/i1052-5173-23-1-4.htm [Accessed 22 November 2013].
- Taye, M.T., et al., 2011. Assessment of climate change impact on hydrological extremes in two source regions of the Nile River Basin. Hydrology and Earth System Sciences, 15 (1), 209–222.
- Te Linde, A.H., et al., 2011. Future flood risk estimates along the river Rhine. Natural Hazards Earth System Sciences, 11, 459–473.
- Teuling, A.J., et al., 2010. Contrasting response of European forest and grassland energy exchange to heatwaves. Nature Geoscience, 3, 722–727.
- Thibault, K.M. and Brown, J.H., 2008. Impact of an extreme climatic event on community assembly. PNAS, 105 (9), 3410–3415.
- Trenberth, K.E., 2012. Framing the way to relate climate extremes to climate change. Climatic change [online]. Available from: http://www.cgd.ucar.edu/cas/Trenberth/trenberth.papers/Framing_ClimCh12.pdf [Accessed 18 October 2012].
- Trenberth, K.E., et al., 2007. Observations: surface and atmospheric climate change. In: S. Solomon, et al., eds. Climate change 2007: the physical science basis. Contribution of Working Group I to the Fourth Assessment Report of the Intergovernmental Panel on Climate Change. Cambridge: Cambridge University Press.
- UNISDR (United Nations International Strategy for Disaster Reduction), 2011. Revealing risk, redefining development. Geneva: UNISDR.
- Villarini, G.F., et al., 2009. On the stationarity of annual flood peaks in the continental United States during the 20th century. Water Resources Research, 45, W08417. doi:10.1029/2008WR007645
- Vörösmarty, C.J., 2002. Global change, the water cycle, and our search for Mauna Loa. Hydrological Processes, 16 (1), 135–139. doi:10.1002/hyp.527
- Wang, J. and Zhang, X., 2008. Downscaling and projection of winter extreme daily precipitation over North America. Journal of Climate, 21 (5), 923–937.
- Wells, L.E., 1990. Holocene history of the El Niño phenomenon as recorded in flood sediments of northern coastal Peru. Geology, 18 (11), 1134–1137. doi:10.1130/0091-7613
- Wenger, C., Hussey, K., and Pittock, J., 2013. Living with floods: key lessons from Australia and abroad [online]. Australian National University and NCCARF. Available from: http://www.nccarf.edu.au/publications/living-floods-key-lessons-australia-and-abroad [Accessed 22 November 2013].
- Wilby, R.L., et al., 2008. Climate change and fluvial flood risk in the UK: more of the same? Hydrological Processes, 22 (14), 2511–2523.
- Wilby, R.L. and Keenan, R., 2012. Adapting to flood risk under climate change. Progress in Physical Geography, 36, 349–379.
- World Bank, 2010. Economics of adaptation to climate change [online]. Washington, DC: World Bank. Available from: http://www-wds.worldbank.org/external/default/WDSContentServer/WDSP/IB/2012/06/27/000425970_20120627163039/Rendered/PDF/702670ESW0P10800EACCSynthesisReport.pdf [Accessed 22 November 2013].
- Wu, X., et al., 2012. An evaluation of land surface modification, storm sewer development, and rainfall variation on waterlogging risk in Shanghai. Natural Hazards, 63, 305–323.
- Zhang, X., et al., 2007. Detection of human influence on twentieth-century precipitation trends. Nature, 448 (7152), 461–465.
- Zolina, O.C., et al., 2010. Changing structure of European precipitation: longer wet periods leading to more abundant rainfalls. Geophysical Research Letters, 37, L06704.