Abstract
Artemisinin, a natural compound from Artemisia annua, is highly effective in treating drug-resistant malaria. Because chemical synthesis of this natural terpenoid is not economically feasible, its only source remains as the native plant which produces only small quantities of it, resulting in a supply that is far short of demand. Extensive efforts have been invested in metabolic engineering for the biosynthesis of artemisinin precursors in microbes. However, the production of artemisinin itself has only been achieved in plants. Since, A. annua possesses only poorly developed genetic resources for traditional breeders, molecular breeding is the best alternative. In this review, we describe the efforts taken to enhance artemisinin production in A. annua via transgenesis and advocate metabolic engineering of the complete functional artemisinin metabolic pathway in heterologous plants. In both cases, we emphasize the need to apply state-of-the-art synthetic biology approaches to ensure successful biosynthesis of the drug.
Introduction
In recent years, there has been a growing demand for artemisinin, a key component of the artemisinin-based combination therapy (ACT) used to treat malaria. Malaria is a disease caused by Plasmodium spp. protozoan parasites; it is a major global epidemic which kills millions, mainly children and pregnant women in sub-Saharan Africa (Chico et al., Citation2012; Marsh, Citation1998; Nayyar, Breman, Newton, & Herrington, Citation2012; World Health Organization [WHO], Citation2005). Artemisinin was identified as the active ingredient in extracts of the Chinese medicinal herb Artemisia annua (commonly known as sweet wormwood or sweet annie) in the 1970s, during research into novel antimalarial drugs. Later, the wide spread of multidrug-resistant malaria led to promotion of the use of ACTs by the World Health Organization (WHO) as the first line of treatment (WHO, Citation2005). A. annua remains the only commercial source of artemisinin, and as is the case for many other secondary metabolites, it is produced in minute amounts of ca. .01–.8% of dry weight (Abdin, Israr, Rehman, & Jain, Citation2003). Furthermore, the dependency of yields on numerous poorly characterized agricultural factors, and the unknown genetic variability of this non-domesticated plant, contributes to a high and unstable cost of artemisinin production and a supply which does not match demand (Van Noorden, Citation2010). In addition to being an effective antimalarial drug, artemisinin has shown promising activity against several types of cancer and other intracellular protozoan parasites of humans and animals (Efferth, Citation2006; Firestone & Sundar, Citation2009; Mazuz et al., Citation2012). Accordingly, there is a call for the development of new artemisinin production platforms and yield improvement by developing fast-track breeding programs, molecular breeding, and synthetic biology systems with the aim of reducing and stabilizing the price of the drug.
Artemisinin is an oxygenated terpene harboring an endoperoxide bridge that is essential for the drug’s effectiveness. A sesquiterpene, its aliphatic backbone amorpha-4,11-diene (amorphadiene) is biosynthesized from farnesyl diphosphate (FDP), which can, in turn, be produced by isopentenyl diphosphate (IPP) arising from the cytosolic mevalonic acid (MVA) pathway or the plastidic 2C-methyl-D-erythritol 4-phosphate (MEP) pathway (Bouvier, Rahier, & Camara, Citation2005). Isotopic labeling and inhibition studies in A. annua have shown that both pathways contribute to building the isoprenoid backbone of artemisinin (Schramek et al., Citation2010; Towler & Weathers, Citation2007). The complete biosynthetic pathway leading from FDP to artemisinin is not fully known. Nevertheless, labeling studies, detailed biochemical analyses, and gene-mining experiments performed in the last decade have revealed that it initiates with the formation of amorphadiene by amorphadiene synthase (ADS), which is then sequentially oxidized by the cytochrome P450 CYP71AV1 and reduced by artemisinic aldehyde reductase (DBR2) (Brown, Citation2010; Zhang et al., Citation2008). The subsequent biochemical steps are not yet clear, but it has been suggested that dihydroartemisinic aldehyde oxidation, catalyzed by aldehyde dehydrogenase 1 (ALDH1), leads to the formation of dihydroartemisinic acid (Figure ) (Covello, Citation2008; Ro et al., Citation2006; Teoh, Polichuk, Reed, & Covello, Citation2006; Teoh, Polichuk, Reed, Nowak, & Covello, Citation2009). Feeding experiments with labeled dihydroartemisinic acid and in vitro examination have demonstrated that this acid is a late-stage artemisinin precursor. Furthermore, there is evidence that in plants, conversion of the acid to artemisinin, via an allylic hydroperoxide intermediate, is nonenzymatic and requires only light and oxygen (Brown, Citation2010).
Figure 1 A schematic summary of metabolic engineering efforts in A. annua. An overview of the MVA pathway (depicted in green) supplying E,E-farnesyl diphosphate (FDP) substrate to the artemisinin pathway (depicted in orange) and to the sterol pathway (depicted in purple) is shown. IPP and dimethylallyl diphosphate (DMAPP) are condensed by FDPS into FDP, which is shunted to artemisinin biosynthesis by amorpha-4,11-diene synthase (ADS), to sterol synthesis by SQS and is also used for other terpenoids such as caryophyllene, which is produced by caryophyllene synthase. Upregulated expression is marked by (↑) and downregulated expression by (↓); the artemisinin-related enzymes are in blue font. Solid arrows, broken arrows, and stippled arrows represent single and multiple enzymatic steps and direct activation, respectively. Abbreviations: HMG, hydroxymethylglutaryl; tHMG, truncated hydroxymethylglutaryl-CoA reductase; CYP, cytochrome P450; CPR, cytochrome P450 reductase; DBR2, artemisinic aldehyde reductase; ALDH1, aldehyde dehydrogenase 1; ERF1 and 2, AP2/ERF-like regulatory factors.
