Abstract
Purpose: To determine the effect of background tissue thermal conductivity on RF ablation heating using ex vivo agar phantoms and computer modelling.
Method: Two-compartment cylindrical agar phantom models (5% agar, 5% NaCl, 3% sucrose) were constructed. These included a standardized inner compartment (2 cm diameter, 4 cm length, 0.25% agar) representing a tumour, surrounded by an outer compartment representing background tissue. The thermal conductivity of the outer compartment was varied from 0.48 W m−1°C (normal liver) to 0.23 W m−1°C (fat) by adding a fat-saturated oil-based solute (10–90%) to the agar. RF ablation was applied at 2000 mA current for 2 min. Temperatures were recorded up to 4 cm from the electrode tip at 1 cm intervals. Subsequently, a 2-D finite element computer model was used to simulate RF ablation of 2–24 min duration for tumours measuring 2–4 cm in diameter surrounded by tissues of different thermal conductivity with the presence or absence of perfusion (0–5 kg m−3 s−1) (n = 44). A comparison of results was performed.
Results: In agar phantoms, the amount of fat in the background tissue correlated with thermal conductivity as a negative exponential function (r2 = 0.98). Significantly increased temperatures were observed at the edge of the inner compartment (1 cm from the electrode tip) as the fat content of the outer compartment increased (p < 0.01). Thus, temperatures at 2 min measured 31.5 ± 2.2°C vs 45.1 ± 3.1°C for thermal conductivities of 0.46 W m−1°C (10% fat) and 0.23 W m−1°C (90% fat), respectively. On the other hand, higher levels of fat led to lower temperature increases in the background compartment (0.2 ± 0.3°C for 90% fat vs. 1.1 ± 0.05°C for 10% fat, p < 0.05). Phantom thermal heating patterns correlated extremely well with computer modelling (r2 = 0.93), demonstrating that background tissues with low thermal conductivity increase heating within the central tumour, particularly for longer durations of RF ablation and in smaller tumours. Furthermore, computer modelling demonstrated that increases in temperature at the tumour margin for background tissues of lower thermal conductivity persisted in the presence of perfusion, with a clinically relevant 4.5°C difference between background thermal conductivities of fat and soft tissue for a 3 cm tumour with perfusion of 2 kg m−3 s−1, treated for 12 min.
Conclusion: Lower thermal conductivity of background tissues significantly increases temperatures within a defined ablation target. These findings provide insight into the ‘oven effect’ (i.e. increased heating efficacy for tumours surrounded by cirrhotic liver or fat) and highlight the importance of both the tumour and the surrounding tissue characteristics when contemplating RF ablation efficacy.
Introduction
While RF has served as an effective therapeutic alternative to standard surgical treatment for focal liver neoplasms Citation[1–3], an increasing number of investigators are extending its use to other sites including the kidney Citation[4–6], lung Citation[7], Citation[8], bone Citation[9], Citation[10], adrenal glands Citation[11], Citation[12] and breast Citation[13]. Due to different tissue characteristics, such as blood flow and electrical and thermal conductivity Citation[14], the effect of surrounding tissue needs to be considered while performing RF ablation on these various tissues, if predictable ablation is to be achieved. However, insufficient fundamental knowledge about tissue–energy interactions and incomplete system optimization and characterization has resulted in the clinical reality of investigators being unable to consistently achieve the desired goal of complete and/or predictable ablation. As such, even with multiple treatment sessions, depending on the tumour type and organ, complete ablation is only achieved in 60–90% of tumours 3–5 cm in diameter Citation[15–17].
Recently, Ahmed et al. Citation[18] have substantiated the importance of not only the tissue characteristics of the tumours but also the background tumour environment by demonstrating significant differences in tumour destruction when performing RF ablation in the same tumour type in the kidney, lung and breast, using the canine venereal sarcoma (CVS) model. An earlier observation about tissue effects concerned thermal conductivity, as Livraghi et al. Citation[19] described an ‘oven effect’ based upon a clinical observation in which RF induced necrosis conformed to the size and shape of the tumour. They hypothesized that the fibrous cirrhotic liver functions as a thermal insulator that concentrates heating in the tumour tissue. Lobo et al. Citation[20] have also shown the importance of thermal conductivity in altering RF outcome for a uniform tissue with computer modelling. Based on these findings, it was hypothesized that RF-induced heating is directly correlated with background tissue thermal conductivity. However, the extent of the effect of the thermal conductivity on RF efficacy is unknown. Thus, the purpose of this study was to quantify and establish a relationship between the effects of background tissue thermal conductivity and RF-induced heating in a controlled experimental phantom environment simulating different background tissue thermal conductivities and to determine the potential thermal and physical basis for such a correlation using computer modelling with and without simulated tissue perfusion.
Materials and methods
Overview of experimental design
The effect of background tissue thermal conductivity on RF-induced heating was studied in a controlled system of agar phantoms measuring 12 cm in height and 10 cm in diameter. Two compartment agar phantoms of different background thermal conductivity were created by forming an inner compartment measuring 2 cm in diameter and 4 cm in length surrounded by background agar of differing thermal conductivities. The relationship between different background tissue thermal conductivities and RF heating at a defined 1 cm, 2 cm, 3 cm, 4 cm from the electrode (T1cm, T2cm, T3cm, T4cm) was established. Next, computer simulation of RF heating was performed using a two-dimensional finite element analysis program (ETherm). Parameters were selected to most closely approximate the model system. Computer generated temperature values were then compared with experimentally, empirically derived data. Once validated, computer modelling was then used to assess the effects of background thermal conductivity for fat and soft tissue of different sizes (2–4 cm) and different durations of heating (2–24 min), with and without simulated tissue perfusion (0–5 kg m−3 s−1) (n = 44 simulations).
