ABSTRACT
Hyporheic exchange induced by dunes is a key process controlling water fluxes and biogeochemical processes in river networks. Owing to the limitations of instrumental detection at small spatial scales, previous studies have focused mainly on dune-induced hyporheic exchange in homogeneous systems. A low-permeability lens is a natural, widespread heterogeneity in stream beds, and probably affects the processes of water flow and contaminant transportation significantly. To quantitatively analyse the response mechanism of hyporheic exchange to a low-permeability lens, a two-dimensional dune-generated hyporheic exchange model was developed using the VS2DH model. The results indicate a lens in a stream bed can hinder or enhance hyporheic exchange processes, depending on its relative spatial location to dunes. Both the increase in length and thickness of the lens could strengthen its impacts on hyporheic exchange. Regional groundwater–surface water interactions of higher intensity suppress the flow of hyporheic exchange in a stream bed with a low-permeability lens.
Editor A. Castellarin Associate editor A. Fiori
Introduction
Hyporheic exchange, a ubiquitous component of groundwater–surface water (GW–SW) interactions, is defined as the movement of surface water across the bed and banks of streams into shallow subsurface flow paths that then return to the stream (Winter et al. Citation1998). This type of GW–SW exchange plays a fundamental role in contaminant transport and remediation, water resources management, and the functioning of aquatic ecosystems (Sophocleous Citation2002, Boulton et al. Citation2010, Hester and Gooseff Citation2010, Krause et al. Citation2013). Investigating the mechanisms that drive hyporheic exchange is of great importance to improve the understanding of the physical, biogeochemical and thermal processes in streams, hyporheic zones and aquifers (Cardenas and Wilson Citation2007a, Citation2007b, Jin et al. Citation2011).
One fundamental mechanism of hyporheic exchange is the presence of pressure gradients at the stream bed surface generated by surface water flowing over stream bed geomorphologic features (Jin et al. Citation2010, Bhaskar et al. Citation2012, Boano et al. Citation2015). Different types of geomorphologic features can induce hyporheic exchange of specific spatial scales (Boano et al. Citation2015). Small bedforms, such as ripples and dunes, typically induce hyporheic flow ranging from centimetres to metres (e.g. Elliott and Brooks Citation1997a, Citation1997b, Packman and Brooks Citation2001, Bottacin-Busolin and Marion Citation2010). Exchanges generated by sediment bars have typical lengths of approximately 10–100 m (e.g. Tonina and Buffington Citation2007, Marzadri et al. Citation2010). For larger geomorphologic features such as meander bends, the induced hyporheic flow could have longer flow paths of hundreds or even thousands of metres (e.g. Peterson and Sickbert Citation2006, Revelli et al. Citation2008, Gomez et al. Citation2012).
Among all scales of geomorphologic features, bedform size is often chosen to be the fundamental length scale, which is consistent with the scales associated with many environmental and ecological problems, where the most frequent typical depths associated with benthic nutrient uptake, contaminant transport, roots of aquatic plants, and aquatic animal activity range from a few centimetres to one metre (Jones and Mulholland Citation2000, Boano et al. Citation2015). In addition, bedforms are often the fundamental unit for physically-based modelling and experimental investigations of hyporheic exchange (Thibodeaux and Boyle Citation1987, Salehin et al. Citation2004, Boano et al. Citation2015). In this study, we focus on hyporheic exchange induced by small bedforms and choose dunes as a representative feature to study physical processes and mechanisms of hyporheic flow influenced by the presence of low-permeability lenses.
Previous studies have made significant advances in our understanding of dune-induced hyporheic exchange by conducting laboratory experiments and numerical simulations. Elliott and Brooks (Citation1997a, Citationb) provided analytical approximations of velocity fields, residence times and net mass exchange in the hyporheic zone generated by dunes. Cardenas and Wilson (Citation2006, Citation2007b) analysed hyporheic flow process induced by dunes with turbulent surface flow using a multiphysics numerical modelling approach. Jin et al. (Citation2010) studied solute transport processes in a stream bed with dunes by combining laboratory experiments and numerical simulations. Apart from physical and biogeochemical processes, many studies have explored internal or external factors on the hyporheic process generated by dunes, such as dune geometry (Cardenas and Wilson Citation2007b) and regional GW–SW exchange (regional groundwater recharge or discharge) (Cardenas and Wilson Citation2006, Citation2007a, Boano et al. Citation2008, Citation2009).
Despite significant advances, most dune-induced hyporheic process studies have focused on homogeneous systems. However, the heterogeneity of stream-bed sediments is recognized to have significant effects on subsurface flow processes and solute transport (Gerber et al. Citation2001, Landon et al. Citation2001, Freitas et al. Citation2015, Wu et al. Citation2015). Some studies have examined the impact of heterogeneity on the hyporheic process at larger spatial scales (Kasahara and Wondzell Citation2003, Storey et al. Citation2003). Owing to the limitation of instrumental detection, few studies have considered heterogeneity at smaller spatial scales (Bridge Citation2003, Cardenas et al. Citation2004, Hester et al. Citation2013, Tonina et al. Citation2016). According to the detailed hydrogeological studies of Jankowski and Beck (Citation2000), even at small scales, hydraulic conductivity (K) can vary by orders of magnitude. The field tracer tests of Wagner and Bretschko (Citation2002) suggests that bed-scale variability of K can result in a complex three-dimensional (3-D) network of flow paths, and heterogeneity was attributed to the patchy distribution of benthic invertebrates. Cardenas et al. (Citation2004) reported that residence times of hyporheic flow can decrease or increase in the presence of heterogeneity, depending on the relative positions of the heterogeneities and the bedforms. In this study, we consider the occurrence of a low-permeability lens, one type of natural heterogeneity in stream beds, and explore the impact of such a feature on the hyporheic process.
In natural streams, the components of stream-bed sediments are complex. In a coarse-grained sedimentary environment, hydrological and ecological processes can be significantly influenced by the presence of fine-grained sediments with low permeability (Faisal et al. Citation2011, Karwan and Saiers Citation2012). Fine-grained sediments are important for watershed biogeochemical cycling and stream metabolic processes but pose a risk to water quality if present in excess, or if carrying sorbed contaminants (Karwan and Saiers Citation2012). To better analyse the effect of low-permeability sediments on hyporheic exchange, we focus on low-permeability lens features in this study. Such features are widespread in natural stream beds. Wu et al. (Citation2015, Citation2016) monitored the distribution of silt/clay lenses in the stream bed of the Dawen River, China, and analysed the variation of low-permeability lenses before and after a flood season. Studies have shown that heterogeneous porous media with low-permeability lenses can produce contaminant plumes with extended tails (Guswa and Freyberg Citation2000). Research has suggested that in heterogeneous sediments the transportation of aqueous solute (Bosch et al. Citation2001, Milosevic et al. Citation2012) and non-aqueous solute (Rathfelder et al. Citation2001, Reynolds and Kueper Citation2001, Faisal et al. Citation2011) are both controlled by the presence of low-permeability lenses. Gomez-Velez et al. (Citation2014) studied the impacts of low-permeability layers on spatial patterns of hyporheic exchange and groundwater upwelling. However, no studies have revealed the impact of low-permeability lenses with varying sizes and locations on the hyporheic process. In this study, a single low-permeability lens was considered to better reveal the mechanism of hyporheic flow under the control of low-permeability lenses.
