ABSTRACT
The thermal regimes of lakes are important for understanding the functions and services of lake ecosystems. However, continuous lake temperature monitoring across the Tibetan Plateau is sparse, which inhibits verification of modeling results and also in-depth understanding of the lake thermal and mixing dynamics in this region. Here, we present the results of continuous temperature monitoring of a dimictic lake, Bangong Co, during 2012–2014, and a meromictic lake, Dagze Co, during 2012–2015, to gain insight into the differences in their thermal dynamics and to understand the factors influencing these differences. No evident changes in the thermal and mixing regimes were observed at the two lakes during the observational period. However, the thermal structure changed in different hydrological years. The air temperature changes and associated glacier melt possibly contribute to changes in the water temperature for Bangong Co, while heat transfer related to wind speed is vital for Dagze Co. Developing in-depth knowledge necessitates long-term monitoring data to confirm the influence of various individual climatic parameters.
Editor A. Castellarin ; Associate editor K. Kochanek
1 Introduction
Lakes are widely distributed all over the world and are one of the most commonly used water resources (Messager et al. Citation2016, Immerzeel et al. Citation2020). Lake sediments are also valuable archives of information on past changes in climate, environments and lake ecosystems, and provide important ecological services (Meyers and Lallier-Vergés Citation1999, Leavitt and Hodgson Citation2001, Smol et al. Citation2005, Fritz Citation2008). However, as the sentinels of climate change on Earth (Adrian et al. Citation2009), lakes are experiencing significant changes (Woolway et al. Citation2020), including increased lake water temperature (O’Reilly et al. Citation2015), decreased winter ice cover (Tan et al. Citation2018, Sharma et al. Citation2019), increased lake evaporation (Wang et al. Citation2018) and modified lake mixing regimes (Woolway and Merchant Citation2019). The thermal regime plays a very important role in the lacustrine ecosystem; for example, changes in the thermal regime affect not only the physical-chemical parameters in the water column but also the distribution of microbial substrates that are important for the aquatic biota (Roemmich and Mcgowan Citation1995, Adrian et al. Citation1999, Wetzel Citation2001, Gerten and Adrian Citation2002, Straile Citation2002, Macintyre Citation2013). The lake mixing regime also exerts an influence on the emission of greenhouse gases (GHGs; mainly CO2, CH4 and N2O). Recent studies have shown that the littoral zone of lakes act as sources of GHGs to the atmosphere (Chen et al. Citation2009, Yan et al. Citation2018). Therefore, an in-depth understanding of thermal and mixing regimes in lakes would help to clarify how these water bodies respond to climate variability, and to extend our knowledge of their role in carbon budgets.
The stratification and thermal characteristics of lakes are mainly affected by surface energy balance, wind, salinity, lake morphometry and the light extinction coefficient of water (Schindler Citation1997, Magnuson et al. Citation2000, Livingstone Citation2003, Winder and Schindler Citation2004, Wang et al. Citation2014). It has been suggested that long-term changes in lake stratification and thermal regimes are influenced by climate change (Macintyre et al. Citation2009, Mueller et al. Citation2009, Kraemer et al. Citation2015, Ficker et al. Citation2017, Woolway and Merchant Citation2019, Woolway et al. Citation2020). For example, the results of a long-term temperature monitoring programme in a small kettle lake in Alaska indicated that the mixing regimes were significantly correlated with mean air temperatures and the frequency and persistence of strong winds (Macintyre et al. Citation2009). Analysis of a temperature profile at Lake Tahoe since 1968 showed that the stratification duration increased gradually during the observation period due to a warming climate over the past several decades, a pattern expected to continuously increase under the current climate warming (Sahoo et al. Citation2016). The thermal regimes within lakes and their influencing factors have been studied for decades around the world (Paerl et al. Citation1975, Gulati et al. Citation2017, Woolway and Merchant Citation2019).
There are ~1400 lakes with areas greater than 1 km2 on the Tibetan Plateau (TP) (Zhang et al. Citation2019), and they constitute the largest group of alpine lakes on the Earth (Wan et al. Citation2016, Zhang et al. Citation2020). It is believed that the lakes in Tibet have unique features in terms of their thermal and mixing dynamics, based on comparisons between in situ observed data from the lakes across Tibet and the temperature profiles of other lakes around the world. However, there has been only limited research focusing on the lake mixing regime across the TP (Wang et al. Citation2014, Citation2019, Citation2020b, Lei et al. Citation2019), and more information on the thermal regimes of alpine lakes around the world is needed. The hydrology and limnology of some of these lakes have been investigated for palaeoclimatological purposes, and the water temperature profiles of TP lakes have been sporadically measured (Li et al. Citation2001, Wang et al. Citation2009, Mischke et al. Citation2010). Few systematic investigations into the thermal regimes on the TP have been carried out, because of logistical and management difficulties.
In 2012, we launched a programme to monitor the temperature variability within the water columns in two alpine lakes, and firstly proved that Dagze Co is a meromictic lake (stably stratified), based on continuous in situ observational data (Wang et al. Citation2014). Investigating the thermal regimes in these lakes will be extremely important for understanding the lakes’ ecological functions. This study aims to achieve the following goals: (1) investigate the thermal regimes and structures of two lakes, Bangong Co on the western TP and Dagze Co on the central TP; and (2) study the potential factors influencing the variation in these lakes’ thermal regimes or structures, based on continuous temperature measurements.
2 Study sites
Bangong Co (33°26ʹ~33°58ʹN, 78°25ʹ~79°56ʹE, 4220 m a.s.l.) is the largest lake of tectonic origin on the western TP ()). The area of the lake and the watershed is 671 km2 and ~25,787 km2, respectively (Khan et al. Citation2014, Wan et al. Citation2016). The maximum depth is 42.6 m. The mean annual precipitation (MAP) is 87 mm, and the mean annual air temperature (MAAT) is 2°C, based on measurements at the Ngari Station for Desert Environment Observation and Research (NASDE, 2010–2012), which is ~15 km south of the lake ()). The eastern part of the lake is mainly fed by meltwater from glaciers via three major inflow rivers: Chiao Ho (alternative name: Duoma Qu, Wu Jiang), Nama Chu (Angmai Qu), and Makha (Maga Zangbu) (Fontes et al. Citation1996). Bangong Co is ice covered from November to April, according to NASDE observation.
Figure 1. (a) Catchment of Bangong Co; (b) catchment of Dagze Co. The inserts show the locations of Bangong Co and Dagze Co on the Tibetan Plateau
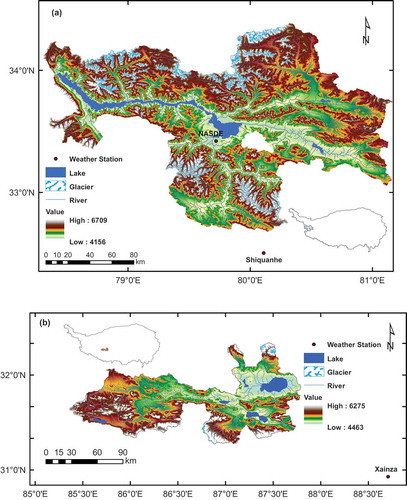
Dagze Co (31°49ʹ~31°59ʹN, 87°25ʹ~87°39ʹE, 4450 m a.s.l.) is a brackish lake on the central TP ()), ~30 km from the town of Nyima. The area of Dagze Co is 311 km2 (Wan et al. Citation2016), and its watershed is 12 849 km2 (Lehner et al. Citation2006). The maximum depth of this lake is 38 m (Liu et al. Citation2013). The lake is mainly fed by precipitation and Bogcarg Zangbo (Zangbo means river in the Tibetan language). The salinity of the surface water was 14.69 g/L (measured on 8 August 2012). The MAP and MAAT, according to Xainza weather station, are 316 mm and 0.55°C, respectively (2012.1–2012.12). The station is located ~150 km southeast of the lake ()), and is the nearest one to the lake.
