Abstract
Editor D. Koutsoyiannis
INTRODUCTION
The Working Group II contribution to the Fifth Assessment Report of the Intergovernmental Panel on Climate Change (IPCC WG II AR5, cf. Field et al. Citation2014) critically reviewed tens of thousands of recent publications to assess current scientific knowledge on climate change impacts, vulnerability and adaptation. Chapter 3 of the report focuses on freshwater resources, but water issues are also prominent in other sectoral chapters and in the regional chapters of the Working Group II report, as well as in various chapters of the Working Group I contribution (IPCC WG I AR5, cf. Stocker et al. Citation2013). With this paper, the lead authors, a review editor and the chapter scientist of the freshwater chapter of the WG II AR5 (Jiménez et al. Citation2014) wish to summarize their assessment of the most relevant risks of climate change related to freshwater systems and to show how assessment and reduction of those risks can be integrated into water management.
THE CONCEPT OF RISK IS WELL SUITED FOR INTEGRATING CLIMATE CHANGE ASPECTS INTO WATER MANAGEMENT
To better support decision-making in the context of climate change, WG II AR5 has adopted a new focus on risks, developing further the concept of risk elaborated in the SREX report (Field et al. Citation2012). Risk can be understood, in agreement with the meaning of the term used in IPCC WGII AR5, as the potential for consequences where something of value is at stake and where the outcome is uncertain. The term “risk” is mostly used when referring to the potential of negative consequences. The risk that a certain impact (adverse consequence for a natural or human system) of climate change occurs results from the interaction of hazards (potentially occurring physical events or trends as affected by climate change), exposure (presence of people, ecosystems and assets in places and settings that could be adversely affected by the hazards) and vulnerability (predisposition to be adversely affected) (). Risk is often estimated as the probability of occurrence of hazardous events or trends multiplied by the impacts that ensue if these events or trends do occur (IPCC Citation2014).
Fig. 1 Illustration of the core concepts of WGII AR5 (in lower, blue part of figure, Fig. SPM.1 in IPCC Citation2014). The risk that a certain impact of climate change occurs results from the interaction of climate-related hazards as impacted on by climate change (including hazardous events and trends) with the vulnerability and exposure of natural and human systems. Changes in both the climate system (left) and socio-economic processes, including adaptation and mitigation (right), are drivers of hazards, exposure and vulnerability. At the top of the figure, risk management approaches that are suitable for water management are indicated. Freshwater-related risks can be addressed by an iterative risk-management approach (“adaptive water management”) that is based on risk assessment. They can be reduced by climate change (CC) mitigation, i.e. reduction of greenhouse gas (GHG) emissions, by adaptation to climate change and by reducing vulnerability and exposure to hazards.
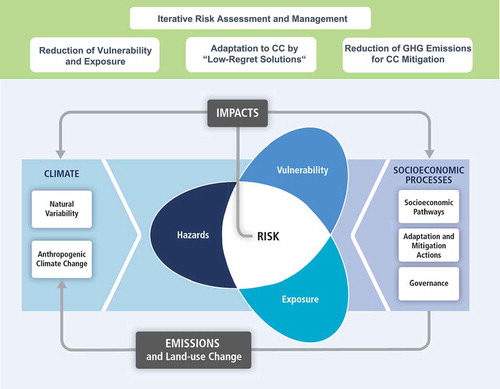
Risks associated with climate change are not caused by anthropogenic climate change alone but also by climate variability and by socio-economic conditions and processes (). Introducing the concept of risk puts climate change risk at par with other global risks, such as loss of biodiversity, environmental pollution, pandemic, famine, economic depression, terrorism and war. It implies that climate change is not the only risk; nonetheless, it is a significant risk.
In water management, probabilistic assessments of hazards and risks are routinely done because of the stochastic nature of weather. Elaboration of flood hazard and risk maps, for example, are required by the European Union floods directive (EU Citation2007). Stochastic variability of streamflow is taken into account in the design of water supply systems. A similar approach is used for producing drinking water from raw water of variable quality. Traditionally, the probability of a certain hazard and thus risk was computed from historic observations of streamflow, conveniently assuming that weather variables and thus climate-related hazards varied with constant variance around a constant mean. This approach is no longer practicable because, due to anthropogenic climate change, climate cannot be regarded as stationary (Milly et al. Citation2008). Climate is now subject to spatially heterogeneous trends in both mean behaviour and variability. As these trends are highly uncertain (like the weather), a risk approach is also appropriate for climate change assessments.
