ABSTRACT
A distributed hydrological model (WaSiM-ETH) was applied to a mesoscale catchment to investigate natural flood management as a nonstructural approach to tackle flood risks from climate change. Peak flows were modelled using climate projections (UKCP09) combined with afforestation-based land-use change options. A significant increase in peak flows was modelled from climate change. Afforestation could reduce some of the increased flow, with greatest benefit from coniferous afforestation, especially replacing lowland farmland. Nevertheless, large-scale woodland expansion was required to maintain peak flows similar to present and beneficial effects were significantly reduced for larger “winter-type” extreme floods. Afforestation was also modelled to increase low-flow risks. Land-use scenarios showed catchment-scale trade-offs across multiple objectives meant “optimal” flood risk solutions were unlikely, especially for afforestation replacing lowland farmland. Hence, combined structural/nonstructural measures may be required in such situations, with integrated catchment management to synergize multiple objectives.
Editor A. Castellarin ASSOCIATE EDITORH. Kreibich
1 Introduction
River flooding is a major cause of damage, injury and loss of life (Jongman et al. Citation2012). Intensification of the hydrological cycle due to climate change is expected to further increase this risk (Milly et al. Citation2002, Hirabayashi et al. Citation2008). Warmer air is more energetic and can hold more moisture, implying an increased likelihood of future extreme events as the climate warms (Allan and Soden Citation2008, IPCC Citation2012). Observational evidence suggests that a trend towards increased precipitation rates is evident in some regions and that this can be attributed to climate change (Lehmann et al. Citation2015). However, relating changes in precipitation to river flows and hence the occurrence of damaging flood events is a complex process that is also contingent on the local context of each river catchment, including factors such as topography, soils, land use and urbanization (Merz and Blöschl Citation2003, Whitfield Citation2012, Ivancic and Shaw Citation2015). River flow response to a given meteorological event is controlled primarily by the availability of water storage within a catchment, the status of that storage due to antecedent conditions, and the response time of catchment water stores to precipitation inputs (Garner et al. Citation2015).
Results from linked climate and hydrological models identify northwest Europe as a region that is likely to experience increased flood risk (Dankers and Feyen Citation2008). However, projected future changes show large variations in the magnitude of risk due to assumptions inherent in the choice of hydrological or climate model, or the climate change scenario (Feyen et al. Citation2012, Kundzewicz et al. Citation2016). Furthermore, although the potential for combined effects has been highlighted (Bronstert et al. Citation2002), most projections do not include the impacts of climate change aggregated with other hydrological drivers. These other drivers, notably land-use change, are known from assessments of historic change to have had a significant role in modifying river flows and flooding regimes (Werritty et al. Citation2006, Wilby et al. Citation2008). Hence, despite good evidence that both climate change (Steele-Dunne et al. Citation2008, Gädeke et al. Citation2014) and land-use change (Niehoff et al. Citation2002, Hundecha and Bárdossy Citation2004, Archer et al. Citation2010) can modify flood hydrology, very few studies have explored catchment-scale interactions between these two drivers (Dwarakish and Ganesri Citation2015). An exception was Bronstert et al. (Citation2007), who used meteorological-forcing increments and land-use change scenarios to highlight the importance of scale in understanding such interactions.
Changes in river flows have important implications for the design of flood protection schemes, challenging conventional design principles that assume stationarity of risk factors (Milly et al. Citation2008). This dichotomy has led to calls for a paradigm shift in concepts and practice of flood risk management in order to facilitate successful adaptation, recognizing multiple systemic risk factors influencing both flood hazard exposure and societal vulnerability (Merz et al. Citation2010, Sayers et al. Citation2014). One adaptation strategy is to prioritize the site-specific upgrade of flood defence structures for the vulnerable areas, notably cities and towns, which are experiencing or expected to experience a change in flood risk. However, this strategy has implications not just in terms of additional economic costs but also regarding environmental consequences and amenity values: flood defence structures modify the natural morphology and habitat of the river and its floodplain, which can lead to loss of biodiversity and associated ecosystem services (Roquette et al. Citation2011). In addition, irreducible uncertainties inherent within climate change projections mean that setting structural design limits through conventional “best estimate” risk assessment approaches incurs a possibility of being locked in to a specific future pathway that does not materialize (Lawrence et al. Citation2013). These challenges have therefore led to greater interest in the role of nonstructural measures in reducing flood risk (Alfieri et al. Citation2016, Ciullo et al. Citation2017).
Natural flood management (NFM) schemes encompass a wide variety of options that aim to work with natural hydrological and hydromorphological processes to manage sources and pathways of flood waters, thereby reducing flood risk (Environment Agency Citation2010). This may include restoration, enhancement or alteration of natural features and characteristics that attenuate rainfall–runoff processes, store water, and attenuate flow regimes of streams and rivers, notably through land and soil management, which have been shown to influence local hydrology (O’Connell et al. Citation2007, Hess et al. Citation2010).
Using afforestation as a type of NFM has been demonstrated in a series of studies (e.g. Andréassian Citation2004, Nisbet and Thomas Citation2008). Afforestation can modify hydrological pathways through increases in interception (Robinson et al. Citation2003), infiltration (Bracken and Croke Citation2007), temporary storage (Ghavasieh et al. Citation2006) or by slowing conveyance (Thomas and Nisbet Citation2006, Lane et al. Citation2007) and attenuating runoff (Hundecha and Bárdossy Citation2004). Increasing woodland in upstream areas has been shown to reduce downstream peak flows using observations from paired catchments (McVicar et al. Citation2007) and model-based assessments (Francés et al. Citation2008, Salazar et al. Citation2012). Experimental evidence from upland control/treatment plots (144 m2) at Pontbren (central Wales, UK) compared 2 years of baseline data of intensive agricultural grazing against a similar period when newly planted with broadleaved woodland, and found woodland could enhance soil infiltration rates and reduce bulk runoff coefficients (aggregated runoff/rainfall ratios) by 78% on average; however, removal of grazing animals alone reduced runoff coefficients by an average of 48% (Marshall et al. Citation2014). Modelling of flood conveyance processes in association with restoration of lowland forested floodplains (20–40% afforestation of the 98 km2 Lymington catchment, southern England, UK) suggested reductions in peak discharge of up to 19% over a 25-year period through de-synchronization of the timings of sub-catchment flood waves (Dixon et al. Citation2016b).
Natural flood management studies have been used to suggest that flood risk management and climate change adaptation could be enhanced by strategic planting of trees and other catchment-scale initiatives; however, evidence appraisals also acknowledge the need for a stronger knowledge base on which to formulate such decisions (Orr et al. Citation2008). Catchment-scale outcomes have often been assumed to scale up from small-scale NFM interventions from which most results are obtained, but there is a relative paucity of evidence on the scale of changes required to alleviate flood risk for catchments over 10 km2 in size (O’Connell et al. Citation2007, Parrott et al. Citation2009, Lane and Milledge Citation2013). As the dominant processes influencing runoff response and flooding are nonlinear and hence vary across scales (Blöschl et al. Citation2007, Bronstert et al. Citation2007), it has been cautioned that results at smaller scales should not simply be generalized to larger scales (Deasy et al. Citation2014). The spatial configuration of land use, rather than just areal components, has also been identified as a key factor controlling runoff and catchment discharge (Ludwig et al. Citation2005). Furthermore, there is rather limited evidence on how land-based NFM options modify floods of different magnitudes or throughout the year. Typically, seasonal variations occur in flood-generating processes: “long-rain floods”, common in winter in northwest Europe, are driven by weeks to months of lower-intensity, advective rainfall that exceeds the storage capacity of the soil and results in saturation-excess overland flow; “short-rain floods”, common in summer, are driven by short-duration, high-intensity, convective rainfall that results in infiltration-excess flow at the surface or sub-surface (Merz and Blöschl Citation2003, Bronstert et al. Citation2007).
The present study aims to improve understanding of catchment-scale NFM as a climate change adaptation strategy, not only regarding peak flows, but also in the context of other ecosystem services (Iacob et al. Citation2014, Collentine and Futter Citation2016). Specific objectives are: (a) to establish the influence of climate change on peak flows; (b) to evaluate the efficacy of afforestation as a form of NFM in reducing peak flows; and (c) to investigate synergies and trade-offs between NFM and other land-use issues.
2 Study area
Tarland Burn is a tributary of the River Dee in northeast Scotland (catchment area 72 km2; elevation 100–617 m a.s.l.; ). The location has a history of disruptive flood events, including in December 2000, October 2002, December 2005, March 2006, February 2009, July 2009, May 2010, December 2013 and December 2015. Most of these flood events occurred in typical “winter” conditions from surface runoff when soils were saturated; the October 2002 and December 2015 events were categorized as “major” due to the scale of inundation and damage to properties. The catchment is situated in impermeable rocks with soils that are mainly cambisols, but with humus-iron podsols on higher ground and a lower basin floor underlain by fine-grained alluvium. A variety of land uses are supported, predominantly arable, improved or unimproved grassland, woodland (mostly coniferous) and upland heath. To facilitate agricultural improvement, the alluvial zone was drained in the 19th century. The Tarland Burn is included in an EU Natura2000 Special Protection Area (SPA) designation for the larger Dee basin, based on biodiversity value. This has led to increased interest in alternative approaches for flood risk management, including nonstructural measures. At the same time, the Scottish Government Land Use Strategy has identified a national policy priority for woodland expansion. Hence, the role of afforestation in reducing flood risk and providing other benefits has been considered as a potential “win-win” option. Historically, emphasis has been placed on coniferous plantations because of their faster growth rates and advantages for timber production, but grant incentives now particularly encourage planting native-species broadleaved woodland (Brown et al. Citation2014, Nijnik et al. Citation2016).