3 Methods
3.1 Temperature measurements
We used water temperature loggers (HOBO Water Temperature Pro v2 Data Logger – U22-001, Onset Computer Corporation, Bourne, Massachusetts, USA) to monitor temperature variability within the water columns of the two lakes. The U22-001 instrument measures temperatures between approximately −40 and +50°C in water, with ± 0.21°C accuracy. At Bangong Co, the temperature loggers were installed at 33°31ʹ34.84″N, 79°49ʹ42.07″E at a water depth of 36.6 m. Seventeen loggers were installed at intervals of 1 m above 11 m depth, at 2 m intervals between 11 and 23 m depth, and at 3 m intervals below 23 m depth. The loggers were programmed to log lake water temperature data beginning at 00:00 on 29 July 2012, with a logging interval of 1 h. The temperature data were retrieved on 20 August 2013 and 4 June 2014. On 4 June 2014, the temperature loggers were replaced in the lake at the same location. However, they were not found in the lake in July 2015 when we attempted to retrieve them. Therefore, we could evaluate temperature data at Bangong Co only from August 2012 to June 2014.
At Dagze Co, the temperature loggers were installed at 31°51ʹ40.30”N, 87°33ʹ30.62”E at a water depth of 37.3 m. Eighteen temperature loggers were installed with the same distribution as that used for Bangong Co. The loggers were programmed to log lake water temperature data beginning at 00:00 on 19 August 2012, and the data-acquisition frequency was set to one measurement per hour (data was logged every 2 h beginning 29 August 2013). The loggers were retrieved on 29 August, 26 June and 11 July in 2013, 2014 and 2015, respectively. The uppermost loggers were set at a depth of ~5 m at Bangong Co and ~4 m at Dagze Co, to prevent the influence of ice freezing and melting throughout the monitoring period. The temperature profiles of the water columns would be different between deep and shallow areas. Hence, we choose monitoring sites near the depocentre of each lake that could represent the thermal regimes for the deep depths within the lake.
3.2 Analysis of the profile data using Lake Analyzer
We analysed the temperature profile data for Bangong Co and Dagze Co using Lake Analyzer, which is a numerical program used to determine the indices of mixing and stratification that are critical to the biogeochemical cycles of lakes and reservoirs (Read et al. 2011). The Lake Analyzer program requires various subsets of the input dataset, including lake bathymetry, water temperature, wind, water level and salinity. The water temperature series at the two lakes were obtained in this study. Wind speed data were measured at the nearest weather station to each lake, in the town of Shiquanhe (32°29′38.36″N, 80°6′5.79″E, 4287 m a.s.l.), which is ~100 km south of Bangong Co ()), and the town of Xaiza (30°55′47.47″N, 88°42′28.88″E, 4686 m a.s.l.), which is ~150 km southeast of Dagze Co ()). The fluctuations of water level were recorded as changes in pressure; hence, the air pressure was subtracted from the nearby weather station to obtain the pressure changes that related to the water column. (For more details regarding the water-level observations, please refer to Lei et al. Citation2017.) Salinity was measured using a YSI Multiparameter Sonde once a year, when we visited the lakes.
We calculated various stability indices using Lake Analyzer, including Schmidt stability (St), Wedderburn number (W), lake number (LN) and the depths of the mixed layers at the two lakes. Schmidt stability represents the resistance to mechanical mixing caused by the potential energy inherent in the stratification of the water column (Schmidt Citation1928, Hutchinson and Edmondson Citation1957, Idso Citation1973). The Wedderburn number describes the likelihood of upwelling events under stratified conditions (Thompson Citation1980). The lake number, defined by Imberger and Patterson (Citation1989), has been used to describe processes relevant to the internal mixing of lakes induced by wind forcing. The depth of the mixed layer was defined as the vertical portion of the water column that is directly influenced by the surface drivers of wind and convective cooling (Imberger Citation1985). Lake Analyzer calculated the depth of the mixed layer based on a density gradient threshold (Read et al. 2011). We focus on Schmidt stability and Wedderburn number in the following discussion.
3.3 Rescaled range (R/S) analysis of temperature profiles
We performed rescaled range (R/S) analysis on the temperature observations at various depths at Bangong Co and Dagze Co. R/S was introduced in 1951 by hydrologist Harold Edwin Hurst, who aimed to measure the variability and assess the persistence of time-series data (Hurst Citation1951). The rescaled range is calculated by dividing the range of the values (R) by the standard deviation (S) of the observation over the same portion of the time series (North and Halliwell Citation1994). The Hurst exponent (H) is defined as the slope between ln(R/S) and ln(n), which quantifies the relative tendency of a time series either to regress strongly to the mean or to cluster in a particular direction (Mishra et al. Citation2009). H values between 0.5 and 1 indicate a time series with long-term positive autocorrelation, and H values between 0 and 0.5 indicate a time series with long-term switching between high and low values in adjacent pairs. Generally, higher H values indicate stronger persistence of a time series.
4 Results
4.1 Temperature profiles at Bangong Co
Continuous temperature measurements at Bangong Co (from 29 July 2012 to 4 June 2014) show that it is a dimictic lake ()). During the summer stratification, a thermocline developed from 14 to 27 m depth with a temperature of ~16°C near the surface and ~8°C at the bottom in late July 2012. During the winter, the lake was covered by ice and exhibited a very small vertical change in water temperature, albeit a negligible weak inverse stratification was detected. The lowest average lake water temperature occurred during late December and early January; subsequently, the lake water progressively warmed to ~3.5°C by the end of March. The lake water overturned completely twice a year, in spring (late April to early June) and autumn (late October to early December). It is worth noting that a short-term stratification was observed during April in 2013 and 2014. Temperature measurements at 11 m were slightly lower than those for water below and above this depth throughout the 2 years. The variations of the temperature at the uppermost logger (~4 m) were generally consistent with the air temperature at the weather station in the town of Shiquanhe, which is located ~100 km south of the lake.