Phantom experiments
Phantom construction
Two compartment agar phantoms were constructed to simulate an inner tumour surrounded by background tissue, as previously reported () Citation[20]. Briefly, agar phantoms were made by heating a solution of 5% agar, 3% sucrose, 5.0% NaCl (all from Fisher Scientific, Fairlawn, NJ) and a designated concentration of hydrogen-saturated fat solution (Crisco, J.M.Smucker Company; Orrville, OH; at 10, 25, 40 and 90%), in 1 l of distilled deionized water (resistivity >1.8 e−7 ohm-cm at 25°C) at 90°C. This solution was allowed to solidify in standardized 1.5 l cylindrical Pyrex® beakers to a temperature of 8°C for at least 6 h prior to use to ensure solidification. To form an inner well compartment, a cylindrical rod with a diameter of 2 cm was inserted to a depth of 4 cm within the phantom prior to refrigeration. After refrigeration, the cylindrical rod was removed and well depth measured. The phantoms were allowed to sit until they were at room temperature (16–18°C) to ensure that they were at the same baseline temperature as the water bath.
Figure 1. Experimental apparatus: An internally-cooled RF electrode (white arrow) has been inserted into an NaCl gel filled well within an Agar phantom, placed in a saline bath at a fixed distance from the grounding pad (G). Thermocouple probes (white curved arrow) have been inserted to measure temperature. An acrylic guide (open arrow) ensures proper positioning of the thermocouple. The RF generator and a temperature measurement device can be seen in the background.
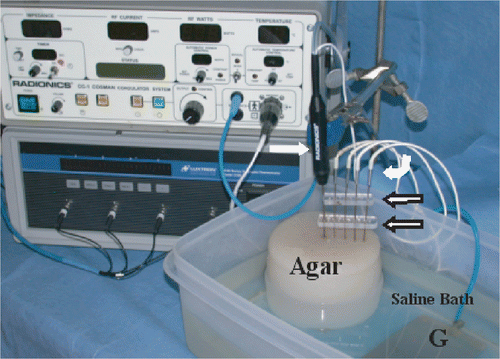
The central well of the agar phantoms were then filled with a solution of 0.25% agar, 3% sucrose and 5% NaCl (all from Fisher Scientific, Fairlawn, NJ) in 1 l of distilled deionized water (resistivity >1.8 e−7 ohm-cm at 25°C) at 90°C. The solution was allowed to cool to room temperature prior to use. To ensure experimental validity, at least three trials were performed for different outer concentrations (four outer concentrations × three trials = 12).
Thermal conductivity measurement
The cylindrical, hot-wire technique has been shown to be a simple and accurate method for measuring thermal conductivity of biomaterials Citation[21], Citation[22]. For this study, a novel thermal conductivity device was constructed consisting of a combination heater probe/thermocouple connected to a data acquisition system (FP-1000 and FP-TC-120 from National Instruments). Initial measurements were made on sponge, gelatin and styrofoam insulation to test the accuracy of the probe. Excellent agreement was reported between the measured and known standard values of thermal conductivity of these materials Citation[21]. Using the same probe, thermal conductivity measurements were subsequently performed upon the Agar phantom materials described above.
RF application
RF was applied by a single author (Y.W.) using a 500 kHz, monopolar radiofrequency generator (CC-1, Valleylab Inc., Boulder, CO) capable of delivering 2000 mA (into 35–65 Ω load impedances). Phantoms were placed on their base in room-temperature water (16°C) so that normal saline bathed the lower 7 cm of the phantom. The RF electrode was inserted to a depth of 3 cm vertically in the centre of the well. The electrical circuit was completed by submerging a standardized metal grounding pad (12.5 × 8 cm; Valleylab) into the saline water bath. To standardize background conductivity, the water was titrated accordingly with NaCl until reaching an impedance of 70 Ω. Internally-cooled, 17 gauge RF electrodes (CC-1020, Valleylab Inc., Boulder, CO) with 2 cm tip exposure delivered RF for 12 min Citation[23]. Electrode tip temperatures were maintained at 10–15°C during RF application by perfusing the electrode with 0°C water.
RF current was rapidly ramped to 2000 mA, the generator output current limit. The current limit of 2000 mA was chosen based upon the actual limitations of commercial generators, including the generator used in the agar phantom experiments. RF current remained at 2000 mA for the duration of the 2 min experiment. The amount of RF power deposited, as well as impedance was recorded every minute.
Temperature measurement
Phantom temperatures were measured (Y.W.) at 1 cm (i.e. in the well abutting the background materials), 2 cm, 3 cm and 4 cm distances from the RF electrode (i.e. in the background phantom material) using a series fluoroptic thermometer (Model 3100 Luxtron, Santa Clara, CA) with fibre diameter of 250 μm and total diameter of 0.5 mm placed to a depth of 2 cm (i.e. at the level of the mid-point of the RF electrode). Temperature sensor distance from the electrode was maintained by an acrylic-stabilizing block placed above the phantom. Baseline measurements of temperature, current, power and overall system impedance were recorded every 60 s.