In this study, we focus on the hyporheic process at the scale of a bedform, aiming to uncover the impact of a low-permeability lens on dune-induced hyporheic exchange, thus contributing to a better understanding of the relationship between heterogeneity at small spatial scales and GW–SW interactions. To quantitatively analyse the response mechanism of hyporheic exchange to a low-permeability lens, a two-dimensional (2-D) dune-generated hyporheic exchange model was built based on the US Geological Survey model, VS2DH (Healy Citation1990, Hsieh et al. Citation2000, Healy and Ronan Citation1996). One single low-permeability lens of varying size and location was imbedded in the stream bed to study its effects on the hyporheic process. According to Fox et al. (Citation2014) and Gariglio et al. (Citation2013), regional GW–SW interactions (also called regional groundwater in this study) could weaken the hyporheic process to some extent. This study also considers whether the influence of a low-permeability lens on hyporheic exchange varies under different streamflow conditions (neutral, losing and gaining). In summary, we address the following two questions in this study: (1) How do hyporheic flow paths and exchange intensity respond to the presence of a low-permeability lens of varying size and location? And (2) What are the effects of regional GW–SW interactions on the aforementioned responding mechanism?
Methodology
Theoretical background
Dunes are significant sources of driving forces for hyporheic exchange, having varying pressure along their surface (Bhaskar et al. Citation2012, Stonedahl et al. Citation2013). According to Elliott and Brooks (Citation1997a, Citation1997b), the hyporheic flow generated by natural triangular dunes could be estimated by that induced by a flat bed with a sinusoidal distribution of pressure (Fig. 1(a)). Thus, dunes in a natural stream bed could be imitated by a flat bed with a sinusoidal pressure profile in a numerical model. This method has been applied in many studies. For example, Boano et al. (Citation2008, Citation2009) analysed the influence of groundwater discharge on bedform-induced hyporheic exchange and derived an equation to estimate the depth of the hyporheic zone based on the assumption of a sinusoidal pressure profile at the stream-bed surface. Fox et al. (Citation2016) built a numerical flow model to analyse the effect of losing and gaining conditions on hyporheic exchange in heterogeneous stream beds, whose boundary condition at the top was defined by the sinusoidal pressure distribution calculated according to Elliott and Brooks (Citation1997a, Citationb). This study also applies a sinusoidal varying pressure profile to imitate the interface of the stream bed with dunes.
shows the dunes in a natural stream bed (yellow line). According to the simulated results of Cardenas and Wilson (Citation2007a, Citation2007b), surface water flows into the stream bed from the upstream face of the dunes and then flows out of the stream-bed surface at points near the crest of the dunes (shown as in Cardenas and Wilson Citation2007a, Citationb). Thus the spatial distribution of the pressure (h) along the flat bed that imitates the natural dunes could be inferred (black line in )), with the minimum value located at the crest of the dunes (shown as the black triangle) and the maximum value at some location in the upstream surface (shown as the black diamond). It is the spatial distribution of h that mainly dominates the hyporheic flow process, which is symmetrical and periodic, thus the location of the intermediate crest of h (black curve in )) was set as the origin point of the coordinate system in this study, instead of the trough of the dunes.
Figure 1. Conceptual illustrations of: (a) theory of Elliott and Brooks (Citation1997a, Citationb); (b)–(d) hyporheic flow (blue dotted line) field and groundwater flow (black dotted line) field beneath the stream bed with dunes under different streamflow conditions (no recharge or discharge, recharge in a losing stream and groundwater discharge in a gaining stream, respectively). Black solid lines in (a) represent the distribution of sinusoidal varied pressures. The black arrows show the flow direction of surface water.
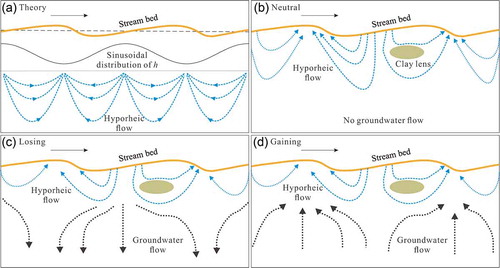
According to Elliott and Brooks (Citation1997a, Citationb), the pressure variation is sinusoidal. Because the wavelength of the dunes in this study is equal to 1.00 m, the distribution of h can be given by:
where hm is the amplitude of the pressure variation (total variation = 2hm) and k is the wavenumber of the variation (, where
is the wavelength of dunes). The value of hm can be derived from an empirically based equation as follows:
where U is the mean stream velocity; H/d is the ratio of dune height to mean water depth and g is gravitational acceleration. Apart from the local morphology of the stream bed (such as dunes in this study), which drives hyporheic exchange, the flow beneath a stream bed is also controlled by the regional forcing of a large-scale hydraulic gradient, which results in losing or gaining streamflow conditions (Fox et al. Citation2014, Marzadri et al. Citation2016). In addition, regional GW–SW interactions could weaken the hyporheic flow (Gariglio et al. Citation2013), as shown in . This study considers three kinds of streamflow conditions: no recharge and discharge (neutral), surface water recharge groundwater (losing) and surface water discharge groundwater (gaining). The existence of a low-permeability lens could alter the flow fields of hyporheic exchange and groundwater flow beneath the stream bed, although the detailed influence of a lens on hyporheic exchange is still unknown.
Numerical simulation
To reveal the response mechanism of hyporheic exchange to a low-permeability lens, a hypothetical model of dune-induced hyporheic flow was built based on the VS2DH model, a 2-D finite difference model that simulates heat transport and water flow in variably saturated sediments (Healy and Ronan Citation1996) (Fig. 2). Model implementation and post-processing are performed using MATLAB. The pressure head distribution needed to calculate groundwater velocity in the vadose zone was determined from the Richards equation, as follows:
where h is the water pressure head, K(h) is the hydraulic conductivity, and C(h) is the specific moisture capacity. In this model, K(h) was constant along the simulation period and C(h) = 0.
The simulated domain of the model represents a stream-bed cross-section that is oriented along the longitudinal direction of the river with a size of 1.00 m × 2.00 m. The flat upper boundary was assigned to be a constant head boundary with a sinusoidal varying pressure, as shown in , which imitates the surface of the stream bed with dunes. According to the conceptual illustrations of dunes and the corresponding sinusoidal pressure in ), the upper boundary of this hypothetical model consists of one dune (length: 1 m) in the middle of the domain and two half dunes on the left and right sides of the domain. In this study, the crest of the pressure profile, where surface water flows vertically into the stream bed, is termed the source point. Similarly, the trough of pressure, where hyporheic exchange flows vertically out of the stream bed, is called the sink point (see ). The left and right sides of the model are both beneath source points, thus the boundary conditions were assigned to be no-flow boundaries. The bottom boundary was assigned to be a constant flow boundary, where groundwater flows in or out of the domain. Three types of streamflow conditions were considered in this study according to the flux of groundwater flow (qG): (neutral),
(losing) and
(gaining).
Figure 2. Domain of a numerical model for 2-D dune-induced hyporheic flow. The intervals of vertical and horizontal grid lines were set to 5 mm. The ellipses represent low-permeability lenses. The coordinate system comprises x and z directions, where x represents horizontal location and z the depth of the low-permeability lens. The location of the intermediate source point was set as the origin point (x = 0, z = 0) and the positive directions in x and z were set to be right and downward, respectively. Diamonds represent the distribution of spatial locations of low-permeability lenses.
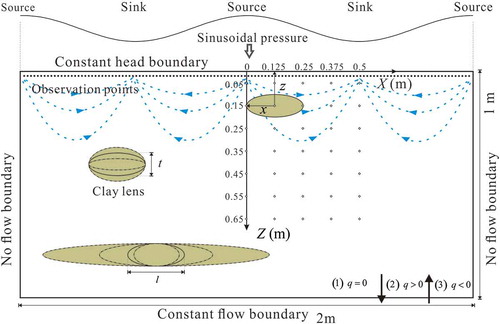
One single low-permeability lens with varying spatial location and size was embedded beneath the stream bed. Because of the symmetry and periodicity of the pressure distribution, the horizontal location of the low-permeability lens varies from x = 0 m to x = 0.5 m (i.e. from source point to sink point), with an interval of 0.125 m. The depth of the low-permeability lens changes from 0.05 m to 0.65 m, with an interval of 0.10 m. At each spatial location, the size of the low-permeability lens (length and thickness) was changed to investigate its impact on hyporheic flow, as shown in (l and t represent length and thickness, respectively). The length and thickness of the low-permeability lens are shown in .