Figure 2. Comparison of Lake Analyzer calculations for (a) metalimnion depth (Depth), (b) lake number (LN), (c) Wedderburn number (W), (d) Schmidt stability (St) and lake water temperature measurements from August 2012 to June 2014 at Bangong Co. (e) shows the isothermals at Bangong Co; numbers represent the lake water temperatures
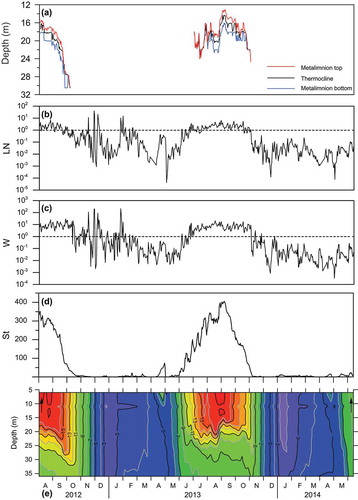
There were slight differences in the thermal regimes at Bangong Co between the two hydrological years (August 2012~July 2013 and August 2013~June 2014). The temperature throughout the water column in the winter of 2013 (December 2013~April 2014) was clearly lower than that in the winter of 2012 (December 2012~April 2013). ) shows that the lake water temperature was below 2°C at depths above 27 m in January 2014, but this was not the case during the same period in the winter of 2012. The lowest water temperature was 0.78°C at 10 m on 22 December 2012, in the winter of 2012. In comparison, the lowest water temperature was 0.26°C at the same depth on 2 January 2014, in the winter of 2013. The summer temperature throughout the water column was lower in 2013 than in 2012. For example, in August 2012, the lowest water temperature was observed at the deepest temperature logger, which averaged at ~9°C; while in August 2013, the lowest temperature was also observed at the deepest temperature logger, which averaged at ~7.7°C. Moreover, the date of complete mixing was slightly different between the two hydrological years (). The complete mixing during the period of the autumn overturn in 2012 occurred on 17 October 2012, but it was ~10 d later in 2013 (31 October 2013) () and (b)). The complete mixing of the water column in spring occurred on 3 May 2013, in comparison to 23 April in 2014.
Figure 3. (a) and (b) show the observed temperature variation at 5 m (the uppermost temperature logger, T-5 m, blue line), 35 m (the lowest temperature logger, T-35 m, black line) and 23 m (the average depth of observed metalimnion, T-23 m, red line) during September 2012~May 2013 and September 2013~May 2014, respectively; (c) and (d) show the maximum wind speed during September 2012~May 2013 and September 2013~May 2014, respectively
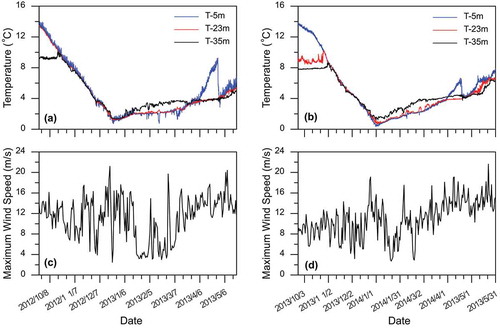
4.2 Temperature profiles at Dagze Co
Continuous temperature measurements at 18 different horizons at Dagze Co (from August 2012 to July 2015) indicate that it is a meromictic lake. The thermal regimes did not change substantially from 2012 to 2015. The water below 23 m maintained a temperature of ~3°C and was isolated from the water above, indicating that the lake remained meromictic during the 3 years of the study ()). A secondary thermocline occurred at ~5 m within the mixolimnion during the summer period.
Figure 4. Comparison of Lake Analyzer calculations for (a) metalimnion depth (Depth), (b) lake number (LN), (c) Wedderburn number (W), (d) Schmidt stability (St) and lake water temperature measurements from August 2012 to July 2015 at Dagze Co. (e) shows the isothermals at Dagze Co; numbers represent the lake water temperatures
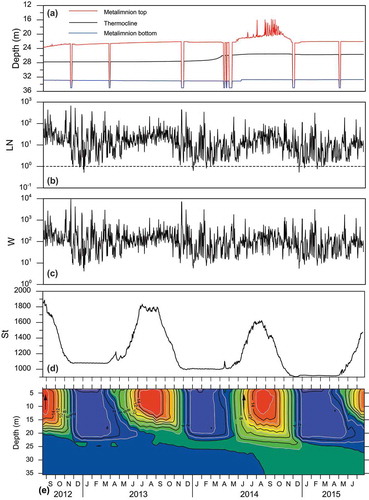
There are also slight differences in the temperature profiles from 2012 to 2015 (). The most evident change is that the water temperature in the monimolimnion increased gradually from 2012 to 2015 ()). The bottom water temperature at 35 m, the depth of the deepest data logger, increased from 2.8°C in 2012 to 3.2°C in 2014 ()). The spring and autumn overturn varied substantially in the mixolimnion from 2012 to 2015. A clear and abrupt spring overturn in the mixolimnion was observed in 2014 but not in 2013 or 2015 ()). The autumn overturn in the mixolimnion occurred much earlier in 2014 (19 October) than in 2012 (6 November) or 2013 (13 November) (), (d) and (f)). The observed depth of the metalimnion between the epilimnion and the hypolimnion varied during the summer in the 3 years of the study. We measured the depths between 12°C and 4°C from August to October during the 3 years and found that they occurred at 17 and 21 m in 2012, 13 and 21 m in 2013, and 17 and 25 m in 2014, indicating that the metalimnion expanded markedly from 2012 to 2014.
Figure 5. Comparison of extreme wind speed and lake water temperature variations within the mixolimnion at Dagze Co. (a), (c) and (e) represent extreme wind speed at Xainza weather station during September 2012~June 2013, September 2013~June 2014 and September 2014~June 2015, respectively; (b), (d) and (f) show temperature variations at 4 m (blue line), 10 m (red line) and 19 m (black line) within the mixolimnion during September 2012~June 2013, September 2013~June 2014 and September 2014~June 2015. A clear overturn occurred in April 2014 which coincided with the occurrence of stronger wind
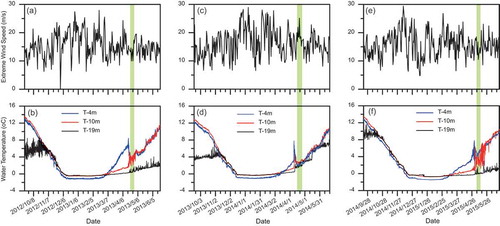
5 Discussion
5.1 Thermal regimes at Bangong and Dagze Co
The water body in the eastern part of the Bangong Co is fresh (salinity was 0.47 g/L throughout the water column, measured on 27 July 2012). The density of water is a function of temperature, pressure and salinity (Millero et al. Citation1976), and the effect of the pressure can be ignored in relatively shallow lakes. As mentioned, Bangong Co is fresh in the eastern basin; hence, the water density of Bangong Co is mainly determined by temperature. Bangong Co is a typical dimictic lake, with the lake water stratified in summer due to the increased density gradient in the water column, overturned in spring and autumn and a weak inverse stratification in winter. The mechanisms for the thermal regimes in a dimictic lake have been studied for decades (Bennett Citation1978), and we will not repeat this information here. However, there is a weak stratification in spring (April) for Bangong Co and then the lake experienced complete mixing before the stable summer stratification. The water column mixing after the short-term spring stratification is ascribed to the rapid decrease in the surface water temperature. Specifically, the wind-induced turbulence caused the heat exchange between the warm subsurface water and the cold bottom water, although the details of these findings are beyond the scope of this paper (Lazhu et al. Citationsubmitted).