A RISK ASSESSMENT APPROACH IS BEST FOR HANDLING THE UNCERTAINTY OF FUTURE CLIMATE CHANGE AND ITS IMPACTS
To support water management under changing climate, it is useful to evaluate potential freshwater-related impacts of climate change in a quantitative way. This involves the application of a chain of models, the output of which is subject to significant uncertainty. Uncertainty stems from: (1) scenarios of future greenhouse gas emissions by integrated assessment models; (2) the translation of greenhouse gas emissions scenarios into atmospheric concentrations and forcings; (3) evaluation of the effects of these forcings on climate by global climate models (GCMs); (4) downscaling and bias-correcting the output of the GCMs; and (5) translation of climate change projections into impact projections by impact models, e.g. hydrological or vegetation models. An additional uncertainty in computing freshwater-related hazards is related to scarce information about the current or reference state of the freshwater system under consideration. It has been shown that climate models, downscaling/bias-correction methods and hydrological models contribute comparable amounts of uncertainty to impact assessments (Quintana Segui et al. Citation2010, Chen et al. Citation2011, Hagemann et al. Citation2013, Schewe et al. Citation2014). Uncertainty regarding future greenhouse gas emissions only becomes important in the second half of the 21st century because near-term climate is strongly conditioned by past greenhouse gas emissions, and emissions scenarios differ more strongly after 2050 (IPCC Citation2013). Finally, uncertainty regarding future socio-economic conditions, affecting exposure and vulnerability and thus risks of climate change (), is at least as large as the climate-related uncertainty.
Due to these uncertainties, the impacts of climate change on freshwater systems, even under a certain emissions scenario, cannot be quantified in a deterministic way; we can only aim at providing a range of plausible projections. Therefore, water managers should no longer base their decisions on deterministic estimates of future hydrological conditions and their impacts but consider instead future freshwater hazards and risks. This means that a broad range of possible future hydrological changes should be considered for managing water under climate change, taking into account a number of emissions and socio-economic scenarios. Due to the uncertainties described above, potential future hydrological changes become hazards () that are (ideally) described by their probability of occurrence. Freshwater-related risks of climate change are then derived by assessing the consequences that would result if the hazard realizes, considering exposure and vulnerability ().
The state-of-the-art approach for dealing with climate model and impact model uncertainties is to perform multi-model studies, where the output of several climate models is used as input to one or, better, to several hydrological models (e.g. Crosbie et al. Citation2013, Davie et al. Citation2013, Dankers et al. Citation2014, Schewe et al. Citation2014) to produce an ensemble of potential changes in risk. As an example, shows the potential changes of mean annual streamflow under climate change that was estimated in a multi-model study on future water scarcity (Schewe et al. Citation2014); in this figure, colours indicate the multi-model ensemble mean, while model-related uncertainty is represented by the saturation of the colours. The characteristics of the ensemble and the range in estimated risks depend on which scenarios and models are incorporated and how—or whether—they are weighted. Multi-model studies make the debatable but (at least at present) necessary assumption that each combination of climate-model and hydrological-model runs should be given the same weight. In this way, a probability distribution of possible impacts of climate change (i.e. a probabilistic description of climate change-driven hazards or risks under a given emissions scenario) can be estimated. Given the uncertainties of the models, the resulting probability distribution is again uncertain.
Fig. 2 Percentage change of mean annual streamflow for a global mean temperature rise of 2°C above 1980–2010 (2.7°C above pre-industrial). Colour hues show the multi-model mean change across five GCMs and 11 global hydrological models (GHMs), and saturation shows the agreement on the sign of change across all 55 GHM-GCM combinations (percentage of model runs agreeing on the sign of change) (from Jiménez et al. Citation2014, based on Schewe et al. Citation2014).
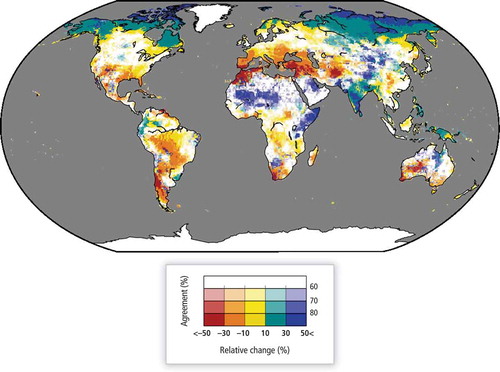
According to IPCC (Citation2014, p. 9), “assessment of the widest possible range of potential impacts, including low-probability outcomes with large consequences, is central to understanding the benefits and trade-offs of alternative risk management actions”. Therefore, not only multi-model ensemble means should be analysed but also less likely outcomes with a high risk, i.e. outcomes that may have strong negative impacts due to high exposure and vulnerability (). An example of support for water management under the new risk concept is a series of maps of probabilities for certain changes of renewable groundwater resources across the Australian continent under different future global warming scenarios; the maps were derived from an ensemble of potential future groundwater resources as quantified by a multi-model study (Crosbie et al. Citation2013). Ensembles could also be used to inform stakeholders with different “safety requirements”; for example, in a case where a particular high-flow event would put the production of safe drinking water at risk and require investment in additional treatment options, stakeholders with a high safety requirement may decide in favour of the investment even if only 10% of the ensemble runs project such a high-flow event over the design period, while stakeholders with a low safety requirement may only invest if at least 50% of the runs do this.