Table 1. Length (l) and thickness (t) of the low-permeability lenses in the numerical experiments.
The hydraulic conductivity of the stream-bed sediments was set at 6.526 m d−1 to represent a sand texture (Bhaskar et al. Citation2012). Considering the low permeability of the lens, the hydraulic conductivity was set at a minimum value of 5.56 × 10–7 m d−1, which could be treated as impermeable in the model. The porosity of the stream bed was set at 0.325. The ratio of the height of the dunes to the mean water depth (H/d) and the mean stream velocity (U) were set at 0.2 and 12.9 cm s−1, respectively, according to Elliott and Brooks (Citation1997a). Thus, from Equation (2), the amplitude of the pressure variation (hm) could be calculated to be 0.0196 cm. The seepage velocities of the grids at the upper boundary were applied to calculate the hyporheic exchange. The x- and z-directional seepage velocity at each grid could be simulated by using the VS2DH model. The total seepage velocities at the bottom boundary were treated as regional groundwater flux (qG).
Calculation of hyporheic exchange
When there is no regional GW–SW interaction, the total flux flows (qT) into the simulated domain through the stream-bed interface equal the flux flowing out of the domain through the interface (Fig. 3(a)), which also equals the hyporheic exchange flux (qhyp) that is generated by the presence of dunes. However, when regional groundwater exists, taking ) as an example, the total flux flowing into the domain through the stream-bed surface equals the sum of the flux flowing out of the domain through the stream-bed interface and the flux flowing out of the bottom boundary of the domain (i.e. regional groundwater, qG) (Boano et al. Citation2008, Hester et al. Citation2013, Fox et al. Citation2014, Marzadri et al. Citation2016). Hyporheic exchange refers to the water flux that moves from the surface water into the subsurface stream bed and then returns to the stream. In losing conditions, the downward flux moving from the surface water is induced by two types of driving functions: local dunes that generate hyporheic exchange flux and a regional hydraulic gradient that results in regional groundwater flux (Boano et al. Citation2008, Hester et al. Citation2013, Fox et al. Citation2014, Marzadri et al. Citation2016). The former flows out of the stream-bed interface and return to surface water, while the latter flows out of the bottom boundary of the domain. Thus, under losing conditions, hyporheic exchange equals the upward flux that flows out of the domain through the interface of the stream bed ()). Under gaining conditions, the upward flux moving from the stream bed consists of hyporheic exchange flux and regional groundwater flux; thus hyporheic exchange equals the downward flux that flows into the domain through the interface of the stream bed ()).
Figure 3. Schematic of flux flows in and out of the simulated domain at (a) neutral condition, (b) losing condition and (c) gaining condition. The solid arrow refers to hyporheic exchange flux and the dashed arrow represents regional groundwater flux.
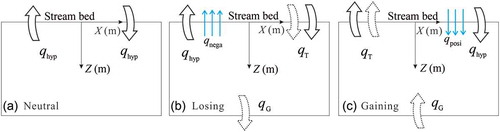
The sum of downward flux and upward flux flowing through the interface can be estimated by adding up all the z-directional positive seepage velocities and negative seepage velocities of each grid, respectively. In the case of a losing stream, the estimated hyporheic exchange qhyp was calculated by the sum of upward flux, i.e. the z-directional negative seepage velocities (qneg). In the case of a stream receiving groundwater inflow, qhyp equals the sum of z-directional positive seepage velocities (qpos). When there is no regional groundwater, hyporheic exchange can be estimated by either the sum of qneg or the sum of qpos as follows:
To evaluate the effect of a low-permeability lens on hyporheic flow processes, the ratio (λ) of hyporheic exchange under the control of a low-permeability lens to that without a lens (q0) was calculated according to:
Obviously, if λ > 1.00, it means that the existence of a low-permeability lens promotes hyporheic exchange.
Results
Intensity of hyporheic exchange
Impact of the location of a low-permeability lens
First, the regional GW–SW interaction qG was set at –0.001 m d−1 to study the impact of varying spatial locations and sizes of the low-permeability lens on hyporheic exchange. To investigate the effects of spatial locations, the length and thickness of the low-permeability lens were assigned as 0.25 m and 0.1 m, respectively. The variations of λ with different horizontal locations and depths are shown in Figure 4. Keeping the depth of the low-permeability lens constant and moving the lens from x = 0 m to x = 0.5 m, λ shows an increasing trend ()), which means the hindering effect of the low-permeability lens on hyporheic exchange weakens or even turns into an enhancement effect as the horizontal location varies from source point to sink point. In addition, λ has great variation in the horizontal direction when the low-permeability lens is at a shallow depth such as 0.05 m. While with increasing depth, the variation decreases gradually and tends to be zero at a relatively deep depth, for example 0.55 m. This suggests depth dependence of the influential intensity for the horizontal location in the hyporheic exchange process. To be specific, the impact of horizontal location decreases with increasing depth.
Figure 4. Variation in λ with (a) horizontal location and (b) depth of the low-permeability lens. The dotted line (λ = 1.00) divides two types of effect on hyporheic exchange generated by the low-permeability lens: hindering below the line and enhancement when λ > 1.00.
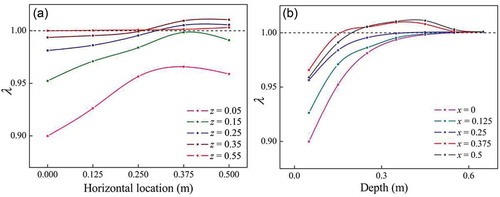
Fixing the horizontal location of the low-permeability lens and gradually increasing the depth of the lens, the dynamic process of λ occurs as shown in ). Generally, λ increases when the vertical location of the lens changes from 0.05 to 0.6 m in depth. After this, it stays nearly constant and equals approx. 1.00. It can also be seen from that the changing patterns of λ with increasing depth are related to different horizontal locations. Specifically, when the low-permeability lens is at the source zone (x = 0, 0.125 and 0.25 m, as in )), λ monotonically increases at first and then tends toward a stable value of 1.00. However, when the lens is at the sink zone (x = 0.375 and 0.5 m), λ increases first until reaching its maximum value at a depth of approximately 0.45 m. After this, λ decreases gradually and finally tends to be stable. That is to say, the impact of a low-permeability lens on hyporheic exchange is dominated by a hindering effect when the lens is at the source zone, and this hindering effect dampens with increasing depth of the lens. In the case when the lens is at the sink zone, the hindering effect weakens first with increasing depth, then the impact of the lens on hyporheic flow changes to enhancement. The enhancement effect increases first and then attenuates at a certain depth. Below the depth of 0.6 m, the low-permeability lens has little influence on hyporheic exchange at all horizontal locations, which means hyporheic exchange tends toward a stable value qs when the lens is below a certain depth ds. The ds (which we term the “stable depth”) could be defined as the deepest depth where the low-permeability lens can affect the process of hyporheic exchange and qs (“stable hyporheic exchange”) defines the stable value of hyporheic exchange when the low-permeability lens is located below the depth of ds.
Impact of the size of the low-permeability lens
The aforementioned influence patterns of spatial location on λ were based on a low-permeability lens with constant length and thickness. To verify its universality, the lengths and thickness of the low-permeability lens were changed according to . It can be seen from Figure 5 that λ shows a similar changing pattern for various lengths and thicknesses of the lens. Specifically, λ increases when the lens is moved from the source zone to the sink zone and from a shallow to a deep depth. The horizontal variations of λ are depth dependent and the vertical variation patterns of λ are horizontal location dependent when the lens is of various sizes. That is to say, the variation pattern of λ and hyporheic exchange to the spatial locations of the lens is unchanged with the sizes of the lens, which can be summarized as follows:
The impact of the lens on hyporheic exchange is associated with its horizontal location. In the source zone, the effect of the lens is dominated by a hindering effect, which is attenuated gradually with increasing depth. In contrast, the impact of the lens at the sink zone is mainly an enhancing effect, which is strengthened at first and then weakened with increasing depth.