Subsequently, the temperature of the near-surface water rapidly decreased from ~7 to ~4°C between late April and early May in 2013, while the bottom water warmed slowly from 3.8 to 4.2°C during the same period. The ice phenology events from moderate resolution imaging spectroradiometer (MODIS) data reveal that the date at which the ice disappears for Bangong Co is around 21~26 April (Kropáček et al. Citation2013, Wang et al. Citation2020a). After the disappearance of lake ice, the average wind speed exceeds 5.5 m/s based on the NASDE weather station observations, and strong winds cause mechanical mixing through the input of wind energy (Churchill and Charles Kerfoot Citation2007). A similar phenomenon has also been observed in some other monitored lakes across the TP, such as Gongzhu Co (unpublished data). No similar thermal stratification dynamics is captured from the lakes in low-altitude regions, such as Lake Goitsche in Germany (Boehrer and Schultze Citation2008), or tropical regions, such as Huguang Maar Lake in China (Wang et al. Citation2012).
At Dagze Co, the lake water density is markedly affected by salinity. We did not measure water salinity continuously due to logistical difficulties; instead, we measured the salinity when we visited the lake during the fieldwork. Salinity in the water column of Dagze Co increased from the surface (14.69 g/L) to the bottom (21.38 g/L), while the water temperature decreased from the surface (15.31°C) to the bottom (2.82°C) (measured on 18 August 2012); as a result, the bottom water was 6.7 kg/m3 denser than the surface water. Mixing only occurs when the density of the surface water increases sufficiently with roughly comparable density to the bottom water. The overturn is also facilitated by the input of kinetic energy through wind stress at the lake surface. However, bringing water to the surface that is denser by 6.7 kg/m3 would typically require a temperature decrease of ~35°C. The substantial difference in density between the surface water and the deep water at Dagze Co prevented wind-induced overturning for the whole water column. Consequently, such a vertical salinity gradient would affect the thermal structure and inhibit the downward mixing of the heat.
Although we do not have salinity measurements throughout the monitoring period, the temperature-depth profile ()) indicates that a permanent monimolimnion existed below the chemocline. The water within the monimolimnion was cold and did not mix with the water above. The mixolimnion (water above the chemocline) indicated a thermal regime similar to that of dimictic lakes. During the summer, the lake was stratified because the increased solar insolation warmed the surface water, lowering its density. The water above the chemocline mixed completely during the autumn from 2012 to 2014.
The origin of the higher salinity at the bottom of Dagze Co is not clear. It may be caused by long-term evaporation processes and partly attributable to groundwater containing dissolved salts from the bedrock flowing into the lake bottom. Groundwater is usually cool and may have affected the thermal regime in the lake water. However, our current understanding of the groundwater at Dagze Co is limited. The higher salinity at Dagze Co may also result from lake evolution under the influence of dry climate conditions in this region. A series of palaeo-shorelines above the modern lake level suggest that the lake was much deeper and larger in the past (Qiao et al. Citation2010); for example, a 91 m higher lakeshore was identified at Dagze Co by Lehmkuhl and Haselein (Citation2000). Lake water was likely lost through evaporation, enriching the major ions in the lake water. Because the inflow rivers bring freshwater (Bogcarg Zangbo, with salinity of 0.62 g/L (Liu et al. Citation2013)) to the lake surface or near the surface, the upper lake water would be expected to be relatively fresh, whereas the deep water should be more saline. More investigations are required to test these assumptions.
5.2 Comparison of mixing regimes between in situ data and modeling results
We used Lake Analyzer to evaluate various indices for stratification at Bangong Co and Dagze Co and compared the results with our observations. As Lake Analyzer provides various parameters describing the lake thermal regimes, we focused on two parameters that corresponded to our observations: Schmidt stability (St) and Wedderburn number (W). We found that the two parameters were highly consistent with our observations at seasonal and interannual scales ().
The indices for stratification calculated using Lake Analyzer were consistent with our observations at Bangong Co (). At Bangong Co, W varied substantially at the seasonal scale from 2012 to 2014 ()). During the summer stratification, the indices for lake stratification (St and W) suggest that the lake water column was in a very stable state. St was much higher than 0, indicating strong resistance to mechanical mixing ()); W remained higher than 1 ()), suggesting a very small probability of upwelling. As the water column became less stable during the autumn, St and W decreased correspondingly. During the autumn overturn, St approached 0, and W fell below 1. During the winter, when the lake was covered by ice, St remained close to 0, and W varied considerably. During the spring overturn, both W and St increased gradually. Lake Analyzer successfully reproduced the difference in the date of autumn overturn at Bangong Co. For example, in the autumns of 2012 and 2013, the dates when W approached 1 and St decreased to its minimum value differed. W decreased below 1 on 18 October 2012, which coincided with the autumn overturn in the lake. In comparison, during the autumn of 2013, W decreased below 1 on October 30, coinciding with the late autumn overturn.
At Dagze Co, the three indices for lake stratification suggest that the lake maintained a meromictic state during the monitoring period (). St varied from 980 to 1860 ()), and W consistently exceeded 1 ()), indicating that the lake was permanently stratified from 2012 to 2015. St and W varied considerably on both seasonal and interannual scales. St reached its maximum values during the summers and minimum values during the winters ()). From August 2012 to July 2015, St showed general decreasing trends. The maximum value of St was 1860 in 2012, and it decreased to 1781 in 2013 and 1600 in 2014 ()). The minimum value of St also decreased, from 1077 to 1002 and then to 900 from 2012 to 2015. We observed a secondary thermocline within the mixolimnion at a depth of ~5 m.
However, Lake Analyzer did not fully reproduce the changes in the mixolimnion. For example, the calculated depth of the metalimnion was not consistent with the temperature measurements (). The inconsistency between the observations and Lake Analyzer calculations likely results from the lack of continuous measurements of lake water salinity during the observation period.
5.3 Influence of atmospheric conditions on the lake thermal structure at Bangong Co
We carefully examined the data from Shiquanhe weather station to assess its relationship with the thermal structure at Bangong Co during the monitoring period. The mean air temperature in August 2012~July 2013 and August 2013–June 2014 was 0.85 and 1.44°C, respectively ()). Here we attempt to gain an understanding of the causes of the lower summer water temperature and the relatively lower winter water temperature in 2013~2014. One possibility is that the relatively lower summer water temperature at Bangong Co results from more glacial meltwater input under the relatively higher average air temperature in 2013~2014, as the lake is mainly fed by glacial meltwater. The input of glacial meltwater into Bangong Co would lower the temperature of the lake water. In comparison, the relatively lower air temperature in the summer of 2012 would bring less meltwater into Bangong Co, which did not decrease lake water temperature as much as in the summer of 2013. The 30-year average temperature (1983–2012) at Shiquanhe is 1.14°C. The mean air temperature in August 2013–June 2014 is higher than the 30-year average, while the mean air temperature in August 2012–July 2013 is lower than the 30-year average. We are presently trying to access more data, such as the runoff and modern glacier monitoring data, to support the speculative conclusion that the catchment glaciers contribute the most input.