3 Methods
3.1 Model description
Empirical work in mesoscale catchments has significant logistical challenges; therefore, numerical modelling provides a rational alternative (Parrott et al. Citation2009, Pattison and Lane Citation2012). As the present study aimed to investigate spatial and temporal variations in hydrological processes associated with climate and land-use change, a distributed hydrological model (WaSiM-ETH) was employed to parameterize catchment variability in soils, topography, land cover and climate on a regular grid. Calibration and validation of the model were undertaken to adequately capture the hydrology of Tarland Burn catchment, and then it was then used to explore hydrological variability due to changes in climate and land use. Change in peak flow was used as the key indicator of modified flood risk.
The WaSiM-ETH model is a fully-distributed, physically-based hydrological model that has been previously used for investigations of land use (Niehoff et al. Citation2002, Verbunt et al. Citation2005, Hölzel et al. Citation2011) and climate change (Jasper et al. Citation2004, Gädeke et al. Citation2014). It has also been used to distinguish different flood generation processes within a catchment (Bronstert et al. Citation2007). Vertical movement of water in the soil is assumed to be one-dimensional within the unsaturated zone, with no exchange of water taking place between neighbouring cells. Soil cells are vertically defined by horizons and grouped into similar classes based on soil type. Water in excess of infiltration capacity feeds directly to surface runoff, and the amount of infiltrating water serves as an upper boundary condition in the unsaturated zone. Percolation and capillary rise are determined by the soil properties and simulated by corresponding vertical moisture profiles and fluxes. The Van Genuchten (Citation1980) equation is used to estimate soil-water retention and release based on hydraulic head and conductivity, soil matrix potential, and the proportion of saturated and residual water content. Water fluxes are calculated on a regular grid using the Richards (Citation1931) equations in the unsaturated zone, but are complemented by a model extension to simulate preferential flow through macropores direct to the saturated zone when precipitation intensity exceeds a threshold infiltration rate associated with the soil matrix. Linear storage approaches are applied to interflow and direct runoff using a single reservoir cascade method (isochronic with additional retention), requiring the calibration of the recession constants due to flow retention. Surface runoff is generated for each grid cell, incorporating both the infiltration excess and saturation excess overland flow when soils are saturated. The generated runoff in each cell is routed to the outlet of the basin by topographic analysis with flow times calculated using the Manning-Strickler equation (Schulla and Jasper Citation2007). Flow velocities for the different water levels in the channel are calculated using both a kinematic wave approach and simple linear storage.
Precipitation type is estimated for each grid cell using the interpolated air temperature: both rainfall and snowfall can occur at the same time within the transition range, and the same temperature-index approach was used to estimate snowmelt. Potential evapotranspiration (ET) is calculated using the Penman-Monteith method based on bulk-surface resistance values referenced for each land-cover type (Monteith Citation1975, Brutsaert Citation1982). To calculate actual ET, potential ET is reduced by the amount of water equal to the interception storage of the plant canopy followed by a reduction based on soil suction properties and plant physiological properties of the land cover (Schulla and Jasper Citation2007). Interception storage is estimated using a simple bucket approach dependent on the total leaf coverage and the maximum height of the water layer on the vegetation. The extraction of water by ET from interception storage is considered at a potential rate in the model. If there is a sufficient amount of water held in interception storage, the storage content is reduced by the potential ET, and no water will be lost from the soil. If the interception storage content is smaller than the potential ET rate, the remaining content will be removed from the soil, unless the soil is too dry when required suction values become too high for plants to access soil moisture.
3.2 Model set-up and application
The WaSiM-ETH model was set up on an hourly time step and all spatial data configured on a 50-m grid. For topographic data, a digital elevation model (DEM) was derived from the Ordnance Survey Land-Form PROFILE dataset. Baseline land-cover data were derived from UK Land Cover Map 2007 (LCM2007: Morton et al. Citation2011) and grouped into broad classes ()), each associated with key model parameters, including leaf area index, rooting depth and aerodynamic roughness (Breuer et al. Citation2003). Soil mapping units were derived from digital 1:25 000 soils maps and attributed according to type profiles in the National Soils Inventory for Scotland (Scotland’s Soils Citation2016). Field drains in the alluvial area were set at a spacing of 25 m based on available site evidence.
Soil matrix hydraulic properties for WaSiM-ETH were estimated from horizon texture data using pedotransfer functions in the ROSETTA program (Schaap et al. Citation2001). In addition, WaSiM-ETH allows the volume of macropores to be parameterized based on soil group properties. However, it is known from empirical data that different land uses can modify soil structure and permeability within the same texture class, particularly due to macropores acting as preferential flow pathways in the rooting zone (Jarvis et al. Citation2013). Gravity is the dominant force for water flow in macropores, with capillary flow negligible compared to its role in the soil matrix (Bevan and Germann Citation2013). Pedotransfer functions can therefore underestimate the importance of soil structure and overestimate texture in deriving hydraulic properties, particularly close to saturation (Gonzalez-Sosa et al. Citation2010, Vereecken et al. Citation2010). To account for this land-use influence, soil saturated hydraulic conductivity (Ks) values as estimated by ROSETTA were further modified by incremental adjustments () derived from analysis of field data by Archer et al. (Citation2013), which, although measured outside Tarland catchment, were based on land-use variations in Ks across similar soil groups. These adjustments are consistent with a wider literature identifying that woodland areas have higher hydraulic conductivity and infiltration rates compared to other land uses due to the presence of extensive deep-rooting systems, and associated fauna, which increases macropore volumes (Lange et al. Citation2009, Peng et al. Citation2012, Schwärzel et al. Citation2012, Jarvis et al. Citation2013, Marshall et al. Citation2014). On arable land, use of heavy machinery with annual crops has modified soil structure such that macropores are less evident, whereas more persistent rooting systems in permanent grassland, especially in less intensively-used semi-natural zones, allow a relative increase in permeability compared to arable land (Gonzales-Sosa et al. Citation2010). Existing evidence was not considered robust enough to quantify different hydraulic properties for coniferous and deciduous woodland (Jost et al. Citation2012). Older woodlands have been found to have higher hydraulic conductivity (Archer et al. Citation2013), but, as the present study is investigating the comparative influence of newly-planted woodland, this age distinction was not included. Hence, represents catchment-scale simplification of soil properties that are often highly variable, spatially and temporally (Archer et al. Citation2016, Jirků et al. Citation2013).
Table 1. Modified soil saturated hydraulic conductivity (Ks) based on land-use type.
3.3 Land-use change
The impact of land-use change was investigated through both sensitivity testing and scenario analysis. For sensitivity testing, proportions of woodland (coniferous or deciduous) were incrementally modified to replace other land uses together with different spatial configurations to explore its influence on hydrology. Scenario analysis investigated concurrent changes in multiple land-cover types as a response to large-scale drivers, providing more realistic but more complex landscape configurations against which to explore hydrological change.
To facilitate both types of analysis, the LandsFACTS toolkit was employed to develop different spatial land-cover configurations. Based on a given set of constraints, LandsFACTS will generate multiple spatial and temporal land-cover allocations for a landscape (Castellazzi et al. Citation2010). To test the influence of afforestation location, two general layouts were investigated: (a) upland afforestation, with a preference for replacing semi-natural habitats and unimproved grassland; and (b) lowland afforestation, with a preference for replacing cultivated land (arable and improved grassland).
For the scenario analysis, possible future changes in land use for the study area in 2050 were available from a previous cross-sectoral assessment using the combined influence of socio-economic scenarios (IPCC SRES framework) and climate change projections (UKCP09) on land-use decisions (Brown and Castellazzi Citation2014; ; ). These scenarios also incorporate prospective responses by decision makers to a warming climate, notably the possibility of an increased area of land capable of being used for intensive agriculture in Scotland. Future scenarios of agricultural intensification associated with a policy priority for food security (national enterprise, NE) or globalization (world markets, WM) therefore act against increased afforestation in some parts of the catchment, particularly lowland areas. These intensification scenarios have previously been shown to lead to rather different land-use patterns in the Tarland catchment when compared to scenarios in which environmental regulation (global sustainability) or community-level decisions (local stewardship) are prioritized (). The global sustainability (GS) or local stewardship (LS) scenarios therefore provide more scope for woodland expansion, whilst also prioritizing native broadleaved rather than non-native coniferous woodland ().
Table 2. Land-use change scenarios (% area of each land use).