The impact of the lens is controlled by its depth. At shallow depths the low-permeability lens hinders the process of hyporheic exchange, while with increasing depth, the lens can enhance hyporheic flux. If the low-permeability lens reaches a stable depth ds (approximately 0.6 m here), the impact of the lens on hyporheic exchange is negligible.
Even though the size of the low-permeability lens has little influence on the altering pattern of λ and hyporheic exchange, it is obvious from that the value of λ is influenced by the size of the lens, which means the intensity of the hyporheic exchange is controlled by the size. The curves of λ versus depth of lenses with different length and thickness are shown in Figure 6(a) and (b), to illustrate the impact of size on the intensity of hyporheic exchange.
Figure 5. Relationship between spatial location and λ under low-permeability lens of various lengths (l) and thicknesses (t).
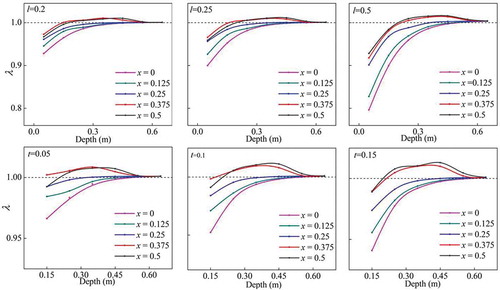
It can be seen from ) that at the source zone (x = 0 and 0.25 m), an increase in length leads to the reduction of λ at shallow depth, which means a longer low-permeability lens has a hindering effect on hyporheic exchange. At the stable depth ds (approximately 0.6 m here), λ tends to have a greater stable value when the length of the low-permeability lens increases ()). For the curves at the sink zone (x = 0.5 m), similarly to the case at the source zone, the increase in length strengthens the hindering effect of the lens on hyporheic flow at shallow depth. However, at deep depths the effect of the lens is dominated by enhancement of exchange; λ rises with increasing length, which means a longer low-permeability lens has greater enhancement effects on hyporheic exchange. Furthermore, the stable value of λ also increases with increasing length of the lens at the sink zone. Thus it can be concluded from the above analysis that the increase of length would strengthen the impact of a low-permeability lens on hyporheic processes. In addition, stable hyporheic exchange qs also increases when induced by a longer low-permeability lens. Similarly, it can be seen from ) that a thicker low-permeability lens has a greater hindering effect on hyporheic exchange at shallow depth and greater enhancement effects at greater depths. That is to say, increasing the thickness also strengthens the impact of a lens on the hyporheic process. However, it should be noted that the change in thickness does not affect the stable value of the stable hyporheic exchange qs.
Figure 6. Relationship between intensity of the hyporheic exchange and (a) length of the low-permeability lens and (b) thickness of the low-permeability lens. Horizontal locations of x = 0, 0.125 and 0.25 m were chosen to represent locations from source point to sink point.
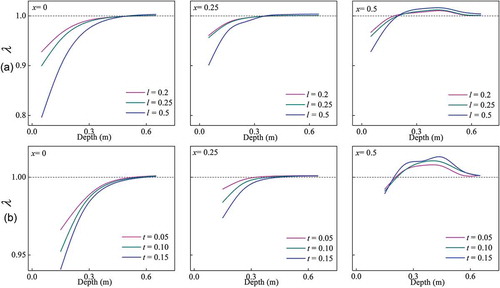
To compare the impact of length and thickness on hyporheic exchange, a sensitivity analysis was conducted according to Lu et al. (Citation2011). The sensitivity coefficient is equal to the ratio of λ increments to the variations in length and thickness, which are expressed as Ul and Ut, respectively. shows the sensitivity coefficients of λ to length and thickness of the low-permeability lens at various spatial locations. If Ul or Ut is less than 0, it means that λ decreases with increasing length or thickness. Otherwise, it means that λ has a positive correlation with length or thickness. It can be seen from that at a shallow depth (z = 0.15 and 0.35 m), the absolute value of Ul is much greater than Ut, which means that hyporheic exchange is more sensitive to the length of the low-permeability lens at shallow depth.
Table 2. Sensitivity coefficient of λ to the length and thickness of the low-permeability lens at various spatial locations.
Impact of regional GW–SW interactions
Previous studies have shown that regional GW–SW interactions could attenuate the process of hyporheic exchange (Gariglio et al. Citation2013, Fox et al. Citation2014); thus, it can be inferred that the effect of low-permeability lenses on the hyporheic process may be influenced by the existence of regional groundwater. Our above analyses were based on a gaining river condition with qG = −0.001 m d−1. To reveal the impact of regional GW–SW interactions on hyporheic exchange controlled by a low-permeability lens, three types of streamflow conditions were considered in the dune-induced hyporheic flow model: neutral ( and ), losing ( and ) and gaining ( and ). In addition, qG was given various intensities to study its impact on hyporheic exchange (qG = 0, ±0.001 and ±0.003 m d−1). We have concluded that the effect of a low-permeability lens on hyporheic exchange is horizontal location dependent and depth dependent. This is also correct under a losing stream condition ( and ). To be specific, in the horizontal direction, the hindering effects or enhancing effects of a low-permeability lens are associated with whether the low-permeability lens is at the source zone or sink zone. In the vertical direction, the low-permeability lens hinders the process of hyporheic exchange when positioned at a shallow depth, while with increasing depth, it mainly strengthens hyporheic exchange. However, in contrast to a gaining condition, it is at the source zone that the low-permeability lens enhances hyporheic exchange (x = 0.05 and 0.125 m; and ) and at the sink zone that the lens hinders hyporheic exchange (x = 0.25, 0.375 and 0.5 m; and ). That is to say, at the source zone and sink zone, whether the impact of low-permeability lens has a hindering or enhancing effect is dependent on the condition of regional GW–SW interactions: a losing stream leads to hindering at the sink zone and enhancing at the source zone, while a gaining stream enhances at the sink zone and hinders at the source zone.
Figure 7. Variation in hyporheic exchange qhyp versus spatial positions of low-permeability lens under: (a,i) and (b,i) neutral stream; (a,ii) and (a,iii) losing stream; and (b,ii) and (b,iii) gaining stream. The dotted lines in (a) and (b) refer to qhyp = q0, i.e. hyporheic exchange in a stream bed without the existence of a low-permeability lens. If qhyp is above the dotted line, the low-permeability lens enhances the hyporheic process; otherwise, the low-permeability lens hinders hyporheic exchange. The intensity of regional groundwater increases from 0 m d−1 in (a,i) and (b,i) to 0.003 m d−1 in (a,iii) and (b,iii). Part (c) shows the variation trend of λ under qG = 0, −0.003 and −0.005 m d−1.