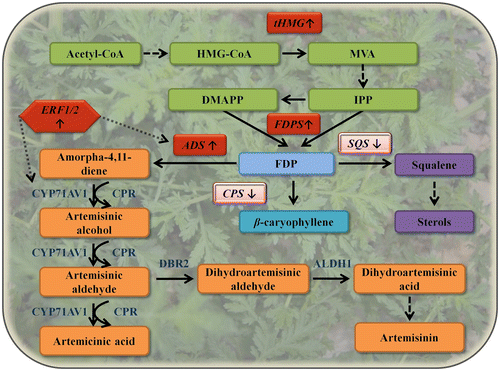
The global drive in recent years to reduce deaths from malaria has led to substantial effort toward improving artemisinin production. A rather large body of research has been geared toward improving agrotechniques and selecting better-performing varieties of A. annua. Nevertheless, since this is not a domesticated species, the prospect of molecular breeding is highly relevant. In parallel, numerous studies have focused on using transgenesis for metabolic engineering toward artemisinin production. In this review, we highlight the recent advances in metabolic engineering for artemisinin production in plants. The remarkable work towards reconstructing the production of artemisinin precursors in microbes led by J. Keasling from the University of California, Berkeley, the aim of which has been to enable the production of feedstock for semi-chemical synthesis of artemisinin in the coming years by Sanofi-Aventis, is beyond the scope of this review. Rather, we will concentrate on studies aimed at improving production yield of A. annua, which still holds considerable as-yet unrealized potential. We will also present recent advances in heterologous plant-based systems for artemisinin production demonstrating the feasibility of fully biosynthesizing artemisinin, and thus opening up numerous possibilities for the use of synthetic biology for the drug’s production.
Homologous metabolic engineering of artemisinin production
One opportunity for improving artemisinin production lies in the classical or molecular breeding of A. annua itself. Indeed, Graham et al. (Citation2010) have utilized genetic variation in A. annua, transcriptome analysis and detailed phenotyping of key traits influencing artemisinin production to define relevant quantitative trait loci. Enhancement of such factors in a hybrid A. annua line have improved production by ∼50% over Artemis, which is a pedigree developed by Mediplant of Switzerland. Although the developed genetic map and molecular markers have paved the way for breeding programs, this solution is limited because of a lack of knowledge on the genetic variability in this non-agronomical species. An alternative way of increasing artemisinin content would be to metabolically modify A. annua via genetic engineering. To this end, transformation of A. annua mediated by Agrobacterium tumefaciens or A. rhizogenes has been reported (Banerjee, Zehra, Gupta, & Kuma, Citation1997; Vergauwe et al., Citation1996). Interestingly, Paniego and Giulietti (Citation1996) have demonstrated that artemisinin can be detected in both shoot and root cultures of A. rhizogenes-transformed A. annua. These findings have demonstrated that artemisinin can be produced in vitro, albeit in low quantities. The ability of hairy root cultures to generate artemisinin in vitro is especially intriguing, since in vivo, it is only produced in specialized tissue – the glandular trichomes (Olsson et al., Citation2009).
The knowledge accumulated on the general terpenoid and specific artemisinin biosynthetic pathways is enabling metabolic engineering in A. annua. Several approaches can be considered to enhance the production of a metabolite such as artemisinin. One option would be to enhance the activity of the artemisinin pathway by overexpressing key genes from this pathway. Another would be to enhance the metabolic flux in the pathway(s) that synthesize the building blocks, i.e. the MVA or MEP pathways, for FDP, the sesquiterpene precursor. In addition, FDP levels might be enhanced by down regulating enzymatic reactions that compete with ADS for its use, such as squalene synthase (SQS) involved in sterol biosynthesis.
Enhancement of metabolic flux in the MVA pathway
In the MVA pathway, 3-hydroxy-3-methylglutaryl-CoA reductase (HMG-R) is considered a main rate-limiting step, although with some debate (Chappell, Wolf, Proulx, Cuellar, & Saunders, Citation1995) regarding plants. When the native form of Catharanthus roseus HMG-R was expressed in A. annua, only a marginal change in artemisinin biosynthesis was observed (Aquil, Husaini, Abdin, & Rather, Citation2009), however when it was coexpressed with ADS, a sevenfold change was measured (Alam & Abdin, Citation2011). These results have suggested that enhanced flux in the MVA pathway itself does not necessarily result in enhanced artemisinin production, probably due to strong utilization of FDP by other pathways. In contrast, expression of cotton farnesyl diphosphate synthase (FDPS) under the control of the CaMV 35S promoter in A. annua led to twofold elevated artemisinin production levels (Chen, Ye, & Li, Citation2000). Interestingly, manipulation of the flux in the MEP pathway, which has been shown to contribute to the artemisinin backbone (Schramek et al., Citation2010; Towler & Weathers, Citation2007) in A. annua, has never been tested.
Repression of pathways competing for artemisinin biosynthesis
Several genes encoding enzymes might possibly compete with the artemisinin pathway for FDP have been isolated from A. annua. To test whether inhibition of sesquiterpene synthases might increase the FDP available for cyclization by ADS, β-caryophyllene synthase was down regulated in transgenic A. annua by RNAi. This led to a decrease in the sesquiterpene production levels and a small increase of artemisinin content in the transgenic lines (Chen et al., Citation2011). One of the major enzymes that utilize FDP in eukaryotes is SQS, which shuttles terpenoids into the sterol pathway. Manipulation of its expression levels with the aim of enhancing sesquiterpenoid production in yeast was shown to be highly effective (Paradise, Kirby, Chan, & Keasling, Citation2008; Ro et al., Citation2006). In A. annua, its suppression was accompanied by reduction in the levels of four major sterols and an increase in artemisinin content (Yang et al., Citation2008; Zhang et al., Citation2009). In both attempts, the authors noticed an effect on the expression of artemisinin-related genes, suggesting intricately interconnected feedback control. It should also be noted that FDP is at a major metabolic branch point and is used for the synthesis of important cellular components such as sterols, ubiquinones, dolichols, and hormones like brassinosteroids and for the modification of proteins (Bouvier et al., Citation2005). Hence, an intrinsic drawback to manipulating factors which utilize this molecule could be a pleiotropic effect on cell metabolism and plant growth (Pulido, Perello, & Rodriguez-Concepcion, Citation2012).
Activation of artemisinin-pathway genes
Perhaps the obvious targets for manipulation in an attempt to elevate artemisinin production in A. annua are the artemisinin pathway-specific genes. However, this was only recently tested, probably because of somewhat low transformation efficiency and long regeneration time in this nonmodel plant. In addition, elevating the activity of a single step might lead to an imbalance in the metabolic pathway and hence to a stress response, due to the accumulation of toxic intermediates. It can also result in a new rate-limiting step and no net change in metabolic flux toward artemisinin production (Anthony et al., Citation2009).