Figure 2. Land-use scenario descriptions (after Brown and Castellazzi Citation2014).
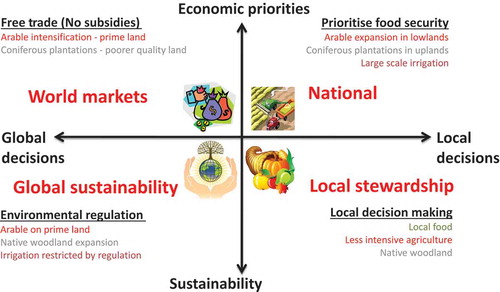
3.4 Climate change
For model calibration (Section 3.5), hourly meteorological data were obtained from Aboyne weather station (archived by British Atmospheric Data Centre). Use was made of a WaSiM-ETH module to interpolate meteorological parameters from station data across the catchment model grid using regression routines to infer parameter relationships with topography (provided by the DEM). To analyse the influence of climate change, synthetic data were derived using the UKCP09 weather generator (WG) (Jones et al. Citation2009). The UKCP09 project provided probabilistic climate projections from an ensemble of global climate models (GCMs), which were further downscaled to 25-km scale using the HadRM3 (Met Office Hadley Centre) regional climate model (RCM) (Murphy et al. Citation2009). The UKCP09 WG allows further downscaling to 5 km based on the use of statistically-derived relationships between parameters, as derived from an observed gridded climatology (Perry and Hollis Citation2005); each run of the WG for future periods represents a stochastic sample from the UKCP09 probability distribution which is constructed as an hourly time series using relationships from the observed climatology. For the present study, 30 years of hourly data were derived from the WG based on aggregation of the 5-km grid cells representing Tarland catchment. For each 30-year period, 100 sample runs of the WG were employed for both baseline (1961–1990) and future periods (2020s, 2050s, 2080s) with future runs based on the UKCP09 medium emissions scenario (equivalent to the IPCC A1B emissions scenario).
3.5 Model calibration and validation
Calibration and validation of the model was undertaken using meteorological data for the period January 2004 to June 2009 and calibrated against flow data for Tarland Burn using gauging stations at Aboyne and Coull. The data record from Coull gauge had consistency problems after 2007 due to channel modification, whereas Aboyne gauge records had some missing data due to instrumental malfunction. Hence, calibration was based on the 2004 and 2005 data from Coull, referenced against Aboyne data for goodness-of-fit purposes, whilst validation used 2006 data from Coull and 2006–2008 data from Aboyne. Calibration was conducted using a nonlinear parameter estimation routine (PEST) that fits model to observation data by minimizing the weighted sum squared error, using a robust variant of the Gauss-Marquardt-Levenberg method that requires fewer model runs compared with similar algorithms for solving nonlinear problems (Doherty and Skahill Citation2006, Singh et al. Citation2012). Nash-Sutcliffe efficiency (NSE) coefficients (Nash and Sutcliffe Citation1970) were calculated for each calibration step and, if the results were considered unsatisfactory (NSE < 0.5), model parameter data were refined before running another set of simulations and recalculating the efficiency coefficients.
3.6 Flow analysis
The overall response of flow regimes to land-use and climate change was summarized using flow duration curves (FDCs) to show discharge values exceeded for a given percentage of time (e.g. 5% time for Q5 discharge; Vogel and Fennessey Citation1994). To evaluate changes to extreme high flows, additional analysis was conducted, distinguishing “summer” and “winter” model calibration to allow for differing seasonal antecedent conditions, notably that storage capacity would typically be more limited during typical “winter” conditions when “long rain” events and saturated soils predominate. Extreme flows were generated from annual maxima of the 30-year WG time series data by using the general extreme values (GEV) probability distribution and L-moments fitting technique to calculate large return period events; this approach has previously been found to provide a robust technique for frequency analysis (Fowler and Kilsby Citation2003, Svensson and Jones Citation2010). Total rainfall for return period events was distributed back to an hourly time step for 7-h and 15-h events using depth–duration–frequency design profiles provided by the UK Flood Estimation Handbook on a 1-km grid, as derived from local raingauges (with at least 10 years of data) and catchment descriptors (Institute of Hydrology Citation1999). The 7-h event is identified as the critical design period based on catchment size, whereas a longer duration is represented by the 15-h event.
4 Results
4.1 Model calibration and validation
The main parameters required for catchment-specific calibration of WaSiM-ETH (Wriedt and Rode Citation2006) were set using the PEST tool () to provide a satisfactory calibration (R2 = 0.76 at Coull; R2 = 0.75 at Aboyne; NSE = 0.76 at Coull; NSE = 0.68 at Aboyne). Validation at the two gauge sites showed a good general fit between observed and modelled discharge (R2 = 0.76 at Coull; R2 = 0.75 at Aboyne; NSE = 0.63 at Coull; NSE = 0.6 at Aboyne), indicating that the model performed well in simulating the overall flow regime (see Supplementary material). However, there is an indication that the model is underestimating some high flow peaks in winter, which may be related to difficulties in simulating snowmelt or rain-on-snow events because of their sensitivity to small temperature changes and other local meteorological interactions (Beven Citation2012). To a lesser extent, the model may also underestimate some flow peaks in summer; this may be related to difficulties in identifying small-scale convectional events that produce locally intense rainfall, but which are only partially represented in the weather station data or have an unusual relationship with catchment topography.
Table 3. Model calibration data.
Modelling simulates saturated overland flow as dominant throughout the catchment during winter-type flood events, which is consistent with observations. During summer-type flood events, infiltration excess is simulated for cambisols (mainly agricultural uses) and podsols on slopes around the basin, with this water routed by overland flow and inter-flow to the flat alluvial basin floor, where both infiltration excess and saturation occur as the capacity of the field drains is exceeded. Summer-type events are therefore rather more variable in terms of the relative dominance of flood-generating processes, related to differing precipitation rates and antecedent soil moisture conditions; as flood events are rarer they are more difficult to compare against limited observations.
4.2 Climate change and high flows
Extreme value analysis based on the WG data and hydrological modelling shows that the magnitude of precipitation events and extreme high flow events could increase substantially (). These results suggest that, by the 2080s, 100-year extreme flows could increase by up to approx. 26% for both winter and summer 7-h duration events, with possible greater changes for longer 15-h events in summer. The larger increases have been inferred for the more extreme (100-year) events, although this needs to be interpreted with caution because of the limited data on which it is based. An increase in extreme precipitation and peak flows would be consistent with previous work in Scotland that has analysed UKCP09 and HadRM3 data (Kay et al. Citation2014b).
Table 4. Changes for 10- and 100-year return period events for Tarland Burn catchment (% differences from baseline in parentheses): (a) precipitation (mm); (b) peak flow (m3 s−1) at Coull.
4.3 Afforestation and high flows
Sensitivity testing () showed a general relationship between increased afforestation extent and reduction in high flows (Q5 metric), flow reduction being proportional to the increase in woodland area. For example, a 24% increase in new woodland decreases Q5 flow by up to 19%. Woodland expansion with coniferous trees has a larger effect in reducing high flows; differences in flow reduction between coniferous and deciduous woodland were found greatest in winter when most flood events occur. Modelled reduction in high flows was therefore greatest for full catchment afforestation with coniferous woodland, albeit with the major caveat that such an outcome would be highly unlikely to happen because of the importance of agriculture in the study area (see Section 4.6). Greater reduction in high flows was found for woodland planted in the lowland zone replacing cultivated agricultural land: 10% new woodland produced 8% reduction in Q5 for coniferous and 1% reduction for deciduous woodland. The results for the same proportion of new upland afforestation were smaller: 5% reduction in Q5 for coniferous and 0.5% for deciduous woodland.
The results for extreme high flows () suggest some differences compared to general Q5 high flows. Assuming no climate change, full catchment afforestation with coniferous woodland decreased the winter 7-h 10-year event (12.5 m3 s1) by 30% (compared with 62% reduction for Q5), although such a large land-use change is considered unlikely. Lesser amounts of catchment afforestation produced a smaller proportional reduction in extreme high flows than this upper potential value and less reduction than found with Q5 flows. This suggests a diminution in the capacity of new woodland to reduce peak flows for the more extreme flood events; the main effects are modelled in summer and for smaller magnitude events.
Table 5. Peak flows (m3 s−1) for afforestation options and UKCP09 WG climate change scenarios (mean ensemble value medium emissions). con: coniferous; dec: deciduous.
The results also suggest that afforestation can contribute to flood risk management by delaying the time taken to reach peak flow. Model simulations show full afforestation (100% cover) with coniferous woodland delayed the time to peak flow by 2 h in the summer, and by 1 h in the winter, for a 10-year 15-h duration event. An increase in woodland to 75% cover delayed time to peak flow by 1 h for the same reference event (10-year event, 15-h duration) but only in summer. Similar results were found for larger magnitude events: for full coniferous afforestation, the 100-year 15-h event was found to take 1 h longer to reach its peak; however, for the same event there is almost negligible delay in peak flow for 75% afforestation. This suggests that large land-use changes are required to induce this delayed flood peak and that they may be less effective for the largest extreme events.