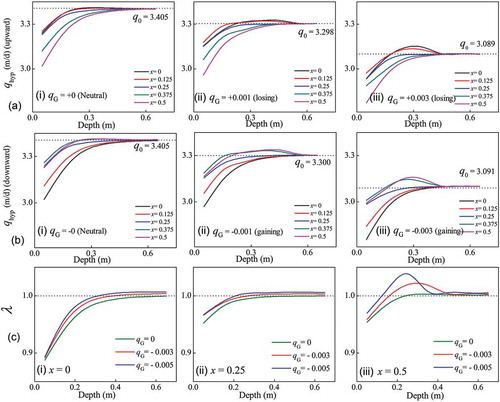
When there is no regional groundwater, hyporheic exchange equals the downward flux that flows into the domain and upward flux that flows out of the domain through the stream-bed interface (), which means qhyp could be estimated by either the sum of qneg or the sum of qpos when qG = 0 m d−1 (Equations (4) and (5), respectively).The variation of the trend of qhyp calculated by Equations (4) and (5) is shown in and , respectively. Overall, qhyp rises with increasing depth of the low-permeability lens and then approaches q0 at the depth of approximately 0.65 m. It can be seen from and that the influence of the lens on hyporheic exchange is dominated by a hindering effect and the enhancing effect is insignificant when qG = 0 m d−1. Furthermore, the hindering effect strengthens when the low-permeability lens is moved from the source zone to the sink zone if hyporheic exchange is estimated by the upward flux (qG = +0 m d−1; ). In contrast, when hyporheic exchange is estimated by downward flux, the hindering effects are mainly focused on the source zone and the low-permeability lens has little effect on the hyporheic process when positioned at the sink zone (qG = −0 m d−1; ). That is, at the source zone, the lens hinders downward hyporheic exchange significantly while having little influence on upward hyporheic exchange in the model. As for the sink zone, upward hyporheic flow is hindered significantly by the low-permeability lens, while downward hyporheic flow is nearly uninfluenced. In theory, upward hyporheic exchange equals downward hyporheic exchange when qG = 0 m d−1. However, it should be noted that, in this study, hyporheic exchange only reflects the vertical seepage velocities that flow into or out of the domain because the x-directional seepage velocities were ignored when calculating qhyp (Equations (4) and (5)). Thus, the variation in qhyp in is not in contradiction to that in ; the former reflects upward hyporheic flow, while the latter shows downward hyporheic flow. In conclusion, a low-permeability lens hinders the hyporheic process when there is no regional groundwater.
With increasing intensity of regional GW–SW interactions, the value of qhyp decreases gradually at all spatial locations, which means the higher intensity of qG leads to more hindering effects on hyporheic exchange. Apart from the intensity of qhyp, the stable hyporheic flow (qs) also decreases with the strengthening of regional groundwater, which drops from 3.405 m d−1 (qG = 0 m d−1) to 3.100 m d−1 (qG = 0.003 m d−1).
Even though the zone where the low-permeability lens could affect the process of hyporheic exchange shows a reduction when regional groundwater is intensified, it does not mean the impact of a low-permeability lens on the hyporheic process is also attenuated under qG with higher intensity ()). To compare the intensity of effects of a low-permeability lens under various qG, the variation in trends of λ is shown in ). At shallow depths, the higher intensity of qG leads to a higher λ, which indicates less hindering effect from the lens (). However, at relatively deep depths, λ shows a sharper increase to a higher peak point followed by a quicker decrease under the situation of regional groundwater with higher intensities (), which means the existence of higher qG strengthens the enhancement effect at some depths.
Flow field of the hyporheic zone
The flow fields of hyporheic exchange below the stream-bed surface with varied dune location, size and regional groundwater are shown in Figure 8(a)–(c), respectively. In ), the depths of 0.05, 0.25 and 0.55 m, and the horizontal locations of x = 0, 0.125 and 0.25 m are selected as representatives of all vertical locations from shallow to deep and horizontal locations from source point to sink point. It can be seen in that downward water flow is hindered significantly by a lens that is at the source point. With increasing depth, the hindering effect of downward flow by the lens becomes negligible. When the lens is moved slightly to the sink point (), the hindering effect on the hyporheic exchange weakens because the downward flow detours around the bottom of the low-permeability lens. Even though the downward flow tends to be less influenced by a lens positioned at the sink zone, the processes of hyporheic exchange flowing out of the stream-bed interface, i.e. the upward flow at the sink zone, are obviously obstructed, which also leads to attenuation of hyporheic exchange. It can also be seen in that the hyporheic flow path alters significantly with the existence of a shallow-located lens, while it remains nearly unaffected when the lens is relatively deeper. In contrast, the regional GW–SW flow path is more easily influenced by a deeply located lens.
Figure 8. Flow fields of hyporheic exchange controlled by (a) a low-permeability lens with various spatial locations, (b) a lens with various sizes and (c) various intensities of regional groundwater. The ellipse represents a lens; numbers represent the horizontal location and depths of the low-permeability lenses. The hyporheic flow path and regional GW–SW flow path are shown as a dotted line and a dashed line, respectively.
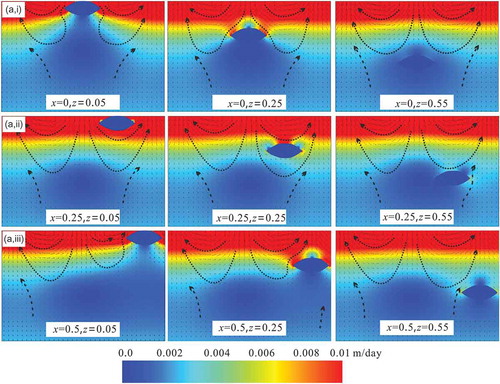
The variation in hyporheic flow and groundwater flow paths under a low-permeability lens with various lengths and thicknesses is shown in ). Two locations, (x = 0, z = 0.15) and (x = 0.375, z = 0.15), are chosen to study the effect of a low-permeability lens at shallow depth at a source zone and a sink zone, respectively. The location (x = 0.25, z = 0.35) is selected to be a representative of relatively deeper locations. Obviously, a longer lens means more obstruction to downward water flowing into the stream bed at the source zone (column x = 0 in ) and more obstruction to upward water flowing out of the stream bed at the sink zone (x = 0.375 in ). For the case when the lens is deeper (x = 0.25 in ), both the hyporheic flow paths and groundwater flow paths are influenced significantly. Compared to the impact of length, the flowing fields of hyporheic exchange do not show obvious variations with increasing thickness (), which means hyporheic flow fields are insensitive to thickness compared to the length of a low-permeability lens.
The flow fields of hyporheic exchange under the impact of qG of various intensities are shown in ). Obviously, the flow of hyporheic exchange in a stream bed is suppressed significantly by regional groundwater, especially a qG of higher intensity (). In addition, the depth of the hyporheic zone (the maximum depth of the stream bed with the existence of hyporheic exchange, which was termed dhyp) also decreases markedly with the strengthening of regional groundwater. It has been revealed from that not only qs but also ds decrease with increasing regional groundwater. Thus, both the hyporheic zone and the zone where the lens can affect hyporheic processes tend to a shallower zone of the stream beds when regional groundwater exists. It can be inferred that ds is positively related to dhyp. Because of the high intensity of the regional groundwater, the flow area of the hyporheic exchange is constrained to a thinner zone of the stream bed, which leads to the lens influence on hyporheic exchange occurring at shallower stream-bed depth.
Variation in the critical line
Apart from the intensity of the hyporheic exchange, the depth where one can distinguish the hindering effect and enhancement effect of a low-permeability lens (or the depth where λ = 1.00) is also influenced by the length and thickness of the lens. This depth is termed “critical depth” herein, and the line drawn by critical depth at all horizontal locations is referred to as the “critical line”. Apparently, the effect of a lens that is above the critical line is hindering, while there is an enhancement effect when the lens is below the critical line. The critical lines under the control of a lens with various lengths and thicknesses are shown in Figure 9(a) and (b), respectively. In general, the critical depth at the source zone is deeper than that at the sink zone, with the shallowest depth at approximately x = 0.4 m. Increasing the length of the lens leads to a decrease in critical depth at the source zone and an increase in the critical depth at the sink zone ()), which means a longer lens weakens the variation in critical depth along the horizontal direction. That is to say, a longer lens leads to a gently varying critical line. However, the critical depth at all horizontal locations increases with increasing thickness ()), resulting in a downward translational critical line when the thickness increases, which indicates that a thicker lens leads to a thicker hindering zone.
Figure 9. Critical lines controlled by (a) low-permeability lenses of various lengths, (b) low-permeability lenses of various thicknesses, (c) losing condition and (d) gaining condition. The x-coordinate refers to the horizontal location and z the depth of the low-permeability lens.