Computer modeling
Finite element analysis was performed (Z.L.) over analytic solutions, given the complexity of the family of numeric solutions needed to solve the electrostatic equations Citation[24–26]. Comparative temperature profiles were created using computer simulation of Pennes’ Bio-Heat equation (ETherm, Field Precision, Albuquerque, NM) Citation[24], Citation[27]. To allow for comparison with phantom data, profiles were created assuming zero perfusion and parameter values were chosen to reflect the experimental conditions. These include: electrical conductivity 7 S m−1 for the outer compartment and 2 S m−1 for inner compartment and a specific heat of 3400 J kg−1°C. Thermal conductivity was varied as measured for both compartments and were in keeping with prior published reports Citation[20]. Computer simulated profiles were created for a distance of 1 cm, 2 cm, 3 cm and 4 cm away from electrode, with a maximum current output of 2000 mA. The current limit was chosen based upon the actual limitations of commercial generators, including the generator used in the agar phantom experiments. For these studies, seven thermal conductivities ranging from 0.1–0.6 W m−1°C were studied. Initial simulations had an inner compartment geometry (i.e. a well of 2 × 4 cm) and duration of RF application (2 min) similar to that of the phantom studies. Subsequent simulations compared background thermal conductivity of 0.46 W m−1°C (i.e. surrounding liver) to 0.23 W m−1°C (i.e. surrounding fat) for a 3 cm well at 6, 12 and 24 min and background thermal conductivity of 0.46 W m−1°C and 0.23 W m−1°C for 2, 3 and 4 cm tumours at 12 min. Finally, simulations were performed for two different background thermal conductivities, fat and soft tissue for different RF application times (6–24 min) and tumour sizes (2–4 cm) over a range of tissue perfusion. Five blood flow values representing the range of clinically relevant perfusion were studied including: 0 kg m−3 s−1 for a no flow state; 1 and 2 kg m−3 s−1 for hypoperfused tissue (equivalent to colorectal tumours and cirrhotic liver); 3.34 kg m−3 s−1 for normal liver; and 5 kg m−3 s−1 for hypervascular tumours were studied.
Statistical analysis
All data is presented as the mean ± standard deviation. To compare thermal conductivity of phantom and fat content, regression analysis between thermal conductivity and fat content of phantom was performed (Z.L., S.N.G.). Non-linear curve-fitting including logarithmic, exponential, Boltzman sigmoidal distributions of both agar phantom and computer simulated data was performed (Origin 6.1, Microcal Software Inc., Northampton, MA) to determine best fit, goodness of fit. Comparison of specified heating parameters was performed using student's T-test. For simulations involving tissue perfusion, regression analysis was performed for different RF ablation durations and simulated tumour sizes over a range of tissue perfusion.
Results
Phantom studies
Thermal conductivity.
In agar phantoms, increasing the amount of fat in the background tissue correlated with lower thermal conductivity. A negative exponential relationship was established between increased background fat concentration and the thermal conductivity (r2 = 0.98) (). The thermal conductivity of the inner well was 0.48 W m−1°C. Thermal conductivity of 10% fat was 0.46 W m−1°C (approximately that of liver) and of 90% fat was 0.23 W m−1°C (approximately that of fat) Citation[28].
RF heating.
For all phantom trials, baseline impedance measured 52.6 ± 6.2 Ω, generator output achieved a maximum of 2000 mA without pulsing. Significantly increased temperatures were observed at the edge of the inner compartment (1 cm from the electrode tip) as the fat content of the outer compartment increased (p < 0.01). Thus, temperatures at the edge at 2 min measured 31.5 ± 2.2°C for thermal conductivities of 0.46 W m−1°C (10% fat) vs. 45.1 ± 3.1°C for thermal conductivities of 0.23 W m−1 °C (90% fat) (p < 0.01). On the other hand, higher levels of fat in the outer compartment led to lower temperature increases (i.e. less heat penetration) in the background compartment. Thus, temperature at 2 cm from probe at 2 min measured 18.2 ± 0.3°C (Δ = 0.2 ± 0.3°C) for 90% fat vs. 19.4 ± 0.05°C (Δ = 1.1 ± 0.05°C) for 10% fat (p < 0.05). Accordingly, highest inner temperatures and lowest outer temperatures were achieved with 90% fat phantoms, resulting in a ‘flip’ in the temperature profiles for the inner compartment compared to the outer compartment ().
Figure 3. Temperature distribution in agar phantoms subject to RF heating. Temperatures recorded at 1 cm, 2 cm, 3 cm, 4 cm from the electrode show significantly increased temperature at 1 cm (the interface between the 0% fat inner compartment and the background tissue), but slightly lower temperature increase at 2 cm for 90% fat background.