The current generation of hydrological models generally does not take into account that vegetation changes in response to anthropogenic climate change. Increased atmospheric carbon dioxide concentrations lead to educed transpiration, which should lead to increased runoff generation (physiological effect, Gerten et al. Citation2014). At the same time, they may also lead to increased biomass and leaf area, which would increase transpiration and canopy evaporation (structural effect). In addition, vegetation reacts directly to climatic changes that may even lead to biome shifts in catchments e.g. from grassland to forest. The net effects on water resources and irrigation requirements of changes in vegetation due to both increasing carbon dioxide concentrations and climate change can be significant but remain highly uncertain (Davie et al. Citation2013, Gerten et al. Citation2014). Nevertheless, future studies on freshwater-related risks at all spatial scales should consider the active role of vegetation in altering water flows under climate change.
CLIMATE VARIABILITY INCREASES SPATIALLY AND TEMPORALLY UNDER CLIMATE CHANGE, WITH DRY REGIONS BECOMING EVEN DRIER, AND FLOOD AND DROUGHT HAZARDS INCREASING IN MANY LOCATIONS
There is high confidence that climate change reduces renewable surface water and groundwater resources significantly in most regions with dry and mediterranean subtropical climates (). This is expected to exacerbate competition among the water users and sectors, i.e. agriculture, ecosystems, settlements, industry and energy producers. In contrast, renewable water resources (defined as long-term average annual runoff or streamflow) are likely to increase at high latitudes, as well as in some currently water-stressed areas of India and China. However, increases in annual runoff or streamflow (as shown in ) may not indicate reduced water stress if they are caused by increases during the wet (monsoon) season, or if no infrastructure is available to capture the additional volume of water. Moreover, increased annual streamflow may indicate exacerbated flood risk. Over the next few decades and for increases in global mean temperature of less than around 2°C above the pre-industrial level, changes in population may alter resource availability more than climate change.
Anthropogenic climate change implies more variable climate and therefore more variable surface water flows. It is likely that the frequency of meteorological droughts (less rainfall) and agricultural droughts (less soil moisture) in presently dry regions will increase by the end of the 21st century under the high-emissions RCP8.5 scenario (for a description of the four scenarios used in IPCC AR5, refer to IPCC Citation2013). Climate change is also likely to increase the frequency of short hydrological droughts (less surface water and groundwater) in these regions. Floods caused by snowmelt are expected to decrease in a warmer climate. However, the hazard due to moraine-dammed glacier-marginal lakes will probably continue to increase. Many such lakes are growing, but the low frequency of dam failures makes it difficult to identify and impractical to project changes in the failure rate. Changes in the probability of rainfall-driven flood events are very difficult to project. In general, there is only low to medium confidence regarding quantitative changes in future drought and flood hazards because model-based projection of future climatic and hydrological extremes is more difficult and uncertain than the projection of mean conditions. However, there is high confidence that heavy rainfall events will become more intense and frequent during the 21st century, except in areas with strongly reduced total precipitation (Field et al. Citation2012).
Coastal flooding, as well as salt water intrusion into coastal aquifers due to sea-level rise and storm surges, pose risks for water supply in cases of unconfined aquifers in low-lying areas such as coral islands and deltas. Soil erosion and sediment load are expected to increase in areas with increased heavy rainfall and in areas affected by losses of ice cover, seasonal snow cover and permafrost; the extent of these changes is highly uncertain.
DECREASING STORAGE OF FRESHWATER AS GLACIER ICE OR SNOW AFFECTS SEASONAL AND ANNUAL STREAMFLOWS
There is high confidence that in regions with snowfall and glaciers, already observed impacts of climate change will become stronger with continuing climate change. Warming causes increased winter flows, earlier and smaller meltwater-driven streamflows and reduced summer flows (except under monsoonal climate), affecting, for example, water supply and freshwater habitats. In glacier-fed rivers, total meltwater yields from stored glacier ice will increase in many regions during the next decades, but decrease thereafter as glaciers become smaller and smaller and finally disappear.