The first attempt to modify the expression of specific genes of the artemisinin pathway was by overexpressing the endogenous ADS which catalyzes the first committed step and diverts FDP into sesquiterpene production (Ma, Wang, et al., Citation2009). This led to some changes in the plant’s metabolic profile – a decrease in the levels of the sesquiterpenes (E)-β-farnesene and germacrene D, and a moderate increase in borneol, phytol, and artemisinin. The only other artemisinin biosynthetic gene tested thus far for its effect on artemisinin production in A. annua is CYP71AV1 (Wang, Han, Kanagarajan, Lundgren, & Brodelius, Citation2013), which encodes a cytochrome P450 oxygenase (Ro et al., Citation2006; Teoh et al., Citation2006). It functions as an amorphadiene 12-hydroxylase and converts the sesquiterpene hydrocarbon to artemisinic alcohol, then artemisinic aldehyde, and finally artemisinic acid. The latter metabolite is now considered to be an off-pathway product of artemisinin biosynthesis (Brown, Citation2010; Zhang et al., Citation2008). Since oxidation by P450 enzymes requires reducing coactivity by a cytochrome P450 reductase (CPR) (Ro et al., Citation2006; Teoh et al., Citation2006), it was coexpressed alongside CYP71AV1 in artemisia. This resulted in only a small elevation in artemisinin production which was not correlated with CYP71AV1 or CPR expression. This might have been due to low and limiting substrate levels, i.e. a different metabolic bottleneck, or by diversion of the pathway toward the dead-end product artemisinic acid. Interestingly, when expressing the entire known artemisinin pathway in a heterologous plant, we observed that CYP71AV1 was not the limiting step in artemisinin biosynthesis (Farhi, Marhevka, Ben-Ari, et al., Citation2011).
Artemisinin production is elevated by hormonal treatment, and it has been shown that at least the promoter of CYP71AV1 is activated in response to methyl jasmonate treatment (Wang et al., Citation2013). Two AP2/ERF subfamily regulatory factors were identified from A. annua and shown to bind to the promoters of ADS and CYP71AV1 in response to jasmonate stimulation. When AaERF1 or AaERF2 were overexpressed in A. annua, artemisinin content was elevated by approximately 50% (Yu et al., Citation2012). An additional defense-related transcription factor, AaWRKY1, was identified as a direct activator of ADS transcription and when transiently expressed in A. annua elevated the expression of other artemisinin-biosynthetic genes (Ma, Pu, et al., Citation2009). However, its influence on artemisinin synthesis was not evaluated.
The above experiments, which are summarized in Figure , imply that levels of available precursors should be elevated in conjunction with specific enhancement of the enzymes dedicated to the artemisinin pathway. In this respect, both multigene transformation (Krichevsky, Zaltsman, King, & Citovsky, Citation2012) and utilization of transcription factors to orchestrate metabolic-pathway activity (Moyal Ben Zvi et al., Citation2008; Spitzer-Rimon et al., Citation2012) are potentially promising avenues to pursue. Furthermore, it should be noted that most of the better achievements in engineering artemisinin production in its native host were in low-accumulating genotypes. This indicates the need, on the one hand, to consider agronomic traits such as leaf area, relation to plant development, trichome number and plant architecture, and on the other, a possible upper limit due to artemisinin toxicity to the plant (Arsenault, Vail, Wobbe, Erickson, & Wethers, Citation2010; Graham et al., Citation2010; Liu, Wang, Du, Li, & Ye, Citation2011).
Non-native plant hosts for the production of artemisinin
In A. annua, artemisinin production is restricted to specialized tissue, i.e. glandular trichomes protruding from the epidermis of leaves and flowers (Olsson et al., Citation2009; Pu, Ma, Wang, Ye, & Liu, Citation2013). Transcripts and enzymes involved in the pathway are expressed in these factories for secondary metabolites, which facilitate their cloning (Covello, Teoh, Polichuk, Reed, & Nowak, Citation2007) but also contribute to the low abundance of artemisinin in its native plant and perhaps to the limited success in engineering its production by a single-gene-modification approach. Since artemisinin is produced only in specialized differentiated tissue, A. annua plant cell cultures are probably not a biotechnological viable solution as in the case of taxol (Roberts & Kolewe, Citation2010; Wilson & Roberts, Citation2012). The use of A. annua has an additional drawback when field-grown for artemisinin harvesting, since this is not a domesticated plant with efficient clonal propagation and agrotechniques that can support high biomass, and artemisinin yields are not well developed. Under current production schemes, it usually takes about a year and a half from sowing artemisia to extracting the artemisinin. Furthermore, despite the fact that genetic variability and chemotypes are hugely diverse in Artemisia, and despite the effort invested in genome-aided breeding (Graham et al., Citation2010), there is still a lack of stable, defined lines available for commercial use. An alternative approach is based on synthetic biology which takes advantage of the known artemisinin pathway and transfers it to a suitably amenable plant host that can support high artemisinin and biomass yield while still relying on the economic advantage of photosynthesis as the energy source.
Engineering production of artemisinin precursors
Early on, Wallaart, Bouwmeester, Hille, Poppinga, and Maijers (Citation2001), who functionally characterized ADS as the sesquiterpene synthase responsible for synthesizing the terpenoid backbone of artemisinin, transformed it into Nicotiana tabacum using the constitutive cauliflower mosaic virus 35S promoter. Similar to other attempts to engineer sesquiterpene production in plants (Wu et al., Citation2006); only low amounts [ca. 1 ng/g fresh weight (FW)] of amorphadiene have been detected (Wallaart et al., Citation2001). It seemed that the production of cytosolic sesquiterpenes is limited by available pools of FDP and/or reflect tight regulation over terpenoid metabolism. Furthermore, several experiments have demonstrated that the terpenoid backbone of sesquiterpenes, including that of amorphadiene in A. annua, can be derived from the plastidic MEP pathway despite the fact that the sesquiterpene synthases are localized in the cytosol and FDP is not known to be generated in plastids (Dudareva et al., Citation2005; Schramek et al., Citation2010).