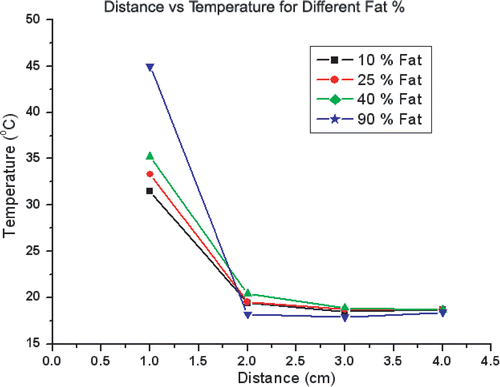
Computer simulation
The increased temperature at the edge of the inner compartment seen in the setting of decreased background thermal conductivity in the phantoms was also observed in computer simulation (). Additionally, computer simulation further demonstrated increased temperatures throughout the inner well. Furthermore, the lower temperature increases in the background (2 cm from electrode) seen in decreased background thermal conductivity setting and resultant ‘flip’ in temperature were also observed in the computer simulations.
Figure 4. Computer generated temperature profiles of RF heating for varied thermal conductivities. Over a wide range of background tissue thermal conductivities, increased temperatures are observed throughout the 1 cm inner compartment possessing a thermal conductivity of normal tissue (0.5 W m−1°C). For the background tissues, lower temperatures are seen in the presence of lower thermal conductivity. These thermal profiles are similar to those experimentally derived and presented in .
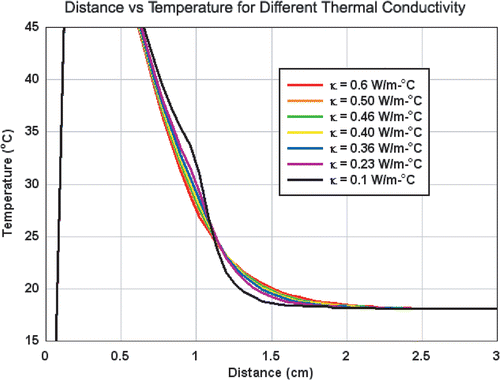
Correlation between computer and experimental studies
Good correlation between computer simulations and experimentally generated data was observed. Indeed, the correlation between the computer and experimental data was r2 = 0.93 (). The average error of temperature was 1.8 ± 2.4°C with a maximum error of 7.6°C. On a percentage basis, average error was 5.7 ± 5.6%.
Figure 5. Comparison of experimental and computer generated temperature profiles. Scatter plots of the measured temperatures for the four phantoms (squares) are compared to computer generated simulation data (diamonds). Overall, a tight linear correlation between computer simulations and experimentally generated data was observed (r2 = 0.93).
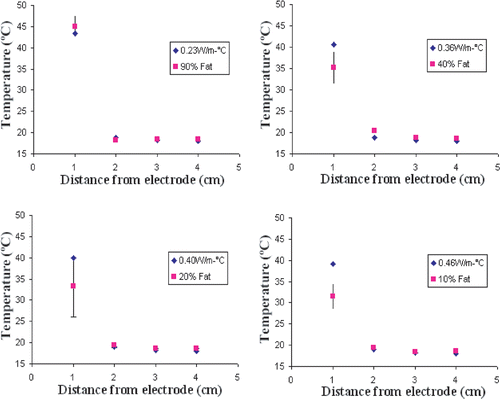
Temperature profiles of over time and for varied tumour sizes
Plotting the temperature profiles of a 3 cm tumour in two different background thermal conductivity surroundings (0.23 W m−1°C, 0.46 W m−1°C) over different time periods demonstrated that both temperatures and gradient between high and low thermal conductivity background tissues with increases over time (). Specifically, the temperature difference at the edge of tumour between two different backgrounds increases from 3.9°C at 6 min to 7.8°C at 24 min (). Plotting temperature profiles of computer simulations of different tumour sizes (2 cm, 3 cm, 4 cm in diameter) in two different thermal conductivity backgrounds (0.23 W −1°C, 0.46 W −1°C) at 12 min likewise demonstrated substantial difference in thermal gradient particularly between 1–2 cm from the electrode (i.e. the range of the radii of the 2–4 cm tumours). Temperature differences were 8.6°C at the 2 cm tumour margin and 2.7°C at the 4 cm tumour margin ().
Figure 6. Computer-modelled effect of perfusion on temperatures at the ‘tumour’ margin for tissues with different thermal conductivities with different RF application times. (a) Decreased thermal conductivity within background tissue (i.e. increased lipid content—0.23 W m−1°C for fat and 0.46 W m−1°C for normal soft tissue) can significantly increase the temperature at the margin of tumour in the absence of perfusion. This ‘oven’ effect can be magnified by increased RF ablation time. (b) The presence of perfusion reduces the ‘oven’ effect (i.e. the temperature difference, ΔT°C, between fat and soft tissue background) and the temperature rise at the margin of the tumour, but the range of variability of temperature at the margin of the tumour falls mostly within 50–65°C (depicted by the gray zone), the range of threshold temperatures for inducing necrosis in various tissues Citation[42]. (c) For all durations studied, there is a linear decrease in the ΔT°C at the tumour margin relative to increasing tissue perfusion (r2 = 0.99). Additionally, the negative slopes of ΔT°C for increasing perfusion is greater for longer RF durations (6–24 min).