4.4 Afforestation and low flows
Sensitivity testing also showed that woodland expansion would cause flow reductions across the FDC, and not just for high flows (). Modelled reductions in low flows (represented by Q95), which would occur mainly during the summer, were found to be proportional to the extent of additional woodland in the catchment and were found to be greatest for coniferous woodland. For example, 75% conifer afforestation was found to reduce Q95 discharge by more than 50%, whilst 100% conifer afforestation was found to reduce Q95 by more than 70% ().
Figure 3. Illustrative land-use scenarios for Tarland catchment in 2050 (after Brown and Castellazzi Citation2014).
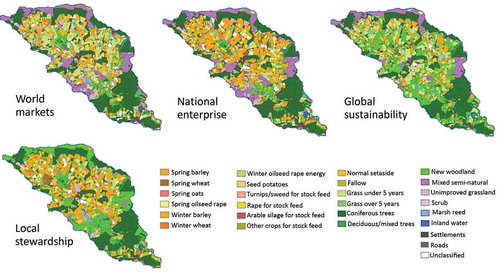
4.5 Afforestation and high flows with climate change
When land-cover changes are combined with future climate projections, results continue to show that afforestation could reduce high flows when compared to existing land use (). With full catchment coniferous afforestation, maximum reductions of 30% peak flow are modelled for a 10-year 7-h winter event for the 2080s compared to existing land use. For the same comparison in summer conditions, reductions could be even higher (up to 65%), but flow peaks are of smaller magnitude.
However, although peak flows are reduced by afforestation relative to existing land use, also shows that the actual magnitude of flood events will still increase in future. Hence, the increase in flood risk due to climate change appears to exceed the capacity of land-use change by afforestation to counteract it. Only full afforestation with coniferous woodland was able to reduce the magnitude of flood risk for the larger events (in winter) to be at a similar level to the baseline period by the 2080s, and this is more of a theoretical option rather than a realistic choice for the study area, due to the importance of agriculture.
4.6 Land-use change scenarios
In all cases, reductions in peak flows from the scenario analysis () were rather less than potential maximum changes obtained from sensitivity testing using arbitrary afforestation increases. All land-use scenarios showed a summer reduction in peak flows compared to the present land use, generally approx. 4–8%, which may be attributed to the expansion in woodland area (). Surprisingly, for both 10- and 100-year 7-h and 15-h events the NE scenario showed slightly higher reductions than other scenarios; although only a small expansion of coniferous woodland (+4%) was involved in this scenario it was mainly located in the lower part of the catchment close to the Coull reference site. For winter conditions, all scenarios showed reductions in peak flow compared to present land use and with smaller reductions simulated for larger magnitude events; however, larger variations between scenarios were found in a consistent pattern for different magnitude events. Hence, WM has the smallest reductions (2–3%), similar to the NE scenario (2–3%, except 5% reduction for 10-year 7-h events). Global sustainability (GS) has the largest reductions (4–8% depending on size of event), similar to local stewardship (LS: 4–7%), both of these scenarios having larger areal expansions of new woodland.
Table 6. Change in 2050s peak flows at Coull for land-use change scenarios and climate change projections (UKCP09 medium emissions) for the 2050s compared to no land-use change. WM: world markets; NE: national enterprise; GS: global sustainability; LS: local stewardship.
Differences between scenarios can be partially explained by the significantly larger extent of afforestation for GS and LS scenarios compared to smaller changes in NE and WM scenarios. However, differences due to afforestation extent are partly offset because NE and WM scenarios prioritize coniferous afforestation which, as shown by the sensitivity testing, was more effective at reducing peak flows than deciduous woodland (as prioritized in GS and LS scenarios). In relative terms, the results suggest that the much larger expansion of woodland in the GS and LS scenarios is more effective than the type of tree in alleviating winter extreme flows, but that the type and location of tree may be more effective than just large-scale planting in alleviating summer extreme flows. However, similar findings to the sensitivity testing apply in absolute terms, namely that the land-use changes are insufficient by themselves to counter the increase in peak flows due to climate change. This inference is particularly pertinent because of the much lower reductions in peak flows of the scenarios compared to the sensitivity tests.
It should be noted that land-use scenarios do not include the possibility of large increases in coniferous woodland in the Tarland catchment, which would seem to offer the greatest potential reduction in flood risk, because scenario priorities act against this outcome (notably either maximizing agricultural production or delivering environmental stewardship outcomes).
5 Discussion
5.1 Benefits of afforestation for NFM
Greater peak flow reductions were modelled with coniferous compared to deciduous woodland, highlighting differing influences of interception and evaporation of water from the tree canopy. Coniferous trees, with higher overall leaf cover, have higher ET rates than deciduous trees (Cannell Citation1999). Differences in interception between coniferous and deciduous stands can vary by as much as 35%, hence UK coniferous woodland has been estimated to intercept and evaporate 25–45% of total annual precipitation, with the equivalent value for broadleaved woodland being 10–25% (Calder et al. Citation2003). By contrast, although forest transpiration rates are influenced by rooting network, leaf area index, stomatal response, albedo and aerodynamic turbulence, they do not appear to show large variations between deciduous and coniferous trees (Jackson et al. Citation2001).
Modelled reductions in peak flows for coniferous afforestation are consistent with previous research. Fahey and Jackson (Citation1997) reported reduced peak flows of 55–65% from 67% afforestation of a small grassland catchment in comparison to an adjacent control catchment (both 200–300 ha in area). Lane et al. (Citation2005) recorded flow peak reductions of 34–100% based on 10 paired catchments. Similarly, greater modelled peak flow reductions for afforestation on cultivated lowland compared to uncultivated upland is also consistent with previous work (Farley et al. Citation2005). By contrast, there appears less consensus about the effects of broadleaved woodland on catchment peak flows (Roberts and Rosier Citation2005, Calder Citation2007).
Reduced runoff rates from afforestation can also occur through increased infiltration rates associated with improved soil structure and macropore formation (Eldridge and Freudenberger Citation2005). In the present study, this was represented by land-use modifications to reference parameter values for soil hydraulic conductivity based on field data (Archer et al. Citation2013). However, interactions between land use and soil processes are complex and dynamic (Robinson et al. Citation2003, Bens et al. Citation2007, Hümann et al. Citation2011, Archer et al. Citation2013), indicating that further investigation of land-use influence on soil hydraulic properties, including for different woodland types, would be advantageous. Soil hydrology has also been further modified in some locations by the presence of artificial drainage systems to improve agriculture or forest productivity, but data on the type and spacing of drains are often limited (Brown Citation2017). Improved drainage systems to counteract soil waterlogging and promote tree growth are common practice for non-native conifer species in Scotland, although good practice guidelines are now meant to minimize disruption to local hydrology.
Further land-use related modification to runoff processes could occur through altered hydraulic roughness of vegetation, but this was not included in modelling for the present study. Previous studies suggest this could provide additional local benefits from afforestation: for example, Odoni et al. (Citation2010) found that riparian woodland and debris dams could reduce peak flows by 8–10%, whilst Dixon et al. (Citation2016b) inferred reductions of up to 19% in peak flows from riparian woodland.
Differences in results between typical “winter” and “summer” conditions occur because soil storage capacity will generally be greater in summer, when ET rates are higher and water tables are lower, by comparison to winter in Scotland, when soils are typically close to saturation (Brown Citation2017). Seasonal differences are further increased for deciduous trees by reduced interception in winter due to leaf loss. Hence, potential peak flow reduction by afforestation decreases in winter because of less opportunity to divert precipitation away from runoff through alternative hydrological pathways. Consequently, a high proportion of precipitation in impermeable-bedrock catchments such as Tarland becomes surface runoff in winter through saturation excess; this proportion increases further during higher-magnitude precipitation events, as any available storage is soon exceeded. Seasonal distinctions therefore have important implications for the effectiveness of NFM options throughout the year: results indicate a relatively reduced flood alleviation potential in winter following “long-rain” events when most flood events occur. Benefits of afforestation are more apparent during summer when alleviating risks of “flash flood” events from intense “short-rain” convective events due to infiltration excess, but these are rarer events in northeast Scotland.
In addition to peak flow reduction, benefits of afforestation have also been suggested to occur through delays in time taken to reach peak flow. Results from the case study, suggesting an additional delay of 1–2 hours before peak flow, are consistent with assessments from riparian woodland in similar catchments (e.g. Nisbet and Thomas Citation2008). This delay can provide additional time for flood warnings and related risk mitigation measures in downstream locations (e.g. evacuation of high-risk properties). However, modelling also suggested that relatively large increases in afforestation would be required to achieve this goal. Implications of such hydrological changes for inundation extents and land or property at risk would require further assessment using hydraulic modelling.
Regarding distribution of land-use change within the catchment, the main factor identified herein has been that lowland afforestation produced greater reductions of peak flows compared to upland afforestation, as attributed to the reduction in area of arable or improved grassland with lower infiltration capacities. There is also an indication from the NE scenario that siting new woodland closer to flow reference stations, and hence potentially adjacent to locations of high vulnerability, may also reduce peak flow risks if planting is strategically targeted, but again this is apparently beneficial only for rarer summer-type floods.