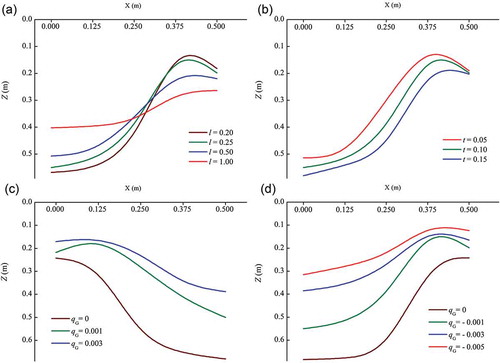
The critical lines under various regional GW–SW interactions are shown in ) and (d). The critical lines under losing conditions ()) show the opposite variation in trends compared to that under gaining conditions ()). Similar to the stable depth ds, the critical depth, which distinguishes the hindering and enhancement effects of a lens, also decreases with strengthening of the regional groundwater. That is to say, because of regional groundwater, not only are the processes of hyporheic exchange suppressed to a smaller region (shallower zone of the stream bed), but also the zone where hyporheic exchange can be influenced by a lens is constrained to a thinner zone of the stream bed. Thus, when we describe the hindering or enhancing effects of a lens at “shallow” or “deep” depth, we actually refer to a relatively shallow and a relatively deep depth, depending on various regional groundwater conditions.
Discussion
Mechanism of enhancing effects
It is easy to understand that the existence of a low-permeability lens can hinder the hyporheic process. To analyse the reason why a lens can enhance hyporheic exchange, the hyporheic flow fields and regional groundwater flow fields under losing conditions and gaining conditions are shown in and , respectively. It has been shown that, under losing conditions, the lens enhances hyporheic processes when positioned at the source zone, while under gaining conditions at the sink zone the lens enhances hyporheic exchange. From the regional groundwater flow fields shown in ) and , it can be seen that regional groundwater flows mainly below the source point when it is a losing stream, while in gaining conditions, the regional groundwater flowing zone is mainly at the sink zone. That is to say, the zones where a lens enhances hyporheic exchange are consistent with the regional groundwater flow zones () and ). The existence of regional groundwater can hinder the processes of hyporheic exchange; thus, it can be inferred that the lens blocks the flow of regional groundwater when positioned at the source zone under losing conditions and at the sink zone under gaining conditions, leading to a reduction in the hindering effect of regional groundwater on hyporheic exchange, which results in “enhancement effects” of the lens on the hyporheic process. At a shallower stream bed, the water flow is dominated by hyporheic exchange, thus the enhancement effects only occur at relatively greater depth.
Figure 10. Conceptual illustrations of hyporheic flow fields and regional groundwater flow fields under (a) losing conditions and (b) gaining conditions. Conceptual illustrations of hindering zone, enhancement zone and stable zone under (c) losing condition, (d) gaining condition and (e) regional groundwater of higher intensity. Dotted lines refer to the critical line, where the upper (red) and lower (green) lines represent critical lines under a longer low-permeability lens and a thicker low-permeability lens, respectively. The solid (blue) line refers to the interface of the hyporheic zone.
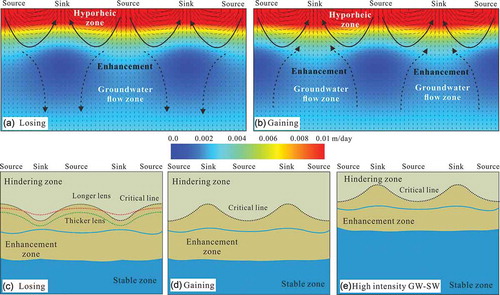
The effect of a low-permeability lens and regional GW–SW interactions on dune-induced hyporheic exchange can be seen in )–. The lens hinders hyporheic exchange at shallower depths, shown as a “hindering zone”. With increasing depth, hyporheic flow is enhanced by the lens, which results in an “enhancement zone” in the stream bed. Below a relatively deeper depth, the effect of the lens on hyporheic exchange can be neglected, and this is shown as a “stable zone” in . The hindering zone and enhancement zone are distinguished by the critical line, which has an opposite variation in trends under losing and gaining conditions () and ). With increasing intensity of regional groundwater, the hyporheic, hindering and enhancement zones are suppressed to a shallower depth of the stream bed ()).
Implications and limitations
Many studies have proved that hyporheic exchange decreased with increasing regional groundwater (Cardenas and Wilson Citation2007b, Fox et al. Citation2014, Marzadri et al. Citation2016) by both numerical simulation and physical experiments. The variation in q0 in provides the same conclusion, which reflects the reliability of the results in this study. According to Cardenas and Wilson (Citation2006), groundwater discharge reduces the spatial extent of hyporheic exchange zones and may completely prevent the development of dune-induced flow at greater groundwater discharge, which can also be seen in in this study. In addition, this study has indicated that not only the process of hyporheic exchange, but also the impact of a lens on hyporheic processes is suppressed to a shallower depth of the stream bed because of regional GW–SW interactions, indicating regional groundwater conditions are the dominant mechanisms of water exchange in a stream bed, which is consistent with the conclusion of Fox et al. (Citation2014).
This study proved that heterogeneity such as a low-permeability lens in stream bed can affect the intensity and flow paths of hyporheic exchange significantly. The impact of a low-permeability lens consists of both hindering effects and enhancement effects, depending on the relative spatial location of the lens to dunes, such as at the source zone or at the sink zone. However, it is difficult to judge whether a lens is at the source zone or sink zone of dunes in natural stream beds. Furthermore, both the size of a lens and regional GW–SW interaction conditions can affect the impact of a lens on hyporheic processes. Even though the effect factors of the lens on hyporheic processes are complicated, this does not mean we can not make some inferences when facing a lens-controlled dune-induced hyporheic exchange at field sites. Generally speaking, a lens at a shallower depth of the stream bed is most likely to hinder the process of hyporheic exchange. In contrast, if the lens is deeper in the stream bed, it is more probable that it will enhance the flow of hyporheic flux or have little influence on the process of hyporheic exchange. For lenses of varying sizes, longer and thicker lenses seems to have greater effects.
In addition to the impact on intensity and the flow path of hyporheic exchange, a low-permeability lens in a stream bed may also affect the ecological and biogeochemical processes in the stream bed. For example, the spatial distribution of the vertical flow at the GW–SW interface is a sensitive output that pertains to ecological processes (Käser et al. Citation2013). Thus, excluding the existence of a lens in stream bed, which can hinder or enhance vertical hyporheic exchange significantly, can lead to an unreliable estimation of hydrological patchiness, and consequently of the actual microhabitat patchiness. The hyporheic flux, hyporheic flow path and residence time distribution play an important role in biogeochemical cycles in stream-bed sediments (Boulton and Valett Citation1998, Hu et al. Citation2014). The existence of a lens can affect the physico-chemical transformations of reactive solutes and thus influence nutrient supply and benthic habitat quality in a riverine ecosystem. For example, in a gaining stream, a lens in the source zone hinders the inflow of surface water, which may result in weakening of the role of sediment as a dissolved organic carbon and dissolved oxygen sink and the reduction of nitrification reaction. A shallow-located lens in a stream bed leads to a shorter flow path length of hyporheic exchange; so neglecting the impact of lenses in the stream bed might result in an overestimation of removal. It can be inferred that a low-permeability lens in a stream bed also alters the distribution of residence times because the intensity of the hyporheic exchange and hyporheic flow path have changed, which would definitely affect the nitrification and denitrification reaction in the hyporheic zone. Hence, the impact of lenses on the residence time distribution of the hyporheic exchange induced by dunes needs to be studied in the future.
It should be noted that, in this study, because of the limitations of the research methods, only vertical seepage velocities are considered when calculating hyporheic exchange, which can result in errors to some extent. In addition, this study only considered the impact of a single low-permeability lens. Hence, improvements in this type of study should be made in the future.
Conclusion
This study focused on hyporheic processes of bedform size and uncovered the impact of low-permeability lenses on dune-induced hyporheic exchange, thus promoting better understanding of the impact of heterogeneity on the hyporheic process at small spatial scales to some extent. The following two questions were addressed in this study: (1) How do hyporheic flow paths and exchange intensity respond to a low-permeability lens with varying spatial locations and sizes? And (2) How do regional GW–SW interactions affect the aforementioned response mechanism?