Figure 6. Comparison of the average wind speed, daily mean temperature, and temperature variation at Bangong Co from August 2012 to June 2014. (a) Average wind speed and (b) daily mean temperature at Shiquanhe weather station. (c) The isothermals at Bangong Co; numbers represent the lake water temperatures, as in
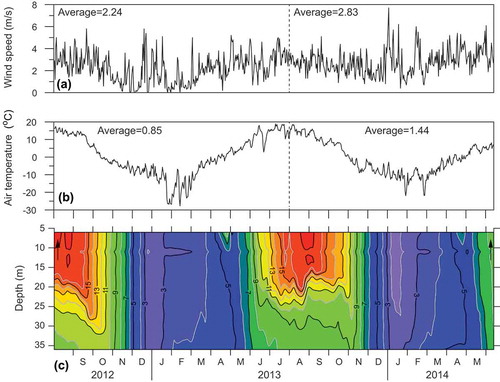
Bangong Co has three major inflow rivers, and the two largest inflow rivers are Chiao Ho and Makha. Unfortunately, there is no available runoff data for Chiao Ho. For Makha, the data are accessible. However, a hydroelectric power station was built in 2004 near the lake inlet that may have an unavoidable impact on surface runoff. Xiongcaigangri is the largest glacier in the catchment of Bangong Co (Li et al. Citation2015); however, the modern glacier monitoring started in 2016 (Shengqiang Jin, personal communication). Hence, the evaluation of catchment glacier meltwater contribution to the temperature profile of Bangong Co remains unclear. Nevertheless, the changes in lake thermal structure suggest that the lakes at high altitude are very sensitive to climate change.
The average wind speed at Shiquanhe station was 2.24 m/s in August 2012~ July 2013 and 2.83 m/s in August 2013–June 2014 ()). Wind is one of the most important actions causing lake mixing, and here we ascribed the timing of overturn to wind speed. Higher instantaneous wind speed will cause the date of the overturn to occur earlier, as supported by the results of sensitivity experiments. Therefore, the later autumn overturn in 2013 was not likely the result of the average wind speed. In the autumn of 2012, the entire water column mixed on 18 October, coinciding with the strong wind in mid to late October. In 2013, the complete vertical mixing occurred on 1 November, also coinciding with the strong wind in late October. We speculated that the late autumn overturn in 2013 at Bangong Co could be attributed to a lack of strong winds in October 2013. In autumn, the density of the lake water increased as the lake surface water cooled, which decreased the density difference between the upper and deeper parts of the water column. In terms of the instantaneous near-surface wind speed, for example, the occurrence of gusts likely triggered the autumn overturn.
5.4 Influence of atmospheric conditions on the lake thermal structure at Dagze Co
We observed the changes in the meteorological data collected at Xainza station and compared them with the thermal structures at Dagze Co. The gradual expansion of the metalimnion from 2012 to 2014 can be attributed to an increase in the heat transfer coefficient related to the average wind speed (). The average wind speed during the summer months (June~September) increased from 2.80 m/s in 2012 to 2.96 m/s and 3.20 m/s in 2013 and 2014, respectively ()). This increase was likely related to the depth of the metalimnion bottom, which increased from 21 m in the summer of 2012 to 24 m and 27 m in the summer of 2013 and 2014, respectively. The increase in the average wind speed likely reduced the stability of the water column within the lake, transferring more heat to deep water and expanding the permanent metalimnion. The expansion of the metalimnion likely increased the water temperature in the monimolimnion.
Figure 7. Comparison of meteorological parameters at Xainza station with temperature variation at Dagze Co from August 2012 to July 2015. (a) Average wind speed, (b) daily mean temperature, and (c) daily precipitation at Xainza. (d) The isothermals at Dagze Co; numbers represent the lake water temperatures
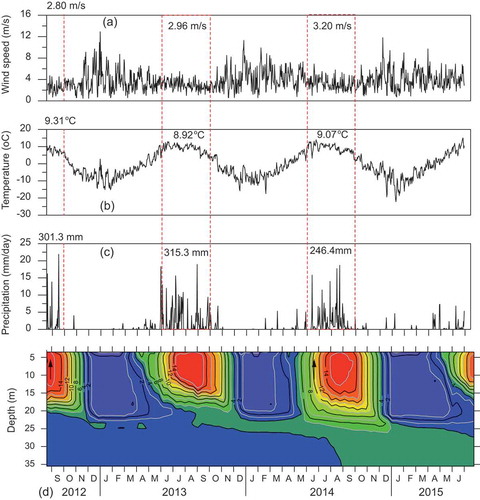
Furthermore, we speculated that the clear spring overturn in 2014 could be attributed to the influence of extreme winds (). During April 2014, extreme winds exceeding 20 m/s were recorded over a ~15-d period beginning on 10 April 2014 ()). In contrast, gales (>20 m/s) rarely occurred in April 2013 and April 2015 and were likely insufficient to overturn the water column in the mixolimnion due to the salinity gradient. We also accessed the shortwave radiation data from the China Meteorological Forcing Dataset (He et al. Citation2020) for the stratification period (from June to September) in 2013 and 2014, and found that there was no difference between the years. Hence, it is deduced that the winds influence the thermal structure within the lake by affecting the heat transfer.
In addition to the direct comparison of temperature profiles and the meteorological data, quantitative analyses of energy budgets are usually used to assess the impact of wind in influencing the lake thermal structure. Wind is one of the most important driving forces generating turbulence, and influencing turbulent mixing intensity (Wang et al. Citation2017). Lake mixing occurs when the turbulent kinetic energy (TKE), stored in the topmost layers, exceeds a potential energy threshold (Magee and Wu Citation2017), hence TKE balancing could be used as a tool to estimate the efficiency of the vertical mixing (Wüest et al. Citation2000). Some previous studies have calculated TKE and compared it with the water column stability and diffusivity (Wüest et al. Citation2000, Saber et al. Citation2018). However, the TKE calculation could not be done in the present study due to the unavailability of necessary data. Hence, we currently ascribe the changes in thermal structure in Dagze Co to wind speed. We recognize the importance of quantitative analyses to understand the mixing process and plan to focus on energy budgets and transfer in future research.
5.5 Rescaled range analysis of lake water temperature observations
We calculated the Hurst exponent (H) to assess the persistence of the temperature variability in various horizons at Bangong Co and Dagze Co (). At Bangong Co, H values were greater than 0.78 above 16 m depth, while H values varied between 0.64 and 0.78 below 16 m depth (). The generally high H values (>0.5) indicate that the dimictic condition would persist at Bangong Co. Throughout the water column, the transition from higher H values to lower H values occurred at 16 m, indicating strong persistence above this depth and relatively weak persistence below the thermocline.