5.2 Data and model uncertainties
The UKCP09 projections and the HadRM3 climate model ensemble have been used in several previous studies of hydrology and climate change (Cloke et al. Citation2010, Bell et al. Citation2012, Kay and Jones Citation2012, Kay et al. Citation2014a, Citation2014b). Nevertheless, challenges remain with the use of RCM data in hydrological modelling due to uncertainties in parameterization of key physical processes, notably local precipitation patterns (Smith et al. Citation2014). Climate data therefore often represent the dominant source of uncertainty in future projections of fluvial flooding (Najafi et al. Citation2011, Prudhomme et al. Citation2013), which is compounded by the need to both downscale projections to catchment level and to accurately simulate different types of extreme event (Cloke et al. Citation2013).
The present study utilized a stochastic weather generator calibrated against a baseline observed dataset to provide downscaled spatial (5 km) and temporal data (hourly) at a level needed to accurately model changing flood risk in mesoscale catchments. Weather generators assume stationarity of local meteorological processes into the future and may therefore be too conservative in representing climate change dynamics (Dixon et al. Citation2016a). RCMs and GCMs use a more dynamic but much more computationally intensive procedure to evaluate such interactions. Derivation of high-resolution spatial and temporal climate data is therefore currently at the limit of skill for climate change modelling (Chapman et al. Citation2015). As highlighted above, challenges are further exacerbated by difficulties in parameterizing distributed hydrological models, particularly soil and vegetation properties, to represent spatiotemporal variations in hydrological processes. These limitations necessitate caution when interpreting results on the actual magnitude of hydrological change associated with dynamic climate and land-cover parameters. Nevertheless, in relative terms, the benefit of afforestation in reducing flood risk compared to existing land uses appears a reasonably robust outcome. The key issue for NFM would therefore appear to be identifying the scale, type and location of afforestation required to achieve significant advantages for flood risk management, whilst also being cognisant of other societal issues associated with this land-use change.
5.3 Trade-offs and climate change adaptation
Despite the potential for afforestation to reduce flood risk, case study findings also suggest that climate change will continue to increase overall risk levels as heavy precipitation events increase in magnitude. Even large changes in land use seem insufficient to maintain flood risk at a similar level to the present, implying other catchment-based adaptation measures are likely to be required, either structural defences or other nonstructural NFM initiatives (e.g. debris dams, reconnecting floodplains). A more radical alternative would be to accept a higher residual flood risk, which would become the default strategy if improved flood protection, either through NFM or structural defences, was not implemented (Alfieri et al. Citation2016).
The NFM benefits of afforestation appear constrained because of limited capacity to reduce flooding in winter when most large events occur. Even for summer, results suggest afforestation to be more effective at reducing risk for smaller events compared to larger extreme events, consistent with other studies suggesting benefits are greatest for return periods less than 5–10 years and rather smaller for more extreme floods (Beschta et al. Citation2000, Lane et al. Citation2005, Francés et al. Citation2008, Salazar et al. Citation2012). Similarly, research in the Rhine basin (Bronstert et al. Citation2007) identified greater afforestation benefits in alleviating flood risk from extreme convectional short-rain events (typically summer) compared to advective long-rain events, whereas the latter are the dominant risk. Nevertheless, even small contributions to flood alleviation may provide an important contribution to risk management (Van Dijk et al. Citation2009, Bathurst et al. Citation2011). Furthermore, there is some evidence that convective events are increasing in frequency and magnitude due to climate warming, increasing the prevalence of “summer” type risks (Ye et al. Citation2017).
An important issue not generally considered for flood risk alleviation schemes (including NFM) is their potential impact on low flows. Case study results showed that woodland expansion also reduces low-flow discharge and that this is most pronounced for coniferous woodland. A shift in catchment hydrology towards increased forest interception and ET rates has previously been linked with reduced baseflows (Bosch and Hewlett Citation1982, Robinson et al. Citation2003). Sensitivity analysis for an upland catchment in central Wales found a general reduction in water yields of 1.5–2% for every 10% of additional mature coniferous forest (Calder et al. Citation2009). When combined with potential flow reductions due to projected trends towards warmer drier summers in the UK (Christiersen et al. Citation2012), poorly-planned afforestation may exacerbate low-flow problems and cause adverse impacts on aquatic ecology and water quality. An additional factor is that if summer drought risk also increases then some tree species such as Sitka spruce, the current dominant conifer species in Scotland, may become increasingly vulnerable to water stress (Green et al. Citation2008); any resulting physiological damage (e.g. leaf loss) may subsequently disrupt ecohydrological function and hence reduce their flood alleviation role during wetter conditions.
A further reality check is that afforestation is normally more preferred as a land-use option on lower quality land where there is less competition from agriculture (Slee et al. Citation2014). In Scotland, this has led to a preference for new woodland to be planted on uncultivated uplands (Brown et al. Citation2014). A shift towards more lowland afforestation would therefore imply that it is integrated with existing agricultural land uses (e.g. as agroforestry or riparian woodland), which raises further issues regarding the scale of intervention required to significantly alleviate flood risk. As shown by the scenario analysis, “optimal” land-use change to deliver NFM benefits is probably unlikely to be fully realized because of the priority given to other drivers in land-use decisions. In practice, flood risk management decisions are made in a landscape of diverse land uses with differing societal benefits and diverse influences from drivers of change. When aggregated at catchment scale, this typically implies complex trade-offs are required.
Diversity in catchment contexts and trade-offs suggest that, despite the benefits of NFM schemes, it may not be appropriate to consider them as universal “win-win” solutions, particularly with regard to climate change adaptation. Afforestation options evaluated in the case study would involve land-use changes over a significant proportion of the catchment and, in addition to potential trade-offs between low- and high-flow objectives, are likely to have major consequences for other ecosystem services, notably loss of productive agricultural land for crops and livestock (Brown and Castellazzi Citation2014, Collentine and Futter Citation2016). Benefits from new woodland also need to be considered in the context of the growth rates of different types of woodland. The faster growth rates of coniferous trees mean they offer greater benefits for timber production and carbon sequestration, in addition to apparent greater benefits for flood risk alleviation. However, deciduous woodland may be considered more multifunctional due to added benefits for native biodiversity, recreation and amenity value (Brown and Castellazzi Citation2014). Trees, particularly conifers, also significantly increase the risk of transfer of acidifying pollutants from air to soil and surface waters (Cannell Citation1999). Trade-offs and synergies imply that NFM benefits from woodland expansion need to be more explicitly integrated with related initiatives, including land-use planning and water management (Rouillard et al. Citation2015). This would allow NFM concepts to be advanced within the wider context of an integrated catchment management strategy to deliver multiple ecosystem services (Calder and Aylward Citation2006, Iacob et al. Citation2014).
6 Conclusions
Model-based investigation of afforestation NFM options in Tarland catchment (northeast Scotland), employing both sensitivity testing and scenario analysis, has shown it can reduce peak high flows, particularly using coniferous woodland. However, peak flow reductions appear to be less for higher magnitude extreme events and less in typical “winter” UK conditions, when soils are saturated and ET rates lower. This suggests that afforestation-based NFM may have only a limited effect in mitigating the largest extreme flood events that typically occur during the winter.
Catchment afforestation was unable to counteract a general increase in peak flows due to climate change. A possible exception may be provided by full catchment conifer afforestation, but this option is considered implausible because a large proportion of land has high agricultural value. Similarly, although sensitivity analysis showed greater NFM benefits for new woodland replacing cultivated agricultural lowlands, such an “optimal” solution would involve loss of land for crop and livestock production. Scenario analysis showed that complex interactions between land use, climate change and policy drivers mean that optimum land-use configurations for flood risk reduction are unlikely to be achieved. Hydrological modelling also showed that afforestation could reduce low flows, particularly for coniferous woodland, despite it providing the greater benefits for reducing high flows, highlighting that options appraisal needs to consider integrated management of low and high flows in a changing climate.
These findings support the use of afforestation as an important contributor to flood risk management. However, they also identify that it needs to be linked with other risk management measures within an integrated catchment management and climate change adaptation strategy. Furthermore, measures need to be designed to best suit local contexts and priorities rather than assuming a universal “win-win” solution. Risk management strategies may therefore need to include other compatible NFM and structural approaches. A strategic advantage of land-use related NFM options may be that they can help provide a flexible “low regrets” approach to risk management, which can adapt and evolve in the context of changing circumstances and knowledge (Ciullo et al. Citation2017).
Supplemental Material
Download MS Word (444.8 KB)Acknowledgements
The authors acknowledge the advice of the anonymous reviewers in improving the quality of the original manuscript.
Disclosure statement
No potential conflict of interest is reported by the authors.
Supplementary data
Supplementary data for this article can be accessed here.