ALL WATER USES AND USERS ARE AFFECTED BY CLIMATE CHANGE BUT IN DIFFERENT WAYS
Irrigated agriculture, by far the largest water-use sector globally, is not only affected by changes in water availability that are caused by climate change. Climate change also alters irrigation water demand (). Higher temperatures and more variable rainfall tend to increase water demand per unit of irrigated area, unless total rainfall increases sufficiently in compensation. However, water demand to produce a given amount of food, feed or fiber will increase less (or decrease more) than demand per unit of irrigated area, as crop water productivity increases due to higher carbon dioxide concentrations (Gerten et al. Citation2014).
Fig. 3 The water–energy–food nexus as related to climate change. The interlinkages of supply/demand, quantity and quality of water, energy and food/feed/fiber with changing climatic conditions have implications for both adaptation and mitigation strategies in water management too (from Arent et al. Citation2014).
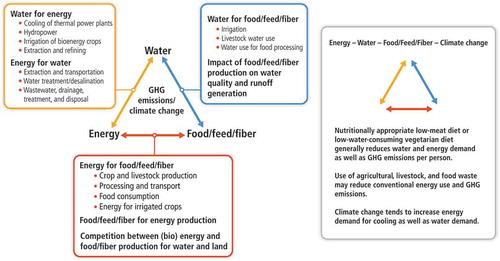
Regarding municipal water services, climate change is expected to reduce raw water quality and pose risks to drinking water quality. However, water quality projections are very difficult. Climate change-related reasons for reduced raw water quality include: (1) increased water temperature; (2) increased sediment, nutrient, and pollutant loadings from heavy rainfall; and (3) increased concentration of pollutants during low-flow periods. During floods, operation of municipal water treatment plants may be disrupted, such that the supply of safe drinking water cannot be assured. Detection of water quality changes is constrained by the fact that only a limited number of water quality variables are monitored; therefore, the final product—drinking water—may still comply with the targeted standard while posing an undetected risk to consumers.
In the energy sector, hydro-electric and thermal power production require large amounts of water () and are therefore affected by changing streamflows. Changing seasonality in snow-dominated basins can support increased hydropower production in winter but lead to decreased production in the summer. In regions with high electricity demands for heating and relatively low demands for cooling, this makes the annual hydrograph more similar to seasonal variations in electricity demand, reducing required reservoir capacities and providing opportunities for operating dams and power stations to the benefit of riverine ecosystems. In general, climate change requires adaptation of operating rules, but existing reservoir capacity may constrain adaptation where inflows become more variable. Regarding thermal power plants, the number of days with a reduced useable capacity is projected to increase due to rising stream temperatures and prolonged low flows. Warmer cooling water lowers thermal power plant efficiency.
Climate change is an additional stressor of freshwater ecosystems. It affects them not only by increasing water temperature and changing water quality, but also by altering ecologically important characteristics of hydrologic regimes in rivers and wetlands, e.g. low- and high-flow magnitudes. Of particular concern are wetlands in dry environments which are hotspots of biodiversity and are at a high risk of drying out. Freshwater ecosystems are also negatively affected by human adaptation to climate change-induced increases in flood risk if adaptation occurs by construction of dykes and dams. Except in areas with intensive irrigation, the streamflow-mediated ecological impacts of climate change are expected to be stronger than historical impacts, owing to anthropogenic alteration of flow regimes by water withdrawals and the construction of reservoirs. However, very little is known about specific impacts of hydrologic alterations on species composition or population numbers (Döll and Bunn Citation2014). Among the different water users, ecosystems may suffer most from climate change as they often have the lowest adaptation capacity, and water is generally allocated to human water users first.