Two breakthroughs in engineering terpene metabolism were achieved by relying on other cellular compartments as sites of production. Previous work demonstrated that targeting a strawberry linalool/nerolidol synthase to the mitochondria of Arabidopsis thaliana enables efficient production of (3S)-(E)-nerolidol, although actual levels and comparison to cytosolic production were not indicated (Kappers, Aharoni, & van Herpen, Citation2005). Interestingly, we found that engineering Saccharomyces cerevisiae with a mitochondrial-targeted ADS allowed for much higher production levels of amorphadiene when compared to cytosolic engineering (Farhi, Marhevka, Masci, et al., Citation2011). The intriguing importance of compartmentalization for terpene engineering was demonstrated when a ca. 10,000-fold elevation in amorphadiene synthesis was achieved after coexpressing ADS and an avian FDPS, both targeted to chloroplasts of N. tabacum, as compared to sole expression of the native cytosolic form of ADS (Wu et al., Citation2006). Interestingly, the same work demonstrated that the accumulated levels of another sesquiterpene, patchoulol, metabolically engineered by targeting patchoulol synthase and FDPS to the plastids, were comparable to those produced by targeting both enzymes to the cytosol and adding a truncated and deregulated HMG-R (tHMG) from hamster. This suggests that despite the advantage of harnessing the plastids, similar production levels can be achieved by using tHMG and FDPS. Coproduction of terpenoids via the cytosol and plastid has not been reported.
Considering the need to activate a precursor supply for artemisinin biosynthesis and taking advantage of other than the cytosol compartment for terpenoid production, van Herpen et al. (Citation2010) attempted to produce the artemisinin-related metabolite artemisinic acid in Nicotiana benthamiana. Employing a transient expression system based on agroinfiltration, they demonstrated that amorphadiene can be produced at levels of ca. 6 μg/g FW by targeting ADS to the mitochondria (mtADS) and coexpressing a mitochondrial-targeted FDPS (mtFDPS, based on ERG20 from yeast) and tHMG cloned from Arabidopsis (van Herpen et al., Citation2010). The coexpression system employed an artificial polycistronic-like multigene construct in which the coding sequences were separated by the 2A ribosomal skipping sequence. When evaluating the separate contribution of mitochondrial FDPS and tHMG, the authors concluded that only the latter makes a strong contribution to amorphadiene biosynthesis in the mitochondria. Moreover, in yeast metabolic engineering of sesquiterpene production, expression of a cytosolic tHMG contributed more than that of a cytosolic FDPS to enhancement in terpenoid production levels by a mtADS (Farhi, Marhevka, Masci, et al., Citation2011). When CYP71AV1 was coinfiltrated into tobacco with the multigene construct, none of the artemisinin-related molecules – artemisinic alcohol, artemisinic aldehyde, or artemisinic acid – were detected. Metabolic analysis revealed that artemisinic acid accumulates as a diglucoside. This demonstrates the obstacles placed by native metabolism on metabolic engineering and the need to identify a suitable host for specific applications of synthetic biology (Lubertozzi & Keasling, Citation2008). On a more positive note, since mtADS was targeted to the mitochondria, while CYP71AV1, being a cytochrome P450, was presumably anchored to the ER, these results suggest that a terpenoid olefin backbone synthesized in one subcellular compartment may still be accessible to cytosolic modification by other enzymes.
Successful production of amorphadiene in N. tabacum and identification of the genes encoding the subsequent enzymes in the artemisinin biosynthetic pathway facilitated Zhang, Nowak, Reed, and Covello’s (Citation2011) attempt to generate the late pathway molecules in plants. They used a multigene construct for the stable transformation of tobacco and expressed A. annua ADS and FPS genes fused with a plastidial targeting sequence and under the control of 35S promoters and Nos terminators (Zhang et al., Citation2011). This allowed for amorphadiene production at an intermediate level of ca. .25 μg/g FW. Addition of CYP71AV1 using a similar vector led to an average reduction in the hydrocarbon’s level and detection of artemisinic alcohol. Interestingly, neither of the expected successive metabolites – artemisinic aldehyde and artemisinic acid – which are produced by the action of CYP71AV1 in vitro and in yeast, could be detected (Zhang et al., Citation2008). As with mitochondria localized production of amorphadiene, the hydrocarbon sesquiterpene synthesized by the plastid-localized ADS, was still accessible to the cytosolic CYP71AV1. This is similar to the case of native cytochrome P450-dependent modifications of terpenoids produced in plastids (Croteau, Davis, Ringer, & Wildung, Citation2005).
In other tobacco lines generated with a construct in which DBR2 was added to CYP71AV1, the plastid-targeted FDPS and ADS, in addition to amorphadiene and artemisinic alcohol, small amounts of molecules with a reduction in the Δ11(13) double bond were detected, but again only dihydroartemisinic alcohol was detected and not the more oxidized successive pathway compounds, i.e. the aldehyde or acid forms. Speculating that CYP71AV1 activity did lead to the formation of aldehydes in the transgenic tobacco but endogenous reducing activity prevented their accumulation, the authors attempted to enhance flux toward dihydroartemisinic acid by adding ALDH1 to the expression cassette and generating new transgenes. However, this did not lead to the accumulation of amorphane acids or artemisinin, but for some reason elevated the total yield of amorphane sesquiterpenes from ∼.25 to .75 μg/g FW. It seems that some native reducing action prevented the successive oxidizing metabolism of the alcohols in the transgenic tobacco, or that in these experiments, the oxidation activity of the cytochrome P450 was not high enough to drive the formation of the aldehydes and acids (Zhang et al., Citation2011). It should be noted that general eukaryotic ER-bound cytochrome P450 enzymes require an interaction with CPR and available NADPH (which acts as cosubstrate). For example, routine functional characterization of cytochrome P450 enzymes using microsomes is performed in lines expressing or overexpressing a suitable reductase, e.g. the S. cerevisiae WAT11 strain expressing the Arabidopsis reductase ATR1 (Rontein et al., Citation2008; Urban, Mignotte, Kazmaier, Delorme, & Pompon, Citation1997).
Metabolic engineering of artemisinin production
Sustainable and potentially low-cost heterologous production of artemisinin would rely on obtaining the final compound rather than precursors, as sub-extractions and chemical reaction steps would require additional resources and time. While recent advances in flow chemistry may lead to the development of semi-synthetic production of artemisinin, they still depend on biosynthesized artemisinic acid feedstock (Kupferschmidt, Citation2012). Unfortunately, A. annua chemotypes with high levels of artemisinic acid are low producers of artemisinin and vice versa (Zhang et al., Citation2008).