Additional information
Funding
References
- Alfieri, L., Feyen, L., and di Baldassarre, G., 2016. Increasing flood risk under climate change: a pan-European assessment of the benefits of four adaptation strategies. Climatic Change, 136, 507–521. doi:10.1007/s10584-016-1641-1
- Allan, R.P. and Soden, B.J., 2008. Atmospheric warming and the amplification of precipitation extremes. Science, 321, 1481–1484. doi:10.1126/science.1160787
- Andréassian, V., 2004. Waters and forests: from historical controversy to scientific debate. Journal of Hydrology, 291, 1–27. doi:10.1016/j.jhydrol.2003.12.015
- Archer, N.A.L., et al., 2015. Rainfall infiltration and soil hydrological characteristics below ancient forest, planted forest and grassland in a temperate northern climate. Ecohydrology, 9, 585–600. doi:10.1002/eco.1658
- Archer, D.R., Climent-Soler, D., and Holman, I., 2010. Changes in discharge rise and fall rates applied to impact assessment of catchment land use. Hydrology Research, 41, 13–26. doi:10.2166/nh.2010.092
- Archer, N.A.L., et al., 2013. Soil characteristics and landcover relationships on soil hydraulic conductivity at a hillslope scale: A view towards local flood management. Journal of Hydrology, 497, 208–222. doi:10.1016/j.jhydrol.2013.05.043
- Bathurst, J.C., et al., 2011. Forest impact on floods due to extreme rainfall and snowmelt in four Latin American environments 1: field data analysis. Journal of Hydrology, 400, 281–291. doi:10.1016/j.jhydrol.2010.11.044
- Bell, V.A., et al., 2012. How might climate change affect river flows across the Thames Basin? An area-wide analysis using the UKCP09 Regional Climate Model ensemble. Journal of Hydrology, 442, 89–104. doi:10.1016/j.jhydrol.2012.04.001
- Bens, O., et al., 2007. Water infiltration and hydraulic conductivity in sandy cambisols: impacts of forest transformation on soil hydrological properties. European Journal of Forest Research, 126, 101–109. doi:10.1007/s10342-006-0133-7
- Beschta, R.L., et al., 2000. Peakflow responses to forest practices in the western cascades of Oregon, USA. Journal of Hydrology, 233, 102–120. doi:10.1016/S0022-1694(00)00231-6
- Beven, K., 2012. Rainfall-runoff modelling: the primer. 2nd ed. Chichester, UK: Wiley-Blackwell.
- Beven, K. and Germann, P., 2013. Macropores and water flow in soils revisited. Water Resources Research, 49, 3071–3092. doi:10.1002/wrcr.20156
- Blöschl, G., et al., 2007. At what scales do climate variability and land cover change impact on flooding and low flows? Hydrological Processes, 21, 1241–1247. doi:10.1002/(ISSN)1099-1085
- Bosch, J.M. and Hewlett, J.D., 1982. A review of catchment experiments to determine the effect of vegetation changes on water yield and evapotranspiration. Journal of Hydrology, 55, 3–23. doi:10.1016/0022-1694(82)90117-2
- Bracken, L.J. and Croke, J., 2007. The concept of hydrological connectivity and its contribution to understanding runoff‐dominated geomorphic systems. Hydrological Processes, 21, 1749–1763. doi:10.1002/(ISSN)1099-1085
- Breuer, L., Eckhardt, K., and Frede, H.-G., 2003. Plant parameter values for models in temperate climates. Ecological Modelling, 169, 237–293. doi:10.1016/S0304-3800(03)00274-6
- Bronstert, A., et al., 2007. Multi-scale modelling of land-use change and river training effects on floods in the Rhine basin. River Research and Applications, 23, 1102–1125. doi:10.1002/(ISSN)1535-1467
- Bronstert, A., Niehoff, D., and Bürger, G., 2002. Effects of climate and land-use change on storm runoff generation: present knowledge and modelling capabilities. Hydrological Processes, 16, 509–529. doi:10.1002/hyp.v16:2
- Brown, I., 2017. Climate change and soil wetness limitations for agriculture: spatial risk assessment framework with application to Scotland. Geoderma, 285, 173–184. doi:10.1016/j.geoderma.2016.09.023
- Brown, I. and Castellazzi, M., 2014. Scenario analysis for regional decision-making on sustainable multifunctional land uses. Regional Environmental Change, 14, 1357–1371. doi:10.1007/s10113-013-0579-3
- Brown, I., Castellazzi, M., and Feliciano, D., 2014. Comparing path dependence and spatial targeting of land use in implementing climate change responses. Land, 3, 850–873. doi:10.3390/land3030850
- Brutsaert, W.H., 1982. Evaporation into the atmosphere: theory, history, and applications. Dordrecht: Reidel Publishing.
- Calder, I.R., et al., 2003. Impact of lowland forests in England on water resources: application of the Hydrological Land Use Change (HYLUC) model. Water Resources Research, 39. doi:10.1029/2003WR002040
- Calder, I.R., 2007. Forests and water—ensuring forest benefits outweigh water costs. Forest Ecology and Management, 251, 110–120. doi:10.1016/j.foreco.2007.06.015
- Calder, I.R. and Aylward, B., 2006. Forest and floods: moving to an evidence-based approach to watershed and integrated flood management. Water International, 31, 87–99. doi:10.1080/02508060608691918
- Calder, I.R., Nisbet, T., and Harrison, J.A., 2009. An evaluation of the impacts of energy tree plantations on water resources in the United Kingdom under present and future UKCIP02 climate scenarios. Water Resources Research, 45. doi:10.1029/2007WR006657
- Cannell, M.G., 1999. Environmental impacts of forest monocultures: water use, acidification, wildlife conservation, and carbon storage. New Forestry, 17, 239–262. doi:10.1023/A:1006551018221
- Castellazzi, M.S., et al., 2010. Simulation scenarios of spatio-temporal arrangement of crops at the landscape scale. Environmental Modelling & Software, 25, 1881–1889. doi:10.1016/j.envsoft.2010.04.006
- Chapman, S.C., Stainforth, D.A., and Watkins, N.W., 2015. Limits to the quantification of local climate change. Environmental Research Letters, 10, 094018. doi:10.1088/1748-9326/10/9/094018
- Christiersen, B.V., Vidal, J.-P., and Wade, S.J., 2012. Using UKCP09 probabilistic climate information for UK water resource planning. Journal of Hydrology, 424, 48–67. doi:10.1016/j.jhydrol.2011.12.020
- Ciullo, A., et al., 2017. Socio-hydrological modelling of flood-risk dynamics: comparing the resilience of green and technological systems. Hydrological Sciences Journal, 62, 880–891. doi:10.1080/02626667.2016.1273527
- Cloke, H.L., et al., 2010. Climate impacts on river flow: projections for the Medway catchment UK with UKCIP and CATCHMOD. Hydrological Processes, 24, 3476–3489. doi:10.1002/hyp.7769
- Cloke, H.L., et al., 2013. Modelling climate impact on floods with ensemble climate projections. Quarterly Journal of the Royal Meteorological Society, 139, 282–297. doi:10.1002/qj.1998
- Collentine, D. and Futter, M.N., 2016. Realising the potential of natural water retention measures in catchment flood management: trade-offs and matching interests. Journal of Flood Risk Management. doi:10.1111/jfr3.12269
- Dankers, R. and Feyen, L., 2008. Climate change impact on flood hazard in Europe: an assessment based on high-resolution climate simulations. Journal of Geophysical Research, 113, D19105. doi:10.1029/2007JD009719
- Deasy, C., Titman, A., and Quinton, J., 2014. Measurement of flood peak effects as a result of soil and land management, with focus on experimental issues and scale. Journal of Environmental Management, 132, 304–312. doi:10.1016/j.jenvman.2013.11.027
- Dixon, K.W., et al., 2016a. Evaluating the stationarity assumption in statistically downscaled climate projections: is past performance an indicator of future results? Climatic Change, 135, 395–408. doi:10.1007/s10584-016-1598-0
- Dixon, S.J., et al., 2016b. The effects of river restoration on catchment scale flood risk and flood hydrology. Earth Surface Processes and Landforms, 41, 997–1008. doi:10.1002/esp.v41.7
- Doherty, J. and Skahill, B.E., 2006. An advanced regularization methodology for use in watershed model calibration. Journal of Hydrology, 327, 564–577. doi:10.1016/j.jhydrol.2005.11.058
- Dwarakish, G.S. and Ganesri, B.P., 2015. Impact of land use change on hydrological systems: A review of current modeling approaches. Cogent Geosciences, 1, 1115691. doi:10.1080/23312041.2015.1115691
- Eldridge, D.J. and Freudenberger, D., 2005. Ecosystem wicks: woodland trees enhance water infiltration in a fragmented agricultural landscape in eastern Australia. Austral Ecology, 30, 336–347. doi:10.1111/aec.2005.30.issue-3
- Environment Agency, 2010. Working with natural processes to manage flood and coastal erosion risk: a guidance document. Bristol, UK: Environment Agency.