Figure 8. Hurst exponent calculated from rescaled range (R/S) analysis of observed temperatures at Bangong Co (open circles) and Dagze Co (solid circles)
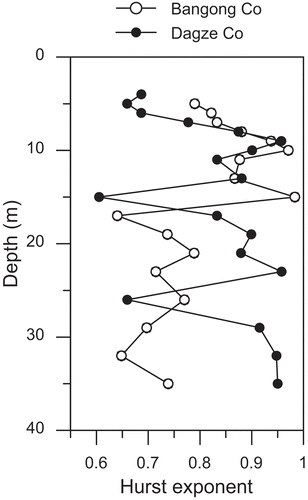
If the climate warms in the future on the western Tibetan Plateau, the dimictic condition will tend not to change. However, the temperature below the thermocline may demonstrate more variability, most likely during the transition from summer stratification to autumn overturn. Note that Bangong Co is mainly fed by glacial meltwater. If the air temperature increases in the future, the temperature variations along the water column will be difficult to predict as (1) there will be stronger sensible heat exchange between the atmosphere and the water; and (2) the lake water will be cooled by more meltwater input as the warmer climate will likely melt more glaciers. Due to the limitations of the currently available dataset, we can hardly predict how the lake water temperature may vary in the future.
At Dagze Co, H values fluctuated between 0.60 and 0.96, with three horizons of lower H values occurring above 5 m, at 15 m and at 26 m (). The generally higher H values suggest that the meromixis would persist at Dagze Co. The lower H values at Dagze Co coincided with the secondary thermocline within the mixolimnion, the upper limit and lower limit of the permanent thermocline, indicating relatively weak persistence at the three horizons. Lower H values also suggest that the patterns of temperature variation likely change. For example, a relatively lower H value of 0.6052 at 15 m suggested the temperature tended to change, which was observed from 2012 to 2015. The lower H value at 26 m suggested the expansion of the thermocline during the 3 year study period. If the climate warms in the future on the central Tibetan Plateau, which would evaporate more water from the lake and drive the surface water to become more saline, the meromixis may persist; however, the thermocline will likely expand even deeper.
6 Summary
We launched a programme to monitor lake water temperature variability on the Tibetan Plateau since 2012 to investigate the thermal regimes and structures of lakes. Current results show that the thermal regimes of a dimictic lake (Bangong Co) and a meromictic lake (Dagze Co) have not undergone significant changes. There are slight differences in different hydrological years at the two lakes. At Bangong Co, (1) both the average summer water temperature and the average winter water temperature were cooler in 2013~2014 than in 2012~2013, and (2) the spring overturn occurred ~10 d earlier in 2013~2014 than in 2012~2013, while the autumn overturn occurred ~14 d later in 2013~2014 than in 2012~2013. At Dagze Co, (1) the water temperature in the monimolimnion increased slightly from 2012 to 2015, (2) the metalimnion expanded gradually from 2012 to 2015, and (3) a clear spring overturn was observed at the mixolimnion in 2014 but not in 2013 or 2015.
We compared our water temperature measurements with meteorological data and adopted Lake Analyzer calculations to obtain a more detailed understanding of the lake thermal structure. Changes in the lake water temperature at Bangong Co might have resulted from variation in air temperature, which influenced glacier melt and freshwater input to the lake. The difference in overturn dates at Bangong Co could be attributed to the occurrence of extreme winds. As Dagze Co is a brackish lake, we speculate that changes in the lake water temperature resulted from variations in average wind speed that affect the heat transfer. We performed rescaled range (R/S) analysis on temperature measurements at the two lakes and found evidence that the lake thermal and mixing dynamics will persist in the near future.
The present observation of the water temperature over the Tibetan Plateau is very limited, covering only two lakes and 3 years. Therefore, our conclusions on the variation in lake water temperature profiles remain rather speculative. We have expanded our observation to more lakes in the past few years, which will help us to understand the mechanisms for changes in lake water temperature in the future.
Acknowledgements
The authors thank Dr Yue He, Mr Erlei Zhu and Mr Maohui Liang for their assistance in retrieving the temperature loggers deployed at the two lakes, and thank Dr Junbo Wang and Dr Yanbin Lei for providing bathymetry data of Bangong Co and lake level data of Dagze Co, respectively. We appreciate Prof. Hucai Zhang and two anonymous reviewers for thoughtful advice and constructive comments. This work was financially supported by the National Key R&D Program of China (Grant No. 2018YFA0606400), Natural Science Foundation of China (Grant No. 41601205) and China Postdoctoral Science Foundation (Grant No. 2016M590142).
Disclosure statement
No potential conflict of interest was reported by the authors.
Additional information
Funding
References
- Adrian, R., et al., 1999. Effects of ice duration on plankton succession during spring in a shallow polymictic lake. Freshwater Biology, 41 (3), 621–634. doi:https://doi.org/10.1046/j.1365-2427.1999.00411.x.
- Adrian, R., et al., 2009. Lakes as sentinels of climate change. Limnology and Oceanography, 54 (6part2), 2283–2297. doi:https://doi.org/10.4319/lo.2009.54.6_part_2.2283.
- Assessment, M.E., 2005. Ecosystems and human well-being: wetlands and water. Washington, DC: World Resources Institute.
- Bennett, E.B., 1978. Characteristics of the thermal regime of lake superior. Journal of Great Lakes Research, 4 (3–4), 310–319. doi:https://doi.org/10.1016/S0380-1330(78)72200-8.
- Boehrer, B. and Schultze, M., 2008. Stratification of lakes. Reviews of Geophysics, 46 (2), 2. doi:https://doi.org/10.1029/2006RG000210.
- Chen, H., et al., 2009. High methane emissions from a littoral zone on the Qinghai-Tibetan Plateau. Atmospheric Environment, 43 (32), 4995–5000. doi:https://doi.org/10.1016/j.atmosenv.2009.07.001.
- Churchill, J.H. and Charles Kerfoot, W., 2007. The impact of surface heat flux and wind on thermal stratification in Portage Lake, Michigan. Journal of Great Lakes Research, 33 (1), 143–155. doi:https://doi.org/10.3394/0380-1330(2007)33[143:TIOSHF]2.0.CO;2.
- Ficker, H., Luger, M., and Gassner, H., 2017. From dimictic to monomictic: empirical evidence of thermal regime transitions in three deep alpine lakes in Austria induced by climate change. Freshwater Biology, 62 (8), 1335–1345. doi:https://doi.org/10.1111/fwb.12946.
- Fontes, J.-C., Gasse, F., and Gibert, E., 1996. Holocene environmental changes in Lake Bangong basin (Western Tibet). Part 1: chronology and stable isotopes of carbonates of a Holocene lacustrine core. Palaeogeography, Palaeoclimatology, Palaeoecology, 120 (1–2), 25–47. doi:https://doi.org/10.1016/0031-0182(95)00032-1.
- Fritz, S.C., 2008. Deciphering climatic history from lake sediments. Journal of Paleolimnology, 39 (1), 5–16. doi:https://doi.org/10.1007/s10933-007-9134-x.
- Gerten, D. and Adrian, R., 2002. Species-specific changes in the phenology and peak abundance of freshwater copepods in response to warm summers. Freshwater Biology, 47 (11), 2163–2173. doi:https://doi.org/10.1046/j.1365-2427.2002.00970.x.
- Gulati, R.D., Zadereev, E.S., and Degermendzhi, A.G., 2017. Ecology of meromictic lakes. Cham, Switzerland: Springer.
- He, J., et al., 2020. The first high-resolution meteorological forcing dataset for land process studies over China. Scientific Data, 7 (1), 25. doi:https://doi.org/10.1038/s41597-020-0369-y.