We were recently able to successfully demonstrate that production of artemisinin is feasible in a heterologous host by combining several metabolic engineering approaches into one strategy (Farhi, Marhevka, Ben-Ari, et al., Citation2011). This advance was based on the suggestion that dihydroartemisinic acid can be converted to artemisinin without any specialized metabolic machinery and hence engineering its accumulation in transgenic tobacco may lead to the active drug’s production. To ensure expression of the artemisinin-pathway genes in a single cell and circumvent the potential genetic instability associated with large multigene constructs and repetitive sequences, all of these were combined into a single transformation vector, with each being under the control of separate and different promoters and terminators. Given that a positive effect of elevating metabolic flux in the MVA pathway via expression of tHMG has been demonstrated on artemisinin and terpenoid production (Liu et al., Citation2011), a bona fide hyperactive tHMG-encoding sequence from yeast was also cloned into the transformation vector. In addition to the artemisinin pathway-specific genes ADS, CYP71AV1, and DBR2, the A. annua-specific CPR was added to avoid low activity of the cytochrome P450 due to its insufficient reduction. The combined expression of these genes in yeast was shown to enable production of dihydroartemisinic acid (Zhang et al., Citation2008). To take advantage of the potentially efficient sesquiterpene production in the mitochondria, ADS gene product was targeted to this organelle. The other transgene products, with the exception of tHMG which forms a soluble and non-ER-anchored form of HMG-R, were in their native ER-bound forms. Several transgenic N. tabacum lines generated with such a multigene construct accumulated artemisinin at ca. 7 μg/g dry weight, thereby demonstrating the potential for metabolic engineering of artemisinin production outside the context of the glandular trichomes of A. annua. The levels of amorphadiene accumulated in our tobacco lines and those reported by Zhang et al. (Citation2011) were comparable and do not seem to be a limiting factor for artemisinin biosynthesis. However, unlike the latter study, our engineering scheme employed coexpression of CPR to support the oxidizing activity of CYP71AV1 (the native copartner from A. annua); this might have made a major difference because lack of oxidation to acids in Zhang et al.’s (Citation2011) study prevented accumulation of dihydroartemisinic acid.
To this end, tobacco is the only plant, apart from A. annua, in which artemisinin production has been demonstrated; this opens up new opportunities for molecular farming of artemisinin in plants with better biomass generation and established fast-growth practices than artemisia. Tobacco itself is a good production platform since there is ample knowledge on its genetics and growing practices to allow for rapid and high biomass yields. Furthermore, tobacco can be efficiently grown in areas such as China and East Africa where A. annua cultivation and extraction facilities are already operational. Other engineered plants may be capable of accumulating higher levels of artemisinin. For example, an interesting collaboration between Dafra Pharma International NV and Plant Research International of the University of Wageningen relies on the observation that Cichorium intybus (chicory) produces large amounts of sesquiterpene lactones, similar to artemisinin. The assumption is that this host may serve as an efficient platform for dihydroartemisinic acid production due to its biochemical properties, which are similar to those of A. annua (http://www.dafra.be/content/chicory-project). The outcome of this initiative should be quite interesting.
We propose that artemisinin production in metabolically engineered plants can be boosted by further modifications to the flux of the FDP precursor’s supply to elevate amorphadiene accumulation and by combining several subcellular compartments for production. For example, flux through the MVA pathway toward FDP synthesis can be increased by expressing more efficient variants of the eukaryotic pathway (Wang et al., Citation2012) or ‘de-bottlenecking’ pathway steps such as FDPS which, aside from HMG-R, seems to be another major rate-limiting factor in the MVA pathway (Keasling, Citation2010; Wu et al., Citation2006). FDP accumulation can also be enhanced by reducing the expression of the enzymes that metabolize it, e.g. SQS; the reduction should be performed in a spatial and temporal manner (by use of specific promoters) to prevent growth retardation. Since it has been shown that compartmentalizing terpenoid biosynthesis to the mitochondria and the chloroplasts enhances production, precursor synthesis can also be specifically elevated in lines engineered for artemisinin production, for example by targeting FDPS to these compartments. In this respect, multigene transformation of the chloroplast genome is proving to be a useful tool, as recently demonstrated in transplastomic plants with the MVA pathway (Kumar et al., Citation2012). It would also be interesting to evaluate whether levels of cofactors, such as NADPH, are limiting for artemisinin production, which can now be manipulated in the heterologous host.
One of the advantages of artemisinin production from tissue other than trichomes is that cell-suspension cultures can be generated for production in plant bioreactors (Wilson & Roberts, Citation2012). Interestingly, we recently observed that milligram per liter quantities of amorphadiene can be produced in a plant cell culture (unpublished data). Cell-suspension culture would be highly suited for rapid testing of the different factors that affect artemisinin production and for metabolic flux analysis in the artemisinin pathway. For example, the effect of manipulating the MVA or MEP pathways could be easily assessed using specific inhibitors such as mevinolin and fosmidomycin, or a reduction in the flux toward phytosterol synthesis could be tested using squalestatin (Pulido et al., Citation2012). Furthermore, cell-suspension culture could be used to evaluate whether conversion of dihydroartemisinic acid (or aldehyde) is spontaneous or catalyzed by internal enzymes by examining the precursor–product relationships when applying different light and oxygen levels or by performing feeding experiments. Labeling and flux-analysis measurements could also help decipher the metabolic network and main intermediate molecules leading to artemisinin production. It would also be interesting to evaluate whether levels of cofactors such as NADPH are limiting for artemisinin production.
Conclusion
The identification of genes encoding the enzymes involved in the artemisinin-biosynthesis pathway along with recent advances in metabolic engineering of plant terpenoids is providing new opportunities for engineering artemisinin biosynthesis. Molecular breeding of A. annua can generate improved lines, potentially via balanced multigene transformation of the relevant pathway genes. However, at this point, such efforts are hampered by the complexity of the transformation and a lack of knowledge about the amorphanes metabolic network controlling artemisinin accumulation in the native plant.
Heterologous production of artemisinin in an alternative plant is now feasible and might enable the development of a designer production platform based on plants with high yields and biomass production rooted in defined agricultural practice. Utilization of multigene transformation and transplastomics, harnessing several cellular compartments and cofactors, will enable elevating production in future engineered lines. Especially, exciting is the prospect of generating cell cultures and the use of in situ product removal for continuous production in bioreactors, which would eliminate the potential toxicity of artemisinin to cells. Figure summarizes some of the metabolic engineering approaches which have enabled artemisinin production in a heterologous plant and that may facilitate future enhancement in production levels. Taken together, we expect that these advances in the metabolic engineering of artemisinin production will help lower and stabilize the drug’s production costs and make it more accessible to malaria sufferers in developing countries. Furthermore, a reduction in artemisinin’s production costs may facilitate its use in the treatment of other apicomplexan-borne diseases, as well in cancer therapy.