The impact of a lens on hyporheic exchange is associated with its spatial location. In the horizontal direction, whether the impact of a lens is dominated by hindering or enhancing effects at the source zone or sink zone is dependent on the condition of regional GW–SW interactions. A losing stream results in hindering at the sink zone and enhancing at the source zone, while a gaining stream results in enhancing at the sink zone and hindering at the source zone. In the vertical direction, a lens hinders the processes of hyporheic exchange at shallower depths, while with increasing depth, a lens can enhance the flow of hyporheic flux. Similar to the intensity of hyporheic exchange, hyporheic flow paths are significantly altered with the existence of a shallow-located lens, while they remain nearly unaffected when the lens is relatively deep. In contrast, the regional GW–SW flow path is more easily influenced by a deeply located lens.
The size of a lens controls the intensity of the effects on hyporheic processes. Specifically, an increase in both length and thickness could strengthen the impacts of the lens on hyporheic exchange, while hyporheic exchange is more sensitive to the length of the lens compared to thickness in a shallow zone. Increasing length of the lens leads to a gently varying critical line. However, an increase in thickness leads to a downward translational critical line.
Regional GW–SW interactions of higher intensity suppress the flow of hyporheic exchange in a stream bed and dominate the effect of a low-permeability lens. A low-permeability lens blocks the flow of regional groundwater, leading to a reduction in the hindering effects of regional groundwater on hyporheic exchange, which results in enhancement effects of a lens on the hyporheic process.
Acknowledgements
The authors would like to express their appreciation for the suggestions and edits provided by Professor Andrew Binley, Lancaster University, UK. The authors also would like to express their appreciation to the suggestions and comments provided by Dr Kuangjia Li, Tianjin University. The data for the numerical model can be obtained from the corresponding author (contact: [email protected]).
Disclosure statement
No potential conflict of interest was reported by the authors.
Additional information
Funding
References
- Bhaskar, A.S., Harvey, J.W., and Henry, E.J., 2012. Resolving hyporheic and groundwater components of streambed water flux using heat as a tracer. Water Resources Research, 48 (8), 1076. doi:10.1029/2011WR011784
- Boano, F., et al., 2015. Hyporheic flow and transport processes: mechanisms, models, and biogeochemical implications. Reviews of Geophysics, 52 (4), 603–679.
- Boano, F., Revelli, R., and Ridolfi, L., 2008. Reduction of the hyporheic zone volume due to the stream-aquifer interaction. Geophysical Research Letters, 35 (9), 250–258. doi:10.1029/2008GL033554
- Boano, F., Revelli, R., and Ridolfi, L., 2009. Quantifying the impact of groundwater discharge on the surface-subsurface exchange. Hydrological Processes, 23 (15), 2108–2116. doi:10.1002/hyp.v23:15
- Bosch, D.D., Truman, C.C., and Davis, F.M., 2001. Vadose zone clay lens impacts on groundwater loading rates. In: Preferential flow: water movement and chemical transport in the environment, 3–5 January, Honolulu, Hawaii, 69–72.
- Bottacin-Busolin, A. and Marion, A., 2010. Combined role of advective pumping and mechanical dispersion on time scales of bed form-induced hyporheic exchange. Water Resources Research, 46 (8), 56–72. doi:10.1029/2009WR008892
- Boulton, A., et al., 2010. Ecology and management of the hyporheic zone: stream-groundwater interactions of running waters and their floodplains. Journal of the North American Benthological Society, 29 (1), 26–40. doi:10.1899/08-017.1
- Boulton, A.J. and Valett, H.M., 1998. The functional significance of the hyporheic zone in streams and rivers. Annual Review of Ecology & Systematics, 29 (1), 59–81. doi:10.1146/annurev.ecolsys.29.1.59
- Bridge, J.S., 2003. Rivers and floodplains: forms, processes, and sedimentary record. Australian Geographical Studies, 48 (3), 386–387.
- Cardenas, M.B. and Wilson, J.L., 2006. The influence of ambient groundwater discharge on exchange zones induced by current–bedform interactions. Journal of Hydrology, 331 (1–2), 103–109. doi:10.1016/j.jhydrol.2006.05.012
- Cardenas, M.B. and Wilson, J.L., 2007a. Exchange across a sediment–water interface with ambient groundwater discharge. Journal of Hydrology, 346 (3–4), 69–80. doi:10.1016/j.jhydrol.2007.08.019
- Cardenas, M.B. and Wilson, J.L., 2007b. Dunes, turbulent eddies, and interfacial exchange with permeable sediments. Water Resources Research, 43 (8), 199–212. doi:10.1029/2006WR005787
- Cardenas, M.B., Wilson, J.L., and Zlotnik, A., 2004. Impact of heterogeneity, bed forms, and stream curvature on subchannel hyporheic exchange. Water Resources Research, 40 (8), 474–480. doi:10.1029/2004WR003008
- Elliott, A.H. and Brooks, N.H., 1997a. Transfer of nonsorbing solutes to a streambed with bed forms: laboratory experiments. Water Resources Research, 33, 137–151. doi:10.1029/96WR02783
- Elliott, A.H. and Brooks, N.H., 1997b. Transfer of nonsorbing solutes to a streambed with bed forms: theory. Water Resources Research, 33 (1), 123–136. doi:10.1029/96WR02784
- Faisal, A.A.H., Kassim, W.M.S., and Hussein, T.K., 2011. Influence of clay lens on migration of light nonaqueous phase liquid in unsaturated zone. Journal of Environmental Engineering-ASCE, 137 (1), 9–14. doi:10.1061/(ASCE)EE.1943-7870.0000297
- Fox, A., et al., 2016. The effect of losing and gaining flow conditions on hyporheic exchange in heterogeneous streambeds. Water Resources Research., 52 (9), 7460–7477. doi:10.1002/2016WR018677
- Fox, A., Boano, F., and Arnon, S., 2014. Impact of losing and gaining streamflow conditions on hyporheic exchange fluxes induced by dune-shaped bed forms. Water Resources Research, 50 (3), 1895–1907. doi:10.1002/2013WR014668
- Freitas, J.G., et al., 2015. Heterogeneous hyporheic zone dechlorination of a TCE groundwater plume discharging to an urban river reach. Science of the Total Environment, 505 (505), 236–252. doi:10.1016/j.scitotenv.2014.09.083
- Gariglio, F.P., Tonina, D., and Luce, C.H., 2013. Spatiotemporal variability of hyporheic exchange through a pool-riffle-pool sequence. Water Resources Research, 49 (11), 7185–7204. doi:10.1002/wrcr.20419
- Gerber, R.E., Boyce, J.I., and Howard, K.W., 2001. Evaluation of heterogeneity and field-scale groundwater flow regime in a leaky till aquitard. Hydrogeology Journal, 9 (1), 60–78. doi:10.1007/s100400000115
- Gomez, J.D., Wilson, J.L., and Bayani, C.M., 2012. Residence time distributions in sinuosity-driven hyporheic zones and their biogeochemical effects. Water Resources Research, 48 (9), 550–556. doi:10.1029/2012WR012180
- Gomez-Velez, J.D., Krause, S., and Wilson, J.L., 2014. Effect of low-permeability layers on spatial patterns of hyporheic exchange and groundwater upwelling. Water Resources Research, 50 (6), 5196–5215. doi:10.1002/2013WR015054
- Guswa, A.J. and Freyberg, D.L., 2000. Slow advection and diffusion through low permeability inclusions. Journal of Contaminant Hydrology, 46 (3–4), 205–232. doi:10.1016/S0169-7722(00)00136-4
- Healy, R. and Ronan, A., 1996. Documentation of computer program VS2DH for simulation of energy transport in variably saturated porous media, Modification of the US Geological Survey’s computer program VS2DT. Reston, VA: US Geological Survey.