CLIMATE CHANGE-RELATED RISKS IN THE WATER SECTOR WILL INCREASE FOR ALL PLAUSIBLE EMISSIONS SCENARIOS, AND MORE STRONGLY IN THE CASE OF HIGH GREENHOUSE GAS EMISSIONS
Modelling studies since the Fourth Assessment Report of the IPCC (cf. Kundzewicz et al. Citation2008, Citation2009, Koutsoyiannis et al. Citation2009), with large but better quantified uncertainties, have demonstrated clear differences between futures with higher emissions and those with lower emissions, which cause less damage and cost less to adapt to (see Table 3-2 in Jiménez et al. Citation2014), particularly further into the second half of the 21st century (Arnell and Lloyd-Hughes Citation2014). For example, the fraction of global population experiencing water scarcity and the fraction affected by major river floods are projected to increase with the amount of global warming in the 21st century. A multi-model study estimated that for each degree of global warming, approximately 7% of the global population will be exposed to a decrease in renewable water resources of at least 20% (up to 2°C of global warming from 1980 to 2010, medium population scenario, ensemble mean; Schewe et al. Citation2014). On average, 4% of the global land area (excluding Greenland and Antarctica) will be affected, for each degree of global warming, by a decrease in renewable groundwater resources of more than 30%, and 1% by a decrease of more than 70% (up to 4°C of global warming from 1971 to 2000, ensemble mean; Portmann et al. Citation2013).
By the end of the 21st century, the number of people affected annually by a late-20th-century 100-year river flood is projected to be three times greater for the high-emissions scenario (RCP8.5) than for the very low-emissions scenario (RCP2.6) (population fixed at 2005 level; Hirabayashi et al. Citation2013). Expected annual flood damages in Europe are estimated to increase, by the 2080s, from about 6 billion €/year to 14–15 billion €/year in the case of a low-emissions scenario (B2) and to 18–21 billion €/year in the case of a high-emissions scenario (A2) (Feyen et al. Citation2012). Unfortunately, it is very difficult to estimate costs of climate change impacts in monetary terms, and there is only a very limited number of studies (IPCC Citation2014).
ADAPTIVE WATER MANAGEMENT CAN SUPPORT REDUCTION OF WATER-RELATED RISKS IN A CHANGING CLIMATE AND THUS ADAPTATION TO CLIMATE CHANGE IN THE WATER SECTOR
Water management needs to assess and reduce the water-related risks of climate change. This involves decision-making in a changing world, with continuing uncertainty about the severity and timing of climate change and its impacts, as well as uncertainty of water-related developments not related to climate change (e.g. increased urbanization or water pollution). To promote sustainable development, water management needs to reduce exposure and vulnerability to water-related hazards in a changing climate, thus supporting adaptation to climate change. To manage water-related risks in a changing climate, integrated water resources management (IWRM), disaster risk management (of water-related disasters such as floods or droughts) and climate change adaptation have to be brought together. Both in principle and in practice, an adaptive approach to water management can cope with the uncertainties of future climate and societal development.
IWRM is an iterative, evolutionary and adaptive process, conceptualized as a “spiral” of problem and goal definition, strategy development, implementation, monitoring and, at the beginning of the next iteration, problem and goal redefinition based on what was learnt during the previous iteration (UNESCO Citation2009). With each iteration, new information and changing external conditions can be taken into account. With this conceptualization, IWRM fits very well with the concept of iterative risk management that is considered to be an appropriate approach for dealing with the risks of climate change (). Thus, “iterative risk management is a useful framework for decision making in complex situations characterized by large potential consequences, persistent uncertainties, long timeframes, potential for learning, and multiple climatic and non-climatic influences changing over time” (IPCC Citation2014, p. 9).
Techniques of adaptive water management include scenario planning, experimental approaches that involve learning from experience, and the development of flexible and low-regret solutions that work satisfactorily within the range of plausible climate futures. Low-regret solutions are suitable for climate change adaptation under uncertainty (), and include measures where moderate investment clearly increases the capacity to cope with projected risks or for which the investment is justifiable under all or almost all plausible scenarios. Involving all stakeholders, reshaping planning processes, coordinating the management of land and water resources, recognizing linkages between water quantity and quality, using surface water and groundwater conjunctively, and protecting and restoring natural systems, are examples of principles that can beneficially inform planning for adaptation (World Bank Citation2007). A comprehensive overview of adaptive water management that explicitly incorporates climate change and its uncertainty is the three-step framework of the US Water Utilities Climate Alliance (WUCA Citation2010): system vulnerability assessment, utility planning using decision-support methods, and decision making and implementation.
A first step towards adaptation to future climate change is the reduction of vulnerability and exposure to present climate variability (, IPCC Citation2014). Water management measures that increase resilience across a range of possible future climates include rainwater harvesting, conservation tillage, maintaining vegetation cover, planting trees on steep slopes, mini-terracing for soil and moisture conservation, improved pasture management, water re-use, desalination, protection and restoration of freshwater habitats and of the water retention capacity of floodplains, as well as improved soil and irrigation management. Jiménez et al. (Citation2014, Table 3-3) provide a list of climate change adaptation options for the management of freshwater resources. Typically, “soft” institutional measures are combined with “hard” infrastructural measures. Measures have to be tailored to local socio-economic and hydrological conditions. For instance, measures that work for perennial rivers cannot be applied in the case of ephemeral rivers (Benito et al. Citation2010). Early warning systems, e.g. for floods and droughts, can reduce adverse climate change impacts. Vulnerability can also be reduced by management measures that help improve human health, livelihoods, social and economic well-being, and environmental quality.