Figure 2 Illustration of the advances in artemisinin production in heterologous plants. The use of multigene mega-vectors and simultaneous harnessing of different subcellular compartments for expression of the pathway genes aimed at engineering drug production in whole plants or cell cultures is portrayed. mtADS and mtFDPS represent mitochondrial-localized amorpha-4,11-diene and FDPSs, respectively. chADS and chFDPS represent plastid-localized amorpha-4,11-diene and FDPSs, respectively. Also shown are artemisinin building blocks originating from acetyl-CoA through the MVA pathway or from pyruvate and glyceraldehyde 3-phosphate (G3P) through the plastid-localized MEP pathway. Solid arrows, broken arrows, and stippled arrows represent single and multiple enzymatic steps, and transport, respectively. Abbreviations: tHMG, truncated hydroxymethylglutaryl-CoA reductase; CYP, cytochrome P450; CPR, cytochrome P450 reductase; DBR2, artemisinic aldehyde reductase; IPP, isopentenyl diphosphate; DMAPP, dimethylallyl diphosphate; FDP, E,E-farnesyl diphosphate.
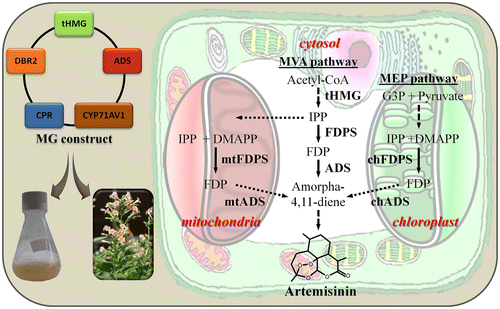
Acknowledgments
We thank I. Kaye for his support and assistance. This work was partially funded by the Israel Science Foundation grant no. 432/10, F.I.R.S.T. 432/10 and BARD grant no. US-4322-10. A.V. is an incumbent of the Wolfson Chair in Floriculture.
References
- Abdin , M. Z. , Israr , M. , Rehman , R. U. and Jain , S. K. 2003 . Artemisinin, a novel antimalarial drug: Biochemical and molecular approaches for enhanced production . Planta Medica , 69 : 289 – 299 .
- Alam , P. and Abdin , M. Z. 2011 . Over-expression of HMG-CoA reductase and amorpha-4,11-diene synthase genes in Artemisia annua L. and its influence on artemisinin content . Plant Cell Reports , 30 : 1919 – 1928 .
- Anthony , J. R. , Anthony , L. C. , Nowroozi , F. , Know , G. , Newman , J. D. and Keasling , J. D. 2009 . Optimization of the mevalonate-based isoprenoid biosynthetic pathway in Escherichia coli for production of the anti-malarial drug precursor amorpha-4,11-diene . Metabolic Engineering , 11 : 13 – 19 .
- Aquil , S. , Husaini , A. M. , Abdin , M. Z. and Rather , G. M. 2009 . Overexpression of the HMG-CoA reductase gene leads to enhanced artemisinin biosynthesis in transgenic Artemisia annua plants . Planta Medica , 75 : 1453 – 1458 .
- Arsenault , P. R. , Vail , D. , Wobbe , K. K. , Erickson , K. and Wethers , P. J. 2010 . Reproductive development modulates gene expression and metabolite levels with possible feedback inhibition of artemisinin in Artemisia annua . Plant Physiology , 154 : 958 – 968 .
- Banerjee , S. , Zehra , M. , Gupta , M. M. and Kuma , S. 1997 . Agrobacterium rhizogenes mediated transformation of Artemisia annua: Production of transgenic plants . Planta Medica , 63 : 467 – 469 .
- Bouvier , F. , Rahier , A. and Camara , B. 2005 . Biogenesis, molecular regulation and function of plant isoprenoids . Progress in Lipid Research , 44 : 357 – 429 .
- Brown , G. D. 2010 . The biosynthesis of artemisinin (Qinghaosu) and the phytochemistry of Artemisia annua L. (Qinghao) . Molecules , 15 : 7603 – 7698 .
- Chappell , J. , Wolf , F. , Proulx , J. , Cuellar , R. and Saunders , C. 1995 . Is the reaction catalyzed by 3-hydroxy-3-methylglutaryl coenzyme A reductase a rate-limiting step for isoprenoid biosynthesis in plants? . Plant Physiology , 109 : 1337 – 1343 .
- Chen , J.-L. , Fang , H.-M. , Ji , Y.-P. , Pu , G.-B. , Guo , Y.-W. , Huang , L.-L. , … and Wang , H. 2011 . Artemisinin biosynthesis enhancement in transgenic Artemisia annua plants by downregulation of the β-caryophyllene synthase gene . Planta Medica , 77 : 1759 – 1765 .
- Chen , D. H. , Ye , H. C. and Li , G. F. 2000 . Expression of a chimeric farnesyl diphosphate synthase gene in Artemisia annua L. transgenic plants via Agrobacterium tumefaciens-mediated transformation . Plant Science , 155 : 179 – 185 .
- Chico , R. M. , Mayaud , P. , Ariti , C. , Mabey , D. , Ronsmans , C. and Chandramohan , D. 2012 . Prevalence of malaria and sexually transmitted and reproductive tract infections in pregnancy in sub-Saharan Africa: A systematic review . Journal of the American Medical Association , 307 : 2079 – 2086 .
- Covello , P. S. 2008 . Making artemisinin . Phytochemistry , 69 : 2881 – 2885 .
- Covello , P. S. , Teoh , K. H. , Polichuk , D. R. , Reed , D. W. and Nowak , G. 2007 . Functional genomics and the biosynthesis of artemisinin . Phytochemistry , 68 : 1864 – 1871 .
- Croteau , R. , Davis , E. , Ringer , K. and Wildung , M. 2005 . (−)-Menthol biosynthesis and molecular genetics . Naturwissenschaften , 92 : 562 – 577 .
- Dudareva , N. , Andersson , S. , Orlova , I. , Gatto , N. , Reichelt , M. , Rhodes , D. , … and Gershenzon , J. 2005 . The nonmevalonate pathway supports both monoterpene and sesquiterpene formation in snapdragon flowers . Proceedings of the National Academy of Sciences of the United States of America , 102 : 933 – 938 .