![Figure 6. Computer-modelled effect of perfusion on temperatures at the ‘tumour’ margin for tissues with different thermal conductivities with different RF application times. (a) Decreased thermal conductivity within background tissue (i.e. increased lipid content—0.23 W m−1°C for fat and 0.46 W m−1°C for normal soft tissue) can significantly increase the temperature at the margin of tumour in the absence of perfusion. This ‘oven’ effect can be magnified by increased RF ablation time. (b) The presence of perfusion reduces the ‘oven’ effect (i.e. the temperature difference, ΔT°C, between fat and soft tissue background) and the temperature rise at the margin of the tumour, but the range of variability of temperature at the margin of the tumour falls mostly within 50–65°C (depicted by the gray zone), the range of threshold temperatures for inducing necrosis in various tissues Citation[42]. (c) For all durations studied, there is a linear decrease in the ΔT°C at the tumour margin relative to increasing tissue perfusion (r2 = 0.99). Additionally, the negative slopes of ΔT°C for increasing perfusion is greater for longer RF durations (6–24 min).](/cms/asset/1da05339-467c-4148-a3fd-b0192fd525be/ihyt_a_160895_f0006_b.gif)
Figure 7. Computer-modelled effect of perfusion on temperatures at the ‘tumour’ margin for tissues with different thermal conductivities with different tumour sizes. Decreased thermal conductivity within background tissue (i.e. increased lipid content—0.23 W m−1°C for fat and 0.46 W m−1°C for normal soft tissue) can significantly increase the temperature difference (ΔT°C) at the margin of tumour in the absence of perfusion (a) and with a perfusion of 2 kg m−3 s−1 (b). This ‘oven’ effect is diminished as tumour diameter increases. (c) Furthermore, there is a decreasing linear relationship between increasing perfusion and temperatures at the margin at the interface of tumour and surrounding tissue for all tumour sizes (2–4 cm in diameter) (r2 = 0.94–0.99).
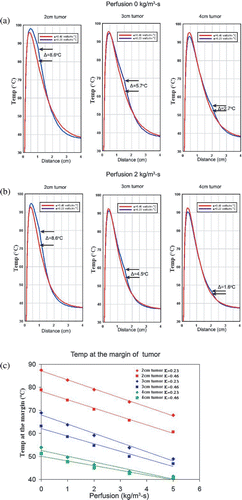
Effect of tissue perfusion
The simulated temperature difference (ΔT°C) observed at the tumour margin between tumours surrounded by tissues with different background thermal conductivity persisted in the presence of underlying tissue perfusion. For simulated 3 cm tumours, this decreased in linear fashion with increasing tissue perfusion for all RF application times (r2 = 0.99, ). Additionally, the negative slope of ΔT°C relative to perfusion increased with RF duration (6–24 min) denoting greater ΔT°C for longer RF duration at lower perfusion states. Temperature differences at the tumour margin persisted in the presence of underlying tissue perfusion for tumours of 2–4 cm in diameter, but also decreased in a linear manner with increasing tissue perfusion rates (r2 = 0.94–0.99, ). Nevertheless, the ΔT°C was dependent on and decreased with tumour size, as the average ΔT°C was 8.6, 4.5 and 1.6°C for 2, 3 and 4 cm tumours, respectively ().
Discussion
As RF ablation is increasingly applied in a wide range of tumour and tissue types Citation[6], Citation[11], Citation[29], Citation[30], further characterization and understanding of the role of intrinsic tumour and tissue characteristics will be required to optimize RF energy delivery paradigms and tumour coagulation. Several studies have demonstrated that differences in tumour and surrounding tissue characteristics including blood flow Citation[31], Citation[32], electrical Citation[18], Citation[20], Citation[33] and thermal conductivity Citation[19], Citation[34] can significantly influence RF energy deposition and tumour coagulation in both in vivo and clinical settings. For example, Livraghi et al. Citation[19] observed increased RF heating at the margins of hepatic tumours surrounded by cirrhotic liver and postulated that differences in thermal conductivity, an ‘oven effect’ of insulation, resulted in differences in overall coagulation.
This study isolates the effect of altered thermal conductivities in the surrounding environment on RF-induced heating in an ex-vivo experimental system and confirms these results with computer modelling. The results demonstrate that maximum heating within the inner ‘tumour’ compartment occurs at lower outer thermal conductivities, which impede heat transmission into the surrounding outer compartment. By the same token, increasing thermal conductivity in the outer surrounding compartment permitted greater heat transmission or ‘loss’ from the inner well, thus decreasing the maximum temperature achieved, but resulting in greater peripheral heating. This pattern, observed in ex-vivo studies with strong correlation to computer modelling studies, suggests that modifying the relationship of inner and outer thermal tissue conductivity, either by increasing the inner thermal conductivity or decreasing the outer, may permit more focused RF heating within the targeted area.
These results have several important implications for RF application in a clinical setting. The observation of lower thermal conductivity of background tissue leading to lower temperature increases in the background tissue correlates well with and indeed proves the hypothesis proposed by Dupuy et al. Citation[35], during RF ablation of the vertebral body, that the insulating effect of lower thermal conductivity cortical bone prevented thermal damage to nerve tissue in the spinal canal. Indeed, an appreciation of different thermal conductivities at these interfaces may allow more tailored RF application to both maximize heating within the tumour while minimizing damage to adjacent structures.