- Fahey, B. and Jackson, R., 1997. Hydrological impacts of converting native forests and grasslands to pine plantations, South Island, New Zealand. Agricultural and Forest Meteorology, 84, 69–82. doi:10.1016/S0168-1923(96)02376-3
- Farley, K.A., Jobbagy, E.G., and Jackson, R.B., 2005. Effects of afforestation on water yield: a global synthesis with implications for policy. Global Change Biology, 11, 1565–1576. doi:10.1111/gcb.2005.11.issue-10
- Feyen, L., et al., 2012. Fluvial flood risk in Europe in present and future climates. Climatic Change, 112, 47–62. doi:10.1007/s10584-011-0339-7
- Fowler, H.J. and Kilsby, C.G., 2003. A regional frequency analysis of United Kingdom extreme rainfall from 1961 to 2000. International Journal of Climatology, 23, 1313–1334. doi:10.1002/(ISSN)1097-0088
- Francés, F., et al., 2008. Efficiency of non-structural flood mitigationmeasures: ‘room for the river’ and ‘retaining water inthe landscape’. CRUE Research Report No I-6, London, UK. Available from: http://www.crue-eranet.net/Calls/Room_for_the_River_final_report.pdf [Accessed 5 September 2016]
- Gädeke, A., et al., 2014. Analysis of uncertainties in the hydrological response of a model‐based climate change impact assessment in a subcatchment of the Spree River, Germany. Hydrological Processes, 28, 3978–3998. doi:10.1002/hyp.v28.12
- Garner, G., et al., 2015. Hydroclimatology of extreme river flows. Freshwater Biology, 60, 2461–2476. doi:10.1111/fwb.2015.60.issue-12
- Ghavasieh, A.R., Poulard, C., and Paquier, A., 2006. Effect of roughened strips on flood propagation: assessment on representative virtual cases and validation. Journal of Hydrology, 318, 121–137. doi:10.1016/j.jhydrol.2005.06.009
- Gonzales-Sosa, E., et al., 2010. Impact of land use on the hydraulic properties of the topsoil in a small French catchment. Hydrological Processes, 24, 2382–2399.
- Green, S., Hendry, S.J., and Redfern, D.B., 2008. Drought damage to pole-stage Sitka spruce and other conifers in north-east Scotland. Scottish Forestry, 62, 10–18.
- Hess, T.M., et al., 2010. Estimating the impact of rural land management changes on catchment runoff generation in England and Wales. Hydrological Processes, 24, 1357–1368.
- Hirabayashi, Y., et al., 2008. Global projections of changing risks of floods and droughts in a changing climate. Hydrological Sciences Journal, 53, 754–772. doi:10.1623/hysj.53.4.754
- Hölzel, H., Rössler, O., and Diekkrüger, B., 2011. Grope in the Dark–Hydrological modelling of the artificial Chicken Creek catchment without validation possibilities. Physics and Chemistry of the Earth, Parts A/B/C, 36, 113–122. doi:10.1016/j.pce.2010.04.017
- Hümann, M., et al., 2011. Identification of runoff processes–The impact of different forest types and soil properties on runoff formation and floods. Journal of Hydrology, 409, 637–649. doi:10.1016/j.jhydrol.2011.08.067
- Hundecha, Y. and Bárdossy, A., 2004. Modeling of the effect of land use changes on the runoff generation of a river basin through parameter regionalization of a watershed model. Journal of Hydrology, 292, 281–295. doi:10.1016/j.jhydrol.2004.01.002
- Iacob, O., et al., 2014. Evaluating wider benefits of natural flood management strategies: an ecosystem-based adaptation perspective. Hydrology Research, 45, 774–787. doi:10.2166/nh.2014.184
- Institute of Hydrology, 1999. Flood estimation handbook, 5 volumes. Wallingford, UK: Centre for Ecology and Hydrology.
- IPCC (Intergovernmental Panel on Climate Change), 2012. Managing the risks of extreme events and disasters to advance climate change adaptation. Cambridge, UK: Cambridge University Press.
- Ivancic, T.J. and Shaw, S.B., 2015. Examining why trends in very heavy precipitation should not be mistaken for trends in very high river discharge. Climatic Change, 133, 681–693. doi:10.1007/s10584-015-1476-1
- Jackson, R.B., et al., 2001. Water in a changing world. Ecological Applications, 11, 1027–1045. doi:10.1890/1051-0761(2001)011[1027:WIACW]2.0.CO;2
- Jarvis, N., et al., 2013. Influence of soil, land use and climatic factors on the hydraulic conductivity of soil. Hydrology and Earth System Sciences, 17, 5185–5195. doi:10.5194/hess-17-5185-2013
- Jasper, K., et al., 2004. Differential impacts of climate change on the hydrology of two alpine river basins. Climate Research, 26, 113–129. doi:10.3354/cr026113
- Jirků, V., et al., 2013. Temporal variability of structure and hydraulic properties of topsoil of three soil types. Geoderma, 204, 43–58. doi:10.1016/j.geoderma.2013.03.024
- Jones, P.D., et al., 2009. UK climate projections science report: projections of future daily climate for the UK from the weather generator. Newcastle, UK: University of Newcastle.
- Jongman, B., Ward, P.J., and Aerts, J.C.J.H., 2012. Global exposure to river and coastal flooding: long term trends and changes. Global Environmental Change, 22, 823–835. doi:10.1016/j.gloenvcha.2012.07.004
- Jost, G., et al., 2012. A hillslope scale comparison of tree species influence on soil moisture dynamics and runoff processes during intense rainfall. Journal of Hydrology, 420-421, 112–124. doi:10.1016/j.jhydrol.2011.11.057
- Kay, A.L., et al., 2014a. Probabilistic impacts of climate change on flood frequency using response surfaces I: England and Wales. Regional Environmental Change, 14, 1215–1227. doi:10.1007/s10113-013-0563-y
- Kay, A.L., et al., 2014b. Probabilistic impacts of climate change on flood frequency using response surfaces II: Scotland. Regional Environmental Change, 14, 1243–1255. doi:10.1007/s10113-013-0564-x
- Kay, A.L. and Jones, D.A., 2012. Transient changes in flood frequency and timing in Britain under potential projections of climate change. International Journal of Climatology, 32, 489–502. doi:10.1002/joc.v32.4
- Kundzewicz, Z.W., et al., 2016. Differences in flood hazard projections in Europe–their causes and consequences for decision making. Hydrological Sciences Journal, 62, 1–14.
- Lane, P.N.J., et al., 2005. The response of flow duration curves to afforestation. Journal of Hydrology, 310, 253–265. doi:10.1016/j.jhydrol.2005.01.006
- Lane, S.N., et al., 2007. Interactions between sediment delivery, channel change, climate change and flood risk in a temperate upland environment. Earth Surface Processes and Landforms, 32, 429–446. doi:10.1002/(ISSN)1096-9837
- Lane, S.N. and Milledge, D.G., 2013. Impacts of upland open drains upon runoff generation: a numerical assessment of catchment‐scale impacts. Hydrological Processes, 27, 1701–1726. doi:10.1002/hyp.v27.12
- Lange, B., Lüescher, P., and Germann, P.F., 2009. Significance of tree roots for preferential infiltration in stagnic soils. Hydrology and Earth System Sciences, 13, 1809–1821. doi:10.5194/hess-13-1809-2009
- Lawrence, J., et al., 2013. Exploring climate change uncertainties to support adaptive management of changing flood-risk. Environmental Science & Policy, 33, 133–142. doi:10.1016/j.envsci.2013.05.008
- Lehmann, J., Coumou, D., and Frieler, K., 2015. Increased record-breaking precipitation events under global warming. Climatic Change, 132, 501–515. doi:10.1007/s10584-015-1434-y
- Ludwig, J.A., et al., 2005. Vegetation patches and runoff–erosion as interacting ecohydrological processes in semiarid landscapes. Ecology, 86, 288–297. doi:10.1890/03-0569
- Marshall, M.R., et al., 2014. The impact of rural land management changes on soil hydraulic properties and runoff processes: results from experimental plots in upland UK. Hydrological Processes, 28, 2617–2629. doi:10.1002/hyp.9826
- McVicar, T.R., et al., 2007. Developing a decision support tool for China’s re-vegetation program: simulating regional impacts of afforestation on average annual streamflow in the Loess Plateau. Forest Ecology and Management, 25, 65–81. doi:10.1016/j.foreco.2007.06.025
- Merz, B., et al., 2010. Fluvial flood risk management in a changing world. Natural Hazards and Earth Systems Science, 10, 509–527. doi:10.5194/nhess-10-509-2010
- Merz, R. and Blöschl, G., 2003. A process typology of regional floods. Water Resources Research, 29, 1340.
- Milly, P., et al., 2002. Increasing risk of great floods in a changing climate. Nature, 415, 514–517. doi:10.1038/415514a
- Milly, P.C.D., et al., 2008. Stationarity is dead: whither water management? Science, 319, 573–574. doi:10.1126/science.1151915
- Monteith, J.L., 1975. Vegetation and the Atmosphere. Vol. 1. Principles. London: Academic Press.
- Morton, D., et al., 2011. Countryside Survey: Final Report for LCM2007- the new UK Land Cover Map. Lancaster, UK: Centre for Ecology & Hydrology.
- Murphy, J.M., et al., 2009. UK climate projections science report: climate change projections. Exeter, UK: Met Office Hadley Centre.