- Efferth , T. 2006 . Molecular pharmacology and pharmacogenomics of artemisinin and its derivatives in cancer cells . Current Drug Targets , 7 : 407 – 421 .
- Farhi , M. , Marhevka , E. , Ben-Ari , J. , Algamas-Dimantov , A. , Liang , Z. , Zeevi , V. , … and Vainstein , A. 2011 . Generation of the potent anti-malarial drug artemisinin in tobacco . Nature Biotechnology , 29 : 1072 – 1074 .
- Farhi , M. , Marhevka , E. , Masci , T. , Marcos , E. , Eyal , Y. , Ovadis , M. , … and Vainstein , A. 2011 . Harnessing yeast subcellular compartments for the production of plant terpenoids . Metabolic Engineering , 13 : 474 – 481 .
- Firestone , G. L. and Sundar , S. N. 2009 . Anticancer activities of artemisinin and its bioactive derivatives . Expert Reviews in Molecular Medicine , 11 : e32
- Graham , I. A. , Besser , K. , Blumer , S. , Branigan , C. A. , Czechowski , T. , Elias , L. , … and Bowles , D. 2010 . The genetic map of Artemisia annua L. identifies loci affecting yield of the antimalarial drug artemisinin . Science , 327 : 328 – 331 .
- Kappers , I. F. , Aharoni , A. and van Herpen , T. W. J. M. 2005 . Genetic engineering of terpenoid metabolism attracts bodyguards to Arabidopsis . Science , 309 : 2070 – 2072 .
- Keasling , J. D. 2010 . Manufacturing molecules through metabolic engineering . Science , 330 : 1355 – 1358 .
- Krichevsky , A. , Zaltsman , A. , King , L. and Citovsky , V. 2012 . Expression of complete metabolic pathways in transgenic plants . Biotechnology and Genetic Engineering Reviews , 28 : 1 – 13 .
- Kumar , S. , Hahn , F. M. , Baidoo , E. , Kahlon , T. S. , Wood , D. F. , McMahan , C. M. , … and Whalen , M. C. 2012 . Remodeling the isoprenoid pathway in tobacco by expressing the cytoplasmic mevalonate pathway in chloroplasts . Metabolic Engineering , 14 : 19 – 28 .
- Kupferschmidt , K. 2012 . Can new chemistry make a malaria drug plentiful and cheap? . Science , 336 : 798 – 799 .
- Liu , B. , Wang , H. , Du , Z. , Li , G. and Ye , H. 2011 . Metabolic engineering of artemisinin biosynthesis in Artemisia annua L . Plant Cell Reports , 30 : 689 – 694 .
- Lubertozzi , D. and Keasling , J. 2008 . Expression of a synthetic Artemisia annua amorphadiene synthase in Aspergillus nidulans yields altered product distribution . Journal of Industrial Microbiology & Biotechnology , 35 : 1191 – 1198 .
- Ma , D. , Pu , G. , Lei , C. , Ma , L. , Wang , H. , Guo , Y. , … and Liu , B. 2009 . Isolation and characterization of AaWRKY1, an Artemisia annua transcription factor that regulates the amorpha-4,11-diene synthase gene, a key gene of artemisinin biosynthesis . Plant and Cell Physiology , 50 : 2146 – 2161 .
- Ma , C. , Wang , H. , Lu , X. , Wang , H. , Xu , G. and Liu , B. 2009 . Terpenoid metabolic profiling analysis of transgenic Artemisia annua L. by comprehensive two-dimensional gas chromatography time-of-flight mass spectrometry . Metabolomics , 5 : 497 – 506 .
- Marsh , K. 1998 . Malaria disaster in Africa . Lancet , 352 : 924 – 924 .
- Mazuz , M. L. , Haynes , R. , Shkap , V. , Fish , L. , Wollkomirsky , R. , Leibovich , B. , Molad , T. , Savitsky , I. and Golenser , J. 2012 . Neospora caninum: In vivo and in vitro treatment with artemisone . Veterinary Parasitology , 187 : 99 – 104 .
- Moyal Ben Zvi , M. , Negre-Zakharov , F. , Masci , T. , Ovadis , M. , Shklarman , E. , Ben-Meir , H. , … and Vainstein , A. 2008 . Interlinking showy traits: Co-engineering of scent and colour biosynthesis in flowers . Plant Biotechnology Journal , 6 : 403 – 415 .
- Nayyar , G. M. L. , Breman , J. G. , Newton , P. N. and Herrington , J. 2012 . Poor-quality antimalarial drugs in Southeast Asia and sub-Saharan Africa . The Lancet Infectious Diseases , 12 : 488 – 496 .
- Olsson , M. E. , Olofsson , L. M. , Lindahl , A.-L. , Lundgren , A. , Brodelius , M. and Brodelius , P. E. 2009 . Localization of enzymes of artemisinin biosynthesis to the apical cells of glandular secretory trichomes of Artemisia annua L . Phytochemistry , 70 : 1123 – 1128 .
- Paniego , N. B. and Giulietti , A. M. 1996 . Artemisinin production by Artemisia annua L-transformed organ cultures . Enzyme and Microbial Technology , 18 : 526 – 530 .
- Paradise , E. M. , Kirby , J. , Chan , R. and Keasling , J. D. 2008 . Redirection of flux through the FPP branch-point in Saccharomyces cerevisiae by down-regulating squalene synthase . Biotechnology and Bioengineering , 100 : 371 – 378 .
- Pu, G.-B., Ma, D.-M., Wang, H., Ye, H.-C., & Liu, B.-Y. (2013). Expression and localization of amorpha-4,11-diene synthase in Artemisia annua L. Plant Molecular Biology Reporter, 31, 32–37.
- Pulido , P. , Perello , C. and Rodriguez-Concepcion , M. 2012 . New insights into plant isoprenoid metabolism . Molecular Plant , 5 : 964 – 967 .
- Ro , D.-K. , Paradise , E. M. , Ouellet , M. , Fisher , K. J. , Newman , K. L. , Ndungu , J. M. , … and Keasling , J. D. 2006 . Production of the antimalarial drug precursor artemisinic acid in engineered yeast . Nature , 440 : 940 – 943 .
- Roberts , S. and Kolewe , M. 2010 . Plant natural products from cultured multipotent cells . Nature Biotechnology , 28 : 1175 – 1176 .