During RF ablation of primary hepatic tumours within cirrhotic liver, Livraghi et al. Citation[19] described an ‘oven effect’ in which increased heating was observed within the tumour secondary to postulated decreased thermal transmission of heat into surrounding cirrhotic liver compared (an insulatory effect) to normal liver tissue. These ‘oven effects’ likely hold true for other tumours surrounded by poor thermal conductivity include tumours surrounded by fat Citation[34], Citation[36] and bone Citation[35]. This is of particular clinical significance in solid organ tumours, where studies document the importance of achieving a 1 cm ‘ablative margin’ to target microscopic metastatic foci in peripheral tissue Citation[37]. These results suggest that differences in thermal conductivity and decreased peripheral tissue heating may restrict the achievable ‘ablative margin’ and, indeed, overall tumour coagulation efficacy. Alternatively, a greater understanding of thermal conductivity relationships may permit more tailored RF applications, and predict those situations which may require closer follow-up or repeated treatments. Additionally, these results will likely vary from patient to patient and, therefore, a non-invasive or minimally-invasive method for measuring thermal conductivity potentially become quite useful in clinical settings.
This study also underscores the role and potential of incorporating computer modelling in further characterization of complex RF-tissue characteristics. Several studies to date, including a recent study by Solazzo et al. Citation[33], demonstrate that computer modelling of the Bio-heat equation with mathematical correlation to ex-vivo studies provides a robust strategy to delineate the role of specific tissue characteristics on RF heating Citation[14], Citation[33], Citation[38]. Indeed, once validated, computer simulations enabled one to gain additional information including continuous thermal profiles, particularly for RF application at longer durations that were not possible to achieve in the phantoms due to their low melting points. Continued use of this strategy with ultimate application to in vivo settings will ideally permit optimization of RF application to suit a wide range of tumour types and tissue environments.
Additional computer modelling in this study of thermal conductivity in a two-compartment model demonstrates that differences in temperature profiles at the interface between the inner and outer compartment vary in a time-dependent fashion. Specifically, temperature differences at the interface are even more pronounced at clinically relevant RF ablation times (>12 min). This suggests that, while the RF heating patterns demonstrated in the ex vivo experiments are likely predictive, they in fact likely under-estimate expected differences in heating in clinical settings. Computer modelling also shows that the effects of background thermal conductivity play less of a role in larger tumours. This is logical as there is both less overall heating at larger distances from the point source of heating and because for larger tumours most of the overall heat transfer occurs in the tumour.
This study further confirms the importance of studying variables such as thermal conductivity in conjunction with other tissue parameters known to effect heating, such as perfusion Citation[39–41]. Indeed, the presence of perfusion does effect the temperature difference at the tumour margin (ΔT°C) induced by differences in inner and outer thermal conductivity. However, even in the presence of perfusion, the simulations suggest that differences in tissue thermal conductivity have clinically relevant effects on tissue heating profiles, particularly when taking into account a wide range of potential thermal sensitivities (50–65°C) to ablation Citation[42]. The modelling shows that, for 3 cm tumours treated for 12 mins (a common tumour size and treatment plan for the modelled electrode Citation[15], Citation[19]), a 4.5°C temperature difference based upon differences in tissue thermal conductivity was observed for a perfusion of 2 kg m−3 s−1 between 0.23–0.46 W m−1°C, suggesting that some targets would only be ablated when surrounded by tissues such as fat.
Increased RF duration times also magnified the thermal conductivity-induced temperature differences at the tumour margins in lower perfusion states. This is relevant in clinical settings, where heating to over 60°C results in decreased or eliminated blood flow secondary to coagulation of vasculature Citation[43]. Furthermore, there are many current clinical scenarios, such as surrounding fat or cirrhotic liver, where surrounding background tissue will demonstrate low-to-negligible blood flow, suggesting that increased RF duration times in these specific scenarios may yield increased heating at the tumour margins secondary to differences in tissue thermal conductivities.
When comparing the effect of different thermal conductivities on temperature differences at the tumour margins, greater differences were observed for smaller tumours. This likely reflects that the tumour-background tissue interface is positioned closer to the electrode for smaller tumours, where higher temperatures are generated and, therefore, temperature differences are more apparent. Although for some tumour sizes modelled for this electrode, the differences observed are not likely to be clinically relevant (as temperatures exceeded 65°C at the margin for all 2 cm tumours in both cases and the temperatures for 4 cm tumours were too low to achieve ablation), as other electrodes and RF application strategies are studied, scenarios where clinically relevant temperature differences are noted will likely emerge.
The underlying principle demonstrated in the study of the effect of the relationship of tissue thermal conductivities on RF tissue heating profiles, specifically at the tumour margins will likely be an important consideration in clinical practice, especially as RF ablation is applied in a wider and more diverse range of tissue and tumour types. There is a continued need for greater study, with modelling and characterization of other electrode systems. Additionally, the assumed perfusion in this study was equal for both the inner tumour zone and outer surrounding background tissue. Future investigation will be required to elucidate the effects of both perfusion and thermal conductivity in different tumour/surrounding tissue perfusion states and potential interactions with electrical conductivity.
While these initial results shed further insight into the role of RF-tissue interactions on RF-induced heating, further characterization of the effects of thermal conductivity is required. This study was performed within a standardized ex-vivo system and characterization in in-vivo models is required. Examining the influence of thermal conductivity combined with other parameters, such as electrical conductivity, may help in characterizing how these factors influence tumour coagulation in conjunction with each other. Indeed, the small discrepancies noted between the computer-modelling ex-vivo data suggests that certain inhomogeneities in the ex-vivo system may be due to other factors influencing RF energy deposition, including potential differences in specific heat that may be seen for tissues of varied fat content Citation[28]. Better appreciation of these changes may improve the modelling accuracy which currently has approximately a 5% error.