- Hurst, H., 1951. Long-term storage capacity of reservoirs. Transactions of the American Society of Civil Engineers, 116 (1), 770–799.
- Hutchinson, G.E. and Edmondson, Y.H., 1957. A treatise on limnology. New York: John Wiley & Sons.
- Idso, S.B., 1973. On the concept of lake stability. Limnology and Oceanography, 18 (4), 681–683. doi:https://doi.org/10.4319/lo.1973.18.4.0681.
- Imberger, J., 1985. The diurnal mixed layer. Limnology and Oceanography, 30 (4), 737–770. doi:https://doi.org/10.4319/lo.1985.30.4.0737.
- Imberger, J. and Patterson, J.C., 1989. Physical limnology. In: J.W. Hutchinson and T.Y. Wu, eds. Advances in applied mechanics. Elsevier, Vol. 27, 303–475. https://doi.org/10.1016/S0065-2156(08)70199-6
- Immerzeel, W.W., et al., 2020. Importance and vulnerability of the world’s water towers. Nature, 577 (7790), 364–369. doi:https://doi.org/10.1038/s41586-019-1822-y.
- Khan, A., et al., 2014. How large is the upper indus basin? The pitfalls of auto-delineation using DEMs. Journal of Hydrology, 509, 442–453. doi:https://doi.org/10.1016/j.jhydrol.2013.11.028.
- Kraemer, B.M., et al., 2015. Morphometry and average temperature affect lake stratification responses to climate change. Geophysical Research Letters, 42 (12), 4981–4988. doi:https://doi.org/10.1002/2015GL064097.
- Kropáček, J., et al., 2013. Analysis of ice phenology of lakes on the Tibetan Plateau from MODIS data. The Cryosphere, 7 (1), 287–301. doi:https://doi.org/10.5194/tc-7-287-2013.
- Lazhu, et al., Submitted. A new finding on the prevalence of rapid water warming during lake ice melting on the Tibetan Plateau.
- Leavitt, P.R. and Hodgson, D.A., 2001. Sedimentary pigments. In: J.P. Smol, ed. Tracking environmental change using lake sediments: terrestrial, algal, and siliceous indicators. Dordrecht: Springer Netherlands, 295–325.
- Lehmkuhl, F. and Haselein, F., 2000. Quaternary paleoenvironmental change on the Tibetan Plateau and adjacent areas (Western China and Western Mongolia). Quaternary International, 65-66, 121–145. doi:https://doi.org/10.1016/S1040-6182(99)00040-3
- Lehner, B., Verdin, K., and Jarvis, A., 2006. HydroSHEDS technical documentation, version 1.0. Washington, DC: World Wildlife Fund US, 1–27.
- Lei, Y., et al., 2017. Lake seasonality across the Tibetan Plateau and their varying relationship with regional mass changes and local hydrology. Geophysical Research Letters, 44 (2), 892–900. doi:https://doi.org/10.1002/2016GL072062.
- Lei, Y., et al., 2019. Thermal regime, energy budget and lake evaporation at Paiku Co, a deep alpine lake in the central Himalayas. Hydrology and Earth System Sciences, 2019, 1–27.
- Li, W., et al., 2001. Meromixis in Zige Tangco, central Tibetan Plateau. Science in China Series D: Earth Sciences, 44 (S1), 338–342. doi:https://doi.org/10.1007/BF02912004.
- Li, Z., et al., 2015. Changes in the glacier extent and surface elevation in Xiongcaigangri region, Southern Karakoram Mountains, China. Quaternary International, 371, 67–75. doi:https://doi.org/10.1016/j.quaint.2014.12.004.
- Liu, S., et al., 2013. The depositional environment and organic sediment component of Dagze Co, a saline lake in Tibet, China. Acta Ecol Sin, 33 (18), 5785–5793. doi:https://doi.org/10.5846/stxb201306071419.
- Livingstone, D.M., 2003. Impact of Secular Climate Change on the Thermal Structure of a Large Temperate Central European Lake. Climatic Change, 57 (1/2), 205–225. doi:https://doi.org/10.1023/A:1022119503144.
- Macintyre, S., et al., 2009. Climate-related variations in mixing dynamics in an Alaskan arctic lake. Limnology and Oceanography, 54 (6, part2), 2401–2417. doi:https://doi.org/10.4319/lo.2009.54.6_part_2.2401.
- Macintyre, S., 2013. Climatic variability, mixing dynamics, and ecological consequences in the African Great Lakes. In: C.R. Goldman, M. Kumagai, and R.D. Robarts, eds. Climatic change and global warming of inland waters: impacts and mitigation for ecosystems and societies. Wiley, 311–336.
- Magee, M.R. and Wu, C.H., 2017. Response of water temperatures and stratification to changing climate in three lakes with different morphometry. Hydrol. Earth Syst. Sci., 21 (12), 6253–6274. doi:https://doi.org/10.5194/hess-21-6253-2017.
- Magnuson, J.J., et al., 2000. Historical trends in lake and river ice cover in the northern hemisphere. Science, 289 (5485), 1743–1746. doi:https://doi.org/10.1126/science.289.5485.1743.
- Messager, M.L., et al., 2016. Estimating the volume and age of water stored in global lakes using a geo-statistical approach. Nature Communications, 7 (1), 13603. doi:https://doi.org/10.1038/ncomms13603.
- Meyers, P.A. and Lallier-Vergés, E., 1999. Lacustrine sedimentary organic matter records of late quaternary paleoclimates. Journal of Paleolimnology, 21 (3), 345–372. doi:https://doi.org/10.1023/A:1008073732192.
- Millero, F.J., Gonzalez, A., and Ward, G.K., 1976. The density of seawater solutions at one atmosphere as a function of temperature and salinity. Journal of Marine Research, 34 (1), 61–93.
- Mischke, S., et al., 2010. Lateglacial and Holocene variation in aeolian sediment flux over the northeastern Tibetan Plateau recorded by laminated sediments of a saline meromictic lake. Journal of Quaternary Science, 25 (2), 162–177. doi:https://doi.org/10.1002/jqs.1288.
- Mishra, A.K., Özger, M., and Singh, V.P., 2009. An entropy-based investigation into the variability of precipitation. Journal of Hydrology, 370 (1–4), 139–154. doi:https://doi.org/10.1016/j.jhydrol.2009.03.006.
- Mueller, D.R., et al., 2009. High Arctic lakes as sentinel ecosystems: cascading regime shifts in climate, ice cover, and mixing. Limnology and Oceanography, 54 (6, part2), 2371–2385. doi:https://doi.org/10.4319/lo.2009.54.6_part_2.2371.
- North, C.P. and Halliwell, D.I., 1994. Bias in estimating fractal dimension with the rescaled-range (R/S) technique. Mathematical Geology, 26 (5), 531–555. doi:https://doi.org/10.1007/BF02089240.
- O’Reilly, C.M., et al., 2015. Rapid and highly variable warming of lake surface waters around the globe. Geophysical Research Letters, 42 (24), 10,773–10,781.
- Paerl, H.W., et al., 1975. Seasonal nitrate cycling as evidence for complete vertical mixing in Lake Tahoe, California-Nevada. Limnology and Oceanography, 20 (1), 1–8. doi:https://doi.org/10.4319/lo.1975.20.1.0001.