- Healy, R.W., 1990. Simulation of solute transport in variably saturated porous media with supplemental information on modifications to the US Geological Survey’s computer program VS2D. Reston, VA: Department of the Interior, US Geological Survey.
- Hester, E.T. and Gooseff, M.N., 2010. Moving beyond the banks: hyporheic restoration is fundamental to restoring ecological services and functions of streams. Environmental Science & Technology, 44 (5), 1521–1525. doi:10.1021/es902988n
- Hester, E.T., Young, K.I., and Widdowson, M.A., 2013. Mixing of surface and groundwater induced by river bed dunes: implications for hyporheic zone definitions and pollutant reactions. Water Resources Research, 49 (9), 5221–5237. doi:10.1002/wrcr.20399
- Hsieh, A., Wingle, W.L., and Healy, R.W., 2000. VS2DI-A graphical software package for simulating fluid flow and solute or energy transport in variably saturated porous media. Reston, VA: US Geological Survey, Water-Resources Investigations Report.
- Hu, H., et al., 2014. Impact of microforms on nitrate transport at the groundwater–surface water interface in gaining streams. Advances in Water Resources, 73 (4), 185–197. doi:10.1016/j.advwatres.2014.07.013
- Jankowski, J. and Beck, P., 2000. Aquifer heterogeneity: hydrogeological and hydrochemical properties of the Botany Sands aquifer and their impact on contaminant transport. Australian Journal of Earth Sciences, 47 (1), 45–64. doi:10.1046/j.1440-0952.2000.00768.x
- Jin, G., et al., 2010. Transport of nonsorbing solutes in a streambed with periodic bedforms. Advances in Water Resources, 33 (11), 1402–1416. doi:10.1016/j.advwatres.2010.09.003
- Jin, G., et al., 2011. Hyporheic flow under periodic bed forms influenced by low-density gradients. Geophysical Research Letters, 38 (22), L22401.1-L22401.6. doi:10.1029/2011GL049694
- Jones, J.B. and Mulholland, J., 2000. Streams and ground waters. Journal of the North American Benthological Society, 19 (4), 760–761. doi:10.2307/1468134
- Karwan, D.L. and Saiers, J.E., 2012. Hyporheic exchange and streambed filtration of suspended particles. Water Resources Research, 48 (1), 273–279. doi:10.1029/2011WR011173
- Kasahara, T. and Wondzell, S.M., 2003. Geomorphic controls on hyporheic exchange flow in mountain streams. Water Resources Research, 39 (1), SBH 3–14. doi:10.1029/2002WR001386
- Käser, D.H., Binley, A., and Heathwaite, A.L., 2013. On the importance of considering channel microforms in groundwater models of hyporheic exchange. River Research & Applications, 29 (4), 528–535. doi:10.1002/rra.v29.4
- Krause, S., et al., 2013. Streambed nitrogen cycling beyond the hyporheic zone: flow controls on horizontal patterns and depth distribution of nitrate and dissolved oxygen in the upwelling groundwater of a lowland river. Journal of Geophysical Research: Biogeosciences, 118 (1), 54–67. doi:10.1029/2012JG002122
- Landon, M.K., Rus, D.L., and Harvey, F.E., 2001. Comparison of instream methods for measuring hydraulic conductivity in sandy streambeds. Ground Water, 39 (6), 870–885. doi:10.1111/j.1745-6584.2001.tb02475.x
- Lu, C., et al., 2011. Parameter estimation for a karst aquifer with unknown thickness using the genetic algorithm method. Environmental Earth Sciences, 63 (4), 797–807. doi:10.1007/s12665-010-0751-8
- Marzadri, A., et al., 2010. Semianalytical analysis of hyporheic flow induced by alternate bars. Water Resources Research, 46 (7), 58–72. doi:10.1029/2009WR008285
- Marzadri, A., et al., 2016. Mixing interfaces, fluxes, residence times and redox conditions of the hyporheic zones induced by dune-like bedforms and ambient groundwater flow. Advances in Water Resources, 88, 139–151. doi:10.1016/j.advwatres.2015.12.014
- Milosevic, N., et al., 2012. Identification of discharge zones and quantification of contaminant mass discharges into a local stream from a landfill in a heterogeneous geologic setting. Journal of Hydrology, 446–447, 13–23. doi:10.1016/j.jhydrol.2012.04.012
- Packman, A.I. and Brooks, N.H., 2001. Hyporheic exchange of solutes and colloids with moving bed forms. Water Resources Research, 37 (10), 2591–2605. doi:10.1029/2001WR000477
- Peterson, E.W. and Sickbert, T.B., 2006. Stream water bypass through a meander neck, laterally extending the hyporheic zone. Hydrogeology Journal, 14 (8), 1443–1451. doi:10.1007/s10040-006-0050-3
- Rathfelder, K.M., et al., 2001. Surfactant enhanced recovery of tetrachloroethylene from a porous medium containing low permeability lenses. 2. Numerical simulation. Journal of Contaminant Hydrology, 48 (3–4), 351–374. doi:10.1016/S0169-7722(00)00186-8
- Revelli, R., et al., 2008. Intra‐meander hyporheic flow in alluvial rivers. Water Resources Research, 44 (12), 181–198. doi:10.1029/2008WR007081
- Reynolds, D.A. and Kueper, B.H., 2001. Multiphase flow and transport in fractured clay/sand sequences. Journal of Contaminant Hydrology, 51 (1–2), 41–62. doi:10.1016/S0169-7722(01)00121-8
- Salehin, M., Packman, A.I., and Paradis, M., 2004. Hyporheic exchange with heterogeneous streambeds: laboratory experiments and modeling. Water Resources Research, 40 (11), 309–316. doi:10.1029/2003WR002567
- Sophocleous, M., 2002. Interactions between groundwater and surface water: the state of the science. Hydrogeology Journal, 10 (2), 348. doi:10.1007/s10040-002-0204-x
- Stonedahl, S.H., Harvey, J.W., and Packman, A.I., 2013. Interactions between hyporheic flow produced by stream meanders, bars, and dunes. Water Resources Research, 49 (9), 5450–5461. doi:10.1002/wrcr.20400
- Storey, R.G., Howard, K.W.F., and Dudley, W.D., 2003. Factors controlling riffle-scale hyporheic exchange flows and their seasonal changes in a gaining stream: a three-dimensional groundwater flow model. Water Resources Research, 39 (2), 180–189. doi:10.1029/2002WR001367
- Thibodeaux, L.J. and Boyle, J.D., 1987. Bedform-generated convective transport in bottom sediment. Nature, 325 (6102), 341–343. doi:10.1038/325341a0
- Tonina, D., et al., 2016. Does streambed heterogeneity matter for hyporheic residence time distribution in sand-bedded streams? Advances in Water Resources, 96, 120–126. doi:10.1016/j.advwatres.2016.07.009
- Tonina, D. and Buffington, J.M., 2007. Hyporheic exchange in gravel bed rivers with pool-riffle morphology: laboratory experiments and three-dimensional modeling. Water Resources Research, 43 (1), 208–214. doi:10.1029/2005WR004328
- Wagner, F.H. and Bretschko, G., 2002. Interstitial flow through preferential flow paths in the hyporheic zone of the Oberer Seebach. Austria: Aquatic Sciences, 64 (3), 307–316.
- Winter, T.C., et al., 1998. Ground water and surface water: a single resource. Reston, VA: US Geological Survey, 1139.
- Wu, G., et al., 2015. Variations of streambed vertical hydraulic conductivity before and after a flood season. Hydrogeology Journal, 23 (7), 1603–1615. doi:10.1007/s10040-015-1275-9
- Wu, G., et al., 2016. The heterogeneity of 3-D vertical hydraulic conductivity in a streambed. Hydrology Research, 47 (1), 15–26. doi:10.2166/nh.2015.224