In conclusion, the results of this study characterize the role of thermal conductivity, in isolation, on RF ablation in a two-compartment model with excellent correlation to computer modelling. The varying heating pattern and relationship of inner and outer thermal conductivities explains observations of increased tumour heating noted in several clinical studies. Thus, clearly, investigation of these properties is essential for further understanding of how best to apply RF and other sources of thermal energy in different tissues. Specifically, given altered thermal effects not only locally around an electrode, but at tumour-tissue interfaces as well, this study also points to the importance of understanding and conceptualizing thermal ablation in terms of a two-compartment system comprising both the tumour and the surrounding background tissue.
Acknowledgements
Supported by a grant from the National Cancer Institute, National Institutes of Health, Bethesda, MD (RO1-CA87992-01A1) and Valleylab, Boulder, CO.
References
- Ahmed M, Goldberg SN. Thermal ablation therapy for hepatocellular carcinoma. J Vasc Interv Radiol 2002; 13(9 Suppl)S231–S244
- Gillams AR, Lees WR. Survival after percutaneous, image-guided, thermal ablation of hepatic metastases from colorectal cancer. Dis Colon Rectum 2000; 3: 656–661
- Buscarini L, Buscarini E. Percutaneous ultrasound-guided radiofrequency ablation of liver tumors. Liver Transpl Surg 1999; 5: 255–257
- Gervais DA, McGovern FJ, Arellano RS, McDougal WS, Muller PR. Renal cell carcinoma: clinical experience and technical success with radio-frequency ablation of 42 tumors. Radiology 2003; 226: 417–424
- Pautler SE, Pavlovich CP, Mikityansky I, Drachenberg DE, Choyke PL, Linehan WM, Wood BJ, Walther MM. Retroperitoneoscopic-guided radiofrequency ablation of renal tumors. Can J Urol 2001; 8: 1330–1333
- Pavlovich CP, Walter MM, Choyke PL, Paulter SE, Chang R, Linehan WM, Wood BJ. Percutaneous radio frequency ablation of small renal tumors: Initial results. J Urol 2002; 167: 10–15
- Zagoria RJ, Chen MY, Kavanagh PV, Torti FM. Radio frequency ablation of lung metastases from renal cell carcinoma. J Urol 2001; 166: 1827–1828
- Dupuy DE, Zagoria RJ, Akerley W, Mayo-Smith WW, Kavanagh PV, Safran H. Percutaneous RF ablation of malignancies in the lung. AJR Am J Roentgenol 2000; 174: 57–60
- Rosenthal DI, Hornicek FJ, Wolfe MW, Jennings LC, Gebhardt MC, Mankin HJ. Percutaneous radiofrequency coagulation of osteoid osteoma compared with operative treatment. J Bone Joint Surg Am 1998; 80: 815–821
- Callstrom MR, Charboneau JW, Goetz MP, Rubin J, Wong GY, Sloan JA, Novotny PJ, Lewis BD, Welch TJ, Farrell MA, et al. Percutaneous CT/US-guided radiofrequency ablation of painful metastases involving bone: A multicenter international study (abstract). Radiology 2002; 225: 163
- Mayo-Smith WW, Dupuy DE. Adrenal neoplasms: CT-guided radiofrequency ablation—preliminary results. Radiology 2004; 231: 225–230
- Wood BJ, Abraham J, Hvizda JL, Alexander HR, Fojo T. Radiofrequency ablation of adrenal tumors and adrenocortical carcinoma metastases. Cancer 2003; 97: 554–560
- Lamuraglia M, Lassau N, Garbay JR, Mathieu MC, Rouzier R, Jaziri S, Rouche A, Leclere J. Doppler US with perfusion software and contrast medium injection in the early evaluation of radiofrequency in breast cancer recurrences: A prospective phase II study. Eur J Radiol 2005; 56: 376–381
- Liu Z, Lobo SM, Humphries S, Horkan C, Solazzo SA, Hines-Peralta AU, Lenkinski RE, Goldberg SN. Radiofrequency tumor ablation: Insight into improved efficacy using computer modeling. AJR Am J Roentgenol 2005; 184: 1347–1352
- Solbiati L, Livraghi T, Goldberg SN, Ierace T, DellaNoce M, Gazelle GS. Percutaneous radiofrequency ablation of hepatic metastases from colorectal cancer: Long term results in 117 patients. Radiology 2001; 221: 159–166
- Livraghi T, Goldberg SN, Lazzaroni S, Meloni F, Ierace T, Solbiati L, Gazelle GS. Hepatocellular carcinoma: Radiofrequency ablation of medium and large lesions. Radiology 2000; 214: 761–768
- Abdalla EK, Vauthey JN, Ellis LM, Ellis V, Pollock R, Broglio KR, Hess K, Curley SA. Recurrence and outcomes following hepatic resection, radiofrequency ablation, and combined resection/ablation for colorectal liver metastases. Ann Surg 2004; 239: 818–25, discussion 825–827