Climate change is only one of the multiple interacting stressors of freshwater systems, all of which have to be managed well. Reduction of risks caused by non-climatic drivers such as human water demand and pollutant emissions will often reduce climate-related risks, as vulnerability to climate change is decreased. In this way, managing the risks of climate change as conceptualized by the IPCC WGII () may at the same time contribute to reducing risks caused by non-climatic drivers.
WATER MANAGEMENT NEEDS TO CONTRIBUTE TO REDUCTION OF GREENHOUSE GAS EMISSIONS AND NOT RESTRICT ITSELF TO CLIMATE CHANGE ADAPTATION
There is high confidence that freshwater-related risks of climate change can only be reduced to a certain extent by climate change adaptation efforts. This is shown in Assessment Box SPM.2 of IPCC (Citation2014, Table 1), which presents key regional risks from climate change, as well as the potential for reducing these risks through adaptation and mitigation. Ten out of the 23 identified key risks in the eight world regions considered in WGII AR5 (excluding the “Oceans” region) concern freshwater. In all cases, risks that remain even after optimal and costly adaptation are assessed to be higher in a 4°C world (a world in which global mean temperature is 4°C higher than in the pre-industrial period, occurring at the end of the 21st century in case of the high-emissions scenario RCP8.5) than in a 2°C world, that can only be reached, at the end of the 21st century, with very strong GHG emissions reductions (RCP2.6).
Given the strong links between greenhouse gas emissions and water management, water management should give priority to both adaptation to and mitigation of climate change. Some of these links can be identified by assessing the water–energy–food nexus (). Water is required for energy production, and some forms of renewable energy put more stress on freshwater systems than others. Hydropower has negative impacts on freshwater ecosystems, which can be reduced by appropriate management. If irrigated, bioenergy crops make a much higher consumptive water demand than other mitigation measures. Climate-change mitigation by carbon capture and storage may decrease groundwater quality, while afforestation can reduce renewable water resources but also flood risk and soil erosion. However, given the need to reduce freshwater-related risks of climate change by minimizing climate change, even those climate-change mitigation actions that imply risks for freshwater systems may turn out to be desirable from the perspective of sustainable water management. Therefore, a thorough assessment of trade-offs and alternative measures for emissions reductions is required where such mitigation measures is proposed.
There are also water management measures that affect greenhouse gas emissions and thus have the potential to contribute to climate-change mitigation. Drainage of wetlands results in carbon dioxide emissions, and peatland rewetting could substantially reduce net greenhouse gas emissions. If rice paddies are drained and refilled (e.g. once or twice) during the growing season, methane emissions could be reduced without increasing significantly the nitrous oxide emissions, but this increases irrigation water demand. As energy is required for water supply and wastewater disposal, the water sector can contribute to climate-change mitigation by reducing its energy demand and using renewable forms of energy (). Trade-offs between minimizing greenhouse gas emissions and securing water supply under climate change exist and can be analysed using a multi-objective optimization approach (see Paton et al. Citation2014 for the example of an urban water supply system in Australia).
CONCLUSIONS
Water is key for many regions, sectors, systems and users. This is why water is discussed in many of the chapters of the IPCC WGII assessment, both as a source of risks and as a means for adaptation. Anthropogenic climate change has made water management more difficult as hydrological conditions will change in the future in a highly uncertain way. To achieve water security in a changing climate, the well-established approach of adaptive IWRM needs to be extended with respect to the risks of climate change. Managing the risks of climate change in IWRM means that the uncertainty of future climate and its impacts are fully embraced in decision making. This can be done by assessing the future water conditions for different scenarios probabilistically, and results in the need to develop a portfolio of low-regret solutions that reduce vulnerability and can be implemented and modified progressively as future conditions evolve.
Acknowledgements
Most of the material presented in this paper results from the authors’ work on the freshwater resources chapter and the summary for policy-makers of the Working Group II contribution to the IPCC’s Fifth Assessment Report. The authors thank the many people who participated in the IPCC process, including IPCC board and Technical Support Unit members, contributing authors, co-authors of cross-chapter boxes and hundreds of reviewers. Finally, we thank Dawen Yang, Alberto Montanari, an anonymous reviewer and the editor for their thoughtful comments that helped to improve the manuscript.