- Rontein , D. , Onillon , S. , Herbette , G. , Lesot , A. , Werck-Reichhart , D. , Sallaud , C. and Tissier , A. 2008 . CYP725A4 from yew catalyzes complex structural rearrangement of taxa-4(5),11(12)-diene into the cyclic ether 5(12)-oxa-3(11)-cyclotaxane . Journal of Biological Chemistry , 283 : 6067 – 6075 .
- Schramek , N. , Wang , H. , Römisch-Margl , W. , Keil , B. , Radykewicz , T. , Winzenhörlein , B. , … and Eisenreich , W. 2010 . Artemisinin biosynthesis in growing plants of Artemisia annua. A 13CO2 study . Phytochemistry , 71 : 179 – 187 .
- Spitzer-Rimon, B., Farhi, M., Albo, B., Cna’ani, A., Moyal Ben-Zvi, M., Masci, T., … Vainstein, A. (2012). EOBI, acting downstream of EOBII, regulates scent production by activating ODO1 and structural scent-related genes. The Plant Cell, 24, 5089–5105.
- Teoh , K. H. , Polichuk , D. R. , Reed , D. W. and Covello , P. S. 2006 . Artemisia annua L. (Asteraceae) trichome-specific cDNAs reveal CYP71AV1, a cytochrome P450 with a key role in the biosynthesis of the antimalarial sesquiterpene lactone artemisinin . FEBS Letters , 580 : 1411 – 1416 .
- Teoh , K. H. , Polichuk , D. R. , Reed , D. W. , Nowak , G. and Covello , P. S. 2009 . Molecular cloning of an aldehyde dehydrogenase implicated in artemisinin biosynthesis in Artemisia annua . Botany , 87 : 635 – 642 .
- Towler , M. and Weathers , P. 2007 . Evidence of artemisinin production from IPP stemming from both the mevalonate and the nonmevalonate pathways . Plant Cell Reports , 26 : 2129 – 2136 .
- Urban , P. , Mignotte , C. , Kazmaier , M. , Delorme , F. and Pompon , D. 1997 . Cloning, yeast expression, and characterization of the coupling of two distantly related Arabidopsis thaliana NADPH-cytochrome P450 reductases with P450 CYP73A5 . Journal of Biological Chemistry , 272 : 19176 – 19186 .
- Van Herpen , T. W. J. M. , Cankar , K. , Nogueira , M. , Bosch , D. , Bouwmeester , H. J. and Beekwilder , J. 2010 . Nicotiana benthamiana as a production platform for artemisinin precursors . PLoS ONE , 5 : e14222
- Van Noorden , R. 2010 . Demand for malaria drug soars . Nature , 466 : 672 – 673 .
- Vergauwe , A. , Cammaert , R. , Vandenberghe , D. , Genetello , C. , Inze , D. , VanMontagu , M. and VandenEeckhout , E. 1996 . Agrobacterium tumefaciens-mediated transformation of Artemisia annua L. and regeneration of transgenic plants . Plant Cell Reports , 15 : 929 – 933 .
- Wallaart , T. E. , Bouwmeester , H. J. , Hille , J. , Poppinga , L. and Maijers , N. C. A. 2001 . Amorpha-4,11-diene synthase: Cloning and functional expression of a key enzyme in the biosynthetic pathway of the novel antimalarial drug artemisinin . Planta , 212 : 460 – 465 .
- Wang, H., Han, J., Kanagarajan., S., Lundgren, A., & Brodelius, P. E. (2013). Trichome-specific expression of the amorpha-4,11-diene 12-hydroxylase (cyp71av1) gene, encoding a key enzyme of artemisinin biosynthesis in Artemisia annua, as reported by a promoter–GUS fusion. Plant Molecular Biology, 81, 119–138.
- Wang , H. , Nagegowda , D. A. , Rawat , R. , Bouvier-Navé , P. , Guo , D. , Bach , T. J. and Chye , M.-L. 2012 . Overexpression of Brassica juncea wild-type and mutant HMG-CoA synthase 1 in Arabidopsis up-regulates genes in sterol biosynthesis and enhances sterol production and stress tolerance . Plant Biotechnology Journal , 10 : 31 – 42 .
- WHO . 2005 . World Malaria Report , Geneva : World Health Organization .
- Wilson , S. A. and Roberts , S. C. 2012 . Recent advances towards development and commercialization of plant cell culture processes for the synthesis of biomolecules . Plant Biotechnology Journal , 10 : 249 – 268 .
- Wu , S. , Schalk , M. , Clark , A. , Miles , R. B. , Coates , R. and Chappell , J. 2006 . Redirection of cytosolic or plastidic isoprenoid precursors elevates terpene production in plants . Nature Biotechnology , 24 : 1441 – 1447 .
- Yang , R.-Y. , Feng , L.-L. , Yang , X.-Q. , Yin , L.-L. , Xu , X.-L. and Zeng , Q.-P. 2008 . Quantitative transcript profiling reveals down-regulation of a sterol pathway relevant gene and overexpression of artemisinin biogenetic genes in transgenic Artemisia annua plants . Planta Medica , 74 : 1510 – 1516 .
- Yu , Z.-X. , Li , J.-X. , Yang , C.-Q. , Hu , W.-L. , Wang , L.-J. and Chen , X.-Y. 2012 . The jasmonate-responsive AP2/ERF transcription factors AaERF1 and AaERF2 positively regulate artemisinin biosynthesis in Artemisia annua L . Molecular Plant , 5 : 353 – 365 .
- Zhang , L. , Jing , F. , Li , F. , Li , M. , Wang , Y. , Wang , G. , … and Tang , K. 2009 . Development of transgenic Artemisia annua (Chinese wormwood) plants with an enhanced content of artemisinin, an effective anti-malarial drug, by hairpin-RNA-mediated gene silencing . Biotechnology and Applied Biochemistry , 52 : 199 – 207 .
- Zhang , Y. , Nowak , G. , Reed , D. W. and Covello , P. S. 2011 . The production of artemisinin precursors in tobacco . Plant Biotechnology Journal , 9 : 445 – 454 .
- Zhang , Y. , Teoh , K. H. , Reed , D. W. , Maes , L. , Goossens , A. , Olson , D. J. H. , … and Covello , P. S. 2008 . The molecular cloning of artemisinic aldehyde Delta 11(13) reductase and its role in glandular trichome-dependent biosynthesis of artemisinin in Artemisia annua . Journal of Biological Chemistry , 283 : 21501 – 21508 .