- Qiao, C., et al., 2010 Lake shrinkage analysis using spectral-spatial coupled remote sensing on Tibetan Plateau. In: IEEE International Geoscience and Remote Sensing Symposium, 25–30 July 2010 Honolulu, HI, 926–929.
- Read, J.S., et al., 2011. Derivation of lake mixing and stratification indices from high-resolution lake buoy data. Environmental Modelling and Software, 26 (11), 1325–1336. doi:https://doi.org/10.1016/j.envsoft.2011.05.006.
- Roemmich, D. and Mcgowan, J., 1995. Climatic warming and the decline of zooplankton in the California Current. Science, 267 (5202), 1324–1326. doi:https://doi.org/10.1126/science.267.5202.1324.
- Saber, A., James, D.E., and Hayes, D.F., 2018. Effects of seasonal fluctuations of surface heat flux and wind stress on mixing and vertical diffusivity of water column in deep lakes. Advances in Water Resources, 119, 150–163. doi:https://doi.org/10.1016/j.advwatres.2018.07.006
- Sahoo, G.B., et al., 2016. Climate change impacts on lake thermal dynamics and ecosystem vulnerabilities. Limnology and Oceanography, 61 (2), 496–507. doi:https://doi.org/10.1002/lno.10228.
- Schindler, D.W., 1997. Widespread effects of climatic warming on freshwater ecosystems in North America. Hydrological Processes, 11 (8), 1043–1067. doi:https://doi.org/10.1002/(SICI)1099-1085(19970630)11:8<1043::AID-HYP517>3.0.CO;2-5.
- Schmidt, W., 1928. Über Die Temperatur-Und Stabili-Tätsverhältnisse Von Seen. Geografiska Annaler, 10 (1–2), 145–177.
- Sharma, S., et al., 2019. Widespread loss of lake ice around the Northern Hemisphere in a warming world. Nature Climate Change, 9 (3), 227–231. doi:https://doi.org/10.1038/s41558-018-0393-5.
- Smol, J.P., et al., 2005. Climate-driven regime shifts in the biological communities of arctic lakes. Proceedings of the National Academy of Sciences of the United States of America, 102 (12), 4397. doi:https://doi.org/10.1073/pnas.0500245102.
- Straile, D., 2002. North atlantic oscillation synchronizes food-web interactions in central European lakes. Proceedings Biological Sciences/The Royal Society, 269 (1489), 391–395. doi:https://doi.org/10.1098/rspb.2001.1907.
- Tan, Z., Yao, H., and Zhuang, Q., 2018. A small temperate lake in the 21st century: dynamics of water temperature, ice phenology, dissolved oxygen, and chlorophyll a. Water Resources Research, 54 (7), 4681–4699. doi:https://doi.org/10.1029/2017WR022334.
- Thompson, R., 1980. Response of a numerical model of a stratified lake to wind stress. In: Proceedings of the second international symposium stratified flows, IAHR, 1980 Trondheim, Norway.
- Wan, W., et al., 2016. A lake data set for the Tibetan Plateau from the 1960s, 2005, and 2014. Scientific Data, 3 (1), 160039. doi:https://doi.org/10.1038/sdata.2016.39.
- Wang, B., et al., 2017. Physical controls on half-hourly, daily, and monthly turbulent flux and energy budget over a high-altitude small lake on the Tibetan Plateau. Journal of Geophysical Research: Atmospheres, 122 (4), 2289–2303.
- Wang, B., et al., 2020a. Quantifying the evaporation amounts of 75 high-elevation large dimictic lakes on the Tibetan Plateau. Science Advances, 6 (26), eaay8558. doi:https://doi.org/10.1126/sciadv.aay8558.
- Wang, J., et al., 2009. Investigation of bathymetry and water quality of Lake Nam Co, the largest lake on the central Tibetan Plateau, China. Limnology, 10 (2), 149–158. doi:https://doi.org/10.1007/s10201-009-0266-8.
- Wang, J., et al., 2019. Spatial and temporal variations in water temperature in a high-altitude deep dimictic mountain lake (Nam Co), central Tibetan Plateau. Journal of Great Lakes Research, 45 (2), 212–223. doi:https://doi.org/10.1016/j.jglr.2018.12.005.
- Wang, J., et al., 2020b. Seasonal stratification of a deep, high-altitude, dimictic lake: nam Co, Tibetan Plateau. Journal of Hydrology, 584, 124668. doi:https://doi.org/10.1016/j.jhydrol.2020.124668.
- Wang, L., et al., 2012. The East Asian winter monsoon over the last 15,000 years: its links to high-latitudes and tropical climate systems and complex correlation to the summer monsoon. Quaternary Science Reviews, 32, 131–142. doi:https://doi.org/10.1016/j.quascirev.2011.11.003.
- Wang, M., Hou, J., and Lei, Y., 2014. Classification of Tibetan lakes based on variations in seasonal lake water temperature. Chinese Science Bulletin, 59 (34), 4847–4855. doi:https://doi.org/10.1007/s11434-014-0588-8.
- Wang, W., et al., 2018. Global lake evaporation accelerated by changes in surface energy allocation in a warmer climate. Nature Geoscience, 11 (6), 410–414. doi:https://doi.org/10.1038/s41561-018-0114-8.
- Wetzel, R.G., 2001. Limnology: lake and river ecosystems. San Diego, CA: Gulf Professional Publishing. https://www.sciencedirect.com/science/article/pii/B9780080574394500022
- Winder, M. and Schindler, D.E., 2004. Climate change uncouples trophic interactions in an aquatic ecosystem. Ecology, 85 (8), 2100–2106. doi:https://doi.org/10.1890/04-0151.
- Woolway, R.I., et al., 2020. Global lake responses to climate change. Nature Reviews Earth & Environment., 1 (8), 388–403. doi:https://doi.org/10.1038/s43017-020-0067-5.
- Woolway, R.I. and Merchant, C.J., 2019. Worldwide alteration of lake mixing regimes in response to climate change. Nature Geoscience, 12 (4), 271–276. doi:https://doi.org/10.1038/s41561-019-0322-x.
- Wüest, A., Piepke, G., and Van Senden, D.C., 2000. Turbulent kinetic energy balance as a tool for estimating vertical diffusivity in wind-forced stratified waters. Limnology and Oceanography, 45 (6), 1388–1400. doi:https://doi.org/10.4319/lo.2000.45.6.1388.
- Yan, F., et al., 2018. Lakes on the Tibetan Plateau as conduits of greenhouse gases to the atmosphere. Journal of Geophysical Research: Biogeosciences, 123 (7), 2091–2103.
- Zhang, G., et al., 2019. A robust but variable lake expansion on the Tibetan Plateau. Science Bulletin, 64 (18), 1306–1309. doi:https://doi.org/10.1016/j.scib.2019.07.018.
- Zhang, G., et al., 2020. Response of Tibetan Plateau lakes to climate change: trends, patterns, and mechanisms. Earth-Science Reviews, 208, 103269. doi:https://doi.org/10.1016/j.earscirev.2020.103269.