REFERENCES
- Arent, D.J., et al., 2014. Cross-chapter box on the water-energy-food/feed/fiber nexus as linked to climate change. In: C.B. Field, et al., eds. Climate change 2014: impacts, adaptation, and vulnerability. Part A: global and sectoral aspects. Contribution of Working Group II to the Fifth Assessment Report of the Intergovernmental Panel on Climate Change [online]. Cambridge: Cambridge University Press, 163–166. Available from: http://ipcc-wg2.gov/AR5/ [Accessed 23 October 2014].
- Arnell, N.W. and Lloyd-Hughes, B., 2014. The global-scale impacts of climate change on water resources and flooding under new climate and socio-economic scenarios. Climatic Change, 122, 127–140. doi:10.1007/s10584-013-0948-4
- Benito, G., et al., 2010. Management of alluvial aquifers in two southern African ephemeral rivers: implications for IWRM. Water Resources Management, 24, 641–667. doi:10.1007/s11269-009-9463-9
- Chen, J., Brissette, F.P., and Leconte, R., 2011. Uncertainty of downscaling method in quantifying the impact of climate change on hydrology. Journal of Hydrology, 401 (3–4), 190–202. doi:10.1016/j.jhydrol.2011.02.020
- Crosbie, R.S., et al., 2013. An assessment of the climate change impacts on groundwater recharge at a continental scale using a probabilistic approach with an ensemble of GCMs. Climatic Change, 117 (1–2), 41–53. doi:10.1007/s10584-012-0558-6
- Dankers, R., et al., 2014. A first look at changes in flood hazard in the ISI-MIP ensemble. Proceedings of the National Academy of Sciences of the United States of America, 111 (9), 3257–3261. doi:10.1073/pnas.1302078110
- Davie, J.C.S., et al., 2013. Comparing projections of future changes in runoff from hydrological and biome models in ISI-MIP. Earth System Dynamics, 4, 359–374. doi:10.5194/esd-4-359-2013
- Döll, P. and Bunn, S.E., 2014. Cros-chapter box on the impact of climate change on freshwater ecosystems due to altered river flow regimes. In: C.B. Field, et al., eds. Climate change 2014: impacts, adaptation, and vulnerability. Part A: global and sectoral aspects. Contribution of Working Group II to the Fifth Assessment Report of the Intergovernmental Panel on Climate Change [online]. Cambridge: Cambridge University Press 143–146. Available from: http://ipcc-wg2.gov/AR5/ [Accessed 23 October 2014].
- EU (European Union), 2007. Directive 2007/60/EC of the European Parliament and of the council of 23 October 2007 on the assessment and management of flood risks. Official Journal of the European Union, L 288, 27–34.
- Feyen, L., et al., 2012. Fluvial flood risk in Europe in present and future climates. Climatic Change, 112 (1), 47–62. doi:10.1007/s10584-011-0339-7
- Field, C.B., et al., eds., 2012. Managing the risks of extreme events and disasters to advance climate change adaptation. Special report of working groups I and II of the Intergovernmental Panel on Climate Change (IPCC). Cambridge: Cambridge University Press [online]. Available from: http://www.ipcc.ch/pdf/special-reports/srex/SREX_Full_Report.pdf [Accessed 23 October 2014].
- Field, C.B., et al., eds., 2014. Climate change 2014: impacts, adaptation, and vulnerability. Part A: global and sectoral aspects. Contribution of Working Group II to the Fifth Assessment Report of the Intergovernmental Panel on Climate Change (IPCC) [online]. Cambridge: Cambridge University Press. Available from: http://ipcc-wg2.gov/AR5/ [Accessed 23 October 2014].
- Gerten, D., Betts, R., and Döll, P., 2014. Cross-chapter box on the active role of vegetation in altering water flows under climate change. In: C.B. Field, et al., eds. Climate change 2014: impacts, adaptation, and vulnerability. Part A: global and sectoral aspects. Contribution of Working Group II to the Fifth Assessment Report of the Intergovernmental Panel on Climate Change [online]. Cambridge: Cambridge University Press, 157–161. Available from: http://ipcc-wg2.gov/AR5/ [Accessed 23 October 2014].