- Najafi, M.R., Moradkhani, H., and Jung, I.W., 2011. Assessing the uncertainties of hydrologic model selection in climate change impact studies. Hydrological Processes, 25, 2814–2826. doi:10.1002/hyp.v25.18
- Nash, J.E. and Sutcliffe, J.V., 1970. River flow forecasting through conceptual models. Part I: A discussion of principles. Journal of Hydrology, 10, 282–290. doi:10.1016/0022-1694(70)90255-6
- Niehoff, D., Fritsch, U., and Bronstert, A., 2002. Land-use impacts on storm-runoff generation: scenarios of land-use change and simulation of hydrological response in a meso-scale catchment in SW-Germany. Journal of Hydrology, 267, 80–93. doi:10.1016/S0022-1694(02)00142-7
- Nijnik, M., Nijnik, A., and Brown, I., 2016. Exploring the linkages between multi-functional forestry goals and the legacy of spruce plantations in Scotland. Canadian Journal of Forest Research, 46, 1247–1254. doi:10.1139/cjfr-2015-0399
- Nisbet, T.R. and Thomas, H., 2008. Restoring floodplain woodland for flood alleviation. Final Report. Defra, London, UK. Available from: http://www.forestry.gov.uk/pdf/defra_floodplain_report_15june2008.pdf/ [Accessed 5 April 2016]
- O’Connell, P.E., et al., 2007. Is there a link between agricultural land-use management and flooding? Hydrology and Earth System Sciences, 11, 96–107. doi:10.5194/hess-11-96-2007
- Odoni, N.A., and Lane, S.N., 2010. Assessment of the impact of upstream land management measures on flood flows in Pickering Beck using overflow. In: Project RMP55455: Slowing the flow at Pickering, Appendix 12.2. Available from: https://www.forestry.gov.uk/fr/infd-7zucqy [Accessed 3 May 2016]
- Orr, H., et al., 2008. Climate change in the uplands: a UK perspective on safeguarding regulatory ecosystem services. Climate Research, 37, 77–98. doi:10.3354/cr00754
- Parrott, A., et al., 2009. Role of rural land use management in flood and coastal risk management. Journal of Flood Risk Management, 2, 272–284. doi:10.1111/jfrm.2009.2.issue-4
- Pattison, I. and Lane, S.N., 2012. The link between land-use management and fluvial flood risk: A chaotic conception? Progress in Physical Geography, 36, 72–92. doi:10.1177/0309133311425398
- Peng, S.L., Wu, J., and You, W.H., 2012. Recovery of saturated hydraulic conductivity along a forest successional series from abandoned land to mature, evergreen broad-leaved forest in eastern China. Soil Research, 50, 257–266. doi:10.1071/SR11149
- Perry, M. and Hollis, D., 2005. The generation of monthly gridded datasets for a range of climatic variables over the UK. International Journal of Climatology, 25, 1041–1054. doi:10.1002/(ISSN)1097-0088
- Prudhomme, C., et al., 2013. Climate change and river flooding: part 2 sensitivity characterisation for British catchments and example vulnerability assessments. Climatic Change, 119, 949–964. doi:10.1007/s10584-013-0726-3
- Richards, L.A., 1931. Capillary conduction of liquids through porous mediums. Physics, 1, 318–333. doi:10.1063/1.1745010
- Roberts, J. and Rosier, P., 2005. The impact of broadleaved woodland on water resources in lowland UK: I. Soil water changes below beech woodland and grass on chalk sites in Hampshire. Hydrology and Earth System Sciences, 9, 596–606. doi:10.5194/hess-9-596-2005
- Robinson, M., et al., 2003. Studies of the impact of forests on peak flows and baseflows: a European perspective. Forest Ecology and Management, 186, 85–97. doi:10.1016/S0378-1127(03)00238-X
- Rouillard, J.J., et al., 2015. Policy implementation of catchment-scale flood risk management: learning from Scotland and England. Environmental Science & Policy, 50, 155–165. doi:10.1016/j.envsci.2015.02.009
- Rouquette, J.R., et al., 2011. Synergies and trade-offs in the management of lowland rural floodplains: an ecosystem services approach. Hydrological Sciences Journal, 56, 1566–1581. doi:10.1080/02626667.2011.629785
- Salazar, S., et al., 2012. A comparative analysis of the effectiveness of flood management measures based on the concept of “retaining water in the landscape” in different European hydro-climatic regions. Natural Hazards and Earth System Science, 12, 3287–3306. doi:10.5194/nhess-12-3287-2012
- Sayers, P., et al., 2014. Strategic flood management: ten ‘golden rules’ to guide a sound approach. International Journal of River Basin Management, 13, 137–151. doi:10.1080/15715124.2014.902378
- Schaap, M.G., Leij, F.J., and van Genuchten, M.T., 2001. Rosetta: a computer program for estimating soil hydraulic parameters with hierarchical pedotransfer functions. Journal of Hydrology, 251, 163–176. doi:10.1016/S0022-1694(01)00466-8
- Schulla, J. and Jasper, K., 2007. Model description WaSiM-ETH. Zürich: Institute for Atmospheric and Climate Science, Swiss Federal Institute of Technology.
- Schwärzel, K., Ebermann, S., and Schalling, N., 2012. Evidence of double funnelling effect of beech trees by visualization of flow pathways using dye tracer. Journal of Hydrology, 470, 184–192. doi:10.1016/j.jhydrol.2012.08.048
- Scotland’s Soils, 2016. National Soil Inventory of Scotland. Available from: http://www.soils-scotland.gov.uk/data/nsis [Accessed 12 June 2016]
- Singh, S.K., Liang, J., and Bárdossy, A., 2012. Improving the calibration strategy of the physically-based model WaSiM-ETH using critical events. Hydrological Sciences Journal, 57, 1487–1505. doi:10.1080/02626667.2012.727091
- Slee, B., et al., 2014. The Squeezed Middle: identifying and addressing conflicting demands on intermediate quality farmland. Land Use Policy, 41, 206–216. doi:10.1016/j.landusepol.2014.06.002
- Smith, A., et al., 2014. Investigating the application of climate models in flood projection across the UK. Hydrological Processes, 28, 2810–2823. doi:10.1002/hyp.v28.5
- Steele-Dunne, S., et al., 2008. The impacts of climate change on hydrology in Ireland. Journal of Hydrology, 356, 28–45. doi:10.1016/j.jhydrol.2008.03.025
- Svensson, C. and Jones, D.A., 2010. Review of rainfall frequency estimation methods. Journal of Flood Risk Management, 3, 1–33. doi:10.1111/j.1753-318X.2010.01079.x
- Thomas, H. and Nisbet, T.R., 2006. An assessment of the impact of floodplain woodland on flood flows. Water and Environment Journal, 21, 114–126. doi:10.1111/j.1747-6593.2006.00056.x
- van Dijk, A.I.M., et al., 2009. Forest–flood relation still tenuous. Comment on ‘Global evidence that deforestation amplifies flood risk and severity in the developing world. Global Change Biology, 15, 110–115. doi:10.1111/j.1365-2486.2008.01708.x
- Van Genuchten, M.T., 1980. A closed-form equation for predicting the hydraulic conductivity of unsaturated soils. Soil Science Society of America Journal, 44, S.892-898. doi:10.2136/sssaj1980.03615995004400050002x
- Verbunt, M., Zwaaftink, M.G., and Gurtz, J., 2005. The hydrologic impact of land cover changes and hydropower stations in the Alpine Rhine basin. Ecological Modelling, 187, 71–84. doi:10.1016/j.ecolmodel.2005.01.027
- Vereecken, H., et al., 2010. Using pedotransfer functions to estimate the van Genuchten-Mualem soil hydraulic properties: a review. Vadose Zone Journal, 9, 795–820. doi:10.2136/vzj2010.0045
- Vogel, R.M. and Fennessey, N.M., 1994. Flow-duration curves. I: new interpretation and confidence intervals. Journal of Water Resources Planning and Management, 120, 485–504. doi:10.1061/(ASCE)0733-9496(1994)120:4(485)
- Werritty, A., et al., 2006. Use of proxy flood records to improve estimates of flood risk: lower Tay, Scotland. Catena, 66, 107–119. doi:10.1016/j.catena.2005.07.012
- Whitfield, P.H., 2012. Floods in future climates: a review. Journal of Flood Risk Management, 5, 336–365. doi:10.1111/jfr3.2012.5.issue-4
- Wilby, R.L., Beven, K.J., and Reynard, N.S., 2008. Climate change and fluvial flood risk in the UK: more of the same? Hydrological Processes, 22, 2511–2523. doi:10.1002/hyp.v22:14
- Wriedt, G. and Rode, M., 2006. Investigation of parameter uncertainty and identifiability of the hydrological model WaSiM-ETH. Advances in Geosciences, 9, 145–150. doi:10.5194/adgeo-9-145-2006
- Ye, H., et al., 2017. Rapid decadal convective precipitation increase over Eurasia during the last three decades of the 20th century. Science Advances, 3, e1600944. doi:10.1126/sciadv.1600944