- Hagemann, S., et al., 2013. Climate change impact on available water resources obtained using multiple global climate and hydrology models. Earth System Dynamics, 4, 129–144. doi:10.5194/esd-4-129-2013
- Hirabayashi, Y., et al., 2013. Global flood risk under climate change. Nature Climate Change, 3, 816–821. doi:10.1038/nclimate1911
- IPCC (Intergovernmental Panel on Climate Change), 2013. Summary for policymakers. In: T.F. Stocker, et al., eds. Climate change 2013: the physical science basis. Contribution of Working Group I to the Fifth Assessment Report of the Intergovernmental Panel on Climate Change [online]. Cambridge: Cambridge University Press, 1–19. Available from: http://www.climatechange2013.org/report/ [Accessed 23 October 2014].
- IPCC (Intergovernmental Panel on Climate Change), 2014. Summary for policymakers. In: C.B. Field, et al., eds. Climate change 2014: impacts, adaptation, and vulnerability. Part A: Global and sectoral aspects. Contribution of Working Group II to the Fifth Assessment Report of the Intergovernmental Panel on Climate Change [online]. Cambridge: Cambridge University Press, 1–32. Available from: http://ipcc-wg2.gov/AR5/ [Accessed 23 October 2014].
- Jiménez, B.E.C., et al., 2014. Freshwater resources. In: C.B. Field, et al., eds. Climate change 2014: impacts, adaptation, and vulnerability. Part A: global and sectoral aspects. Contribution of Working Group II to the Fifth Assessment Report of the Intergovernmental Panel on Climate Change [online]. Cambridge: Cambridge University Press, 229–269. Available from: http://ipcc-wg2.gov/AR5/ [Accessed 23 October 2014].
- Koutsoyiannis, D., et al., 2009. Climate, hydrology and freshwater: towards an interactive incorporation of hydrological experience into climate research—DISCUSSION of “The implications of projected climate change for freshwater resources and their management”. Hydrological Sciences Journal, 54 (2), 394–405. doi:10.1623/hysj.54.2.394
- Kundzewicz, Z.W., et al., 2008. The implications of projected climate change for freshwater resources and their management. Hydrological Sciences Journal [online], 53 (1), 3–10. Available from: http://www.tandfonline.com/doi/pdf/10.1623/hysj.53.1.3 [Accessed 23 October 2014].
- Kundzewicz, Z.W., et al., 2009. Water and climate projections, REPLY to discussion “Climate, hydrology and freshwater: towards an interactive incorporation of hydrological experience into climate research”. Hydrological Sciences Journal, 54 (2), 406–415. doi:10.1623/hysj.54.2.406
- Milly, P.C.D., et al., 2008. Stationarity is dead: whither water management? Science, 319, 573–574. doi:10.1126/science.1151915
- Paton, F.L., et al., 2014. Including adaptation and mitigation responses to climate change in a multiobjective evolutionary algorithm framework for urban water supply systems incorporating GHG emissions. Water Resources Research, 50 (8), 6285–6304. doi:10.1002/2013WR015195
- Portmann, F.T., et al., 2013. Impact of climate change on renewable groundwater resources: assessing the benefits of avoided greenhouse gas emissions using selected CMIP5 climate projections. Environmental Research Letters, 8 (2), 024023. doi:10.1088/1748-9326/8/2/024023
- Quintana Segui, P., et al., 2010. Comparison of three downscaling methods in simulating the impact of climate change on the hydrology of Mediterranean basins. Journal of Hydrology, 383 (1–2), 111–124. doi:10.1016/j.jhydrol.2009.09.050
- Schewe, J., et al., 2014. Multi-model assessment of water scarcity under climate change. Proceedings of the National Academy of Sciences of the United States of America, 111 (9), 3245–3250. doi:10.1073/pnas.1222460110
- Stocker, T.F., et al., eds. 2013. Climate change 2013: the physical science basis. Contribution of working group I to the fifth assessment report of the Intergovernmental Panel on Climate Change (IPCC) [online]. Cambridge: Cambridge University Press. Available from: http://www.climatechange2013.org/report/ [Accessed 23 October 2014].
- UNESCO, 2009. IWRM guidelines at river basin level. Part 1: principles [online]. Available from: http://unesdoc.unesco.org/images/0018/001864/186417e.pdf [Accessed 23 October 2014].
- World Bank, 2007. Mainstreaming adaptation to climate change in agriculture and natural resources management projects: climate change team environment department. Guidance Notes 7. Washington, DC: World Bank, 18 pp.
- WUCA (Water Utility Climate Alliance), 2010. Decision support planning methods: incorporating climate change uncertainties into water planning. San Francisco, CA: Water Utility Climate Alliance (WUCA), 102 pp.