- Ahmed M, Liu Z, Afzal KS, Weeks D, Lobo SM, Kruskal JB, Lenkinski RE, Goldberg SN. Radiofrequency tumor ablation: Effect of surrounding tissue composition on induced coagulation. Radiology 2004; 230: 761–767
- Livraghi T, Goldberg SN, Meloni F, Solbiati L, Gazelle GS. Hepatocellular carcinoma: Comparison of efficacy between percutaneous ethanol instillation and radiofrequency. Radiology 1999; 210: 655–663
- Lobo SM, Afzal KS, Ahmed M, Kruskal JB, Lenkinski RE, Goldberg SN. Radiofrequency thermal ablation using an adjuvant NaCl gel: Effect of electrical conductivity on tissue coagulation. Radiology 2004; 230: 175–182
- Bhattacharya A, Mahajan RL. Temperature dependence of thermal conductivity of biological tissues. Physiol Meas 2003; 24: 769–783
- Liang XG, Ge XS, Zhang YP, Wang GJ. A convenient method of measuring the thermal conductivity of biological tissue. Phys Med Biol 1991; 36: 1599–1605
- Goldberg SN, Gazelle GS, Solbiati L, Rittman WJ, Muller PR. Radiofrequency tissue ablation: Increased lesion diameter with a perfusion electrode. Acad Radiol 1996; 3: 636–644
- Humphries S, Platt RC, Ryan TR. ETherm finite element modeling of electrical heating and non-linear thermal transport in biological media. Proc Am Soc Mech Eng High Temp 1997; 355: 131–134
- Tungjitkusolmun S, Staelin ST, Haemmerich D, Tsai JZ, Webster JG, Lee FT, Jr, Mahvi DM, Vorperian VR. Three-dimensional finite-element analyses for radio-frequency hepatic tumor ablation. IEEE Trans Biomed Eng 2002; 49: 3–9
- Jain MK, Wolf PD. Temperature-controlled and constant-power radio-frequency ablation: What affects lesion growth?. IEEE Trans Biomed Eng 1999; 46: 1405–1412
- Humphries S. Field solutions on computers. CRC Press, Boca Raton, FL 1997, Sects. 11.3, 11.4, 12.2 and 12.3
- Duck FA. Physical properties of tissue: A comprehensive reference book. Academic Press, San Diego, CA 1990
- Dodd GD, III, Soulen MC, Kane RA, Livraghi T, Lees WR, Yamashita Y, Gillams AR, Karahan OI, Rhim H. Minimally invasive treatment of malignant hepatic tumors: At the threshold of a major breakthrough. Radiographics 2000; 20: 9–27
- Rossi S, Buscarini E, Garbagnati F. Percutaneous treatment of small hepatic tumors by an expandable RF needle electrode. AJR Am J Roentgenol 1998; 170: 1015–1022
- Yamakado K, Nakatsuka A, Akeboshi M, Takeda K. Percutaneous radiofrequency ablation of liver neoplasms adjacent to the gastrointestinal tract after balloon catheter interposition. J Vasc Interv Radiol 2003; 14: 1183–1186
- Schultze D, Morris CS, Bhave AD, Worgan BA, Najarian KE. Radiofrequency ablation of renal transitional cell carcinoma with protective cold saline infusion. J Vasc Interv Radiol 2003; 14: 489–492
- Solazzo SA, Liu Z, Lobo SM, Ahmed M, Hines-Peralta AU, Lenkinski RE, Goldberg SN. Radiofrequency ablation: Importance of background tissue electrical conductivity—an agar phantom and computer modeling study. Radiology 2005; 236: 495–502
- Boehm T, Malich A, Reichenbach JR, Fleck M, Kaiser WA. Percutaneous radiofrequency (RF) thermal ablation of rabbit tumors embedded in fat: A model for RF ablation of breast tumors. Invest Radiol 2001; 36: 480–486
- Dupuy DE, Hong R, Oliver BS, Goldberg SN. Radiofrequency ablation of spinal tumors: Temperature distribution in the spinal canal. AJR Am J Roentgenol 2000; 175: 1263–1266
- Ahmed M, Lobo SM, Weinstein J, Kruskal JB, Gazelle GS, Halpern EF, Afzal SK, Lenkinski RE, Goldberg SN. Improved coagulation with saline solution pretreatment during radiofrequency tumor ablation in a canine model. J Vasc Interv Radiol 2002; 13: 717–724
- Goldberg SN, Dupuy DE. Image-guided radiofrequency tumor ablation: Challenges and opportunities - Part I. JVIR 2001; 12: 1021–1032
- Zheng Z, McCormick D, Dairywala I, Surabhi S, Goldberg SN, Turi Z, Vannan MA. Catheter-based intracardiac echocardiography in the interventional cardiac laboratory. Catheter Cardiovasc Interv 2004; 63: 63–71
- Goldberg SN, Hahn PF, Tanabe KK, Mueller PR, Schima S, Athanasoulis CA, Compton CC, Solbiati L, Gazelle GS. Percutaneous radiofrequency tissue ablation: Does perfusion-mediated tissue cooling limit coagulation necrosis?. J Vasc Interv Radiol 1998; 9: 101–111
- Boehm T, Malich A, Reichenbach JR, Fleck M, Kaiser WA. Percutaneous radiofrequency ablation of hepatic tumors during temporary venous occlusion. AJR Am J Roentgenol 2002; 178: 53–59
- Patterson EJ, Scudamore CH, Owen DA, Nagy AG, Buczkowski AK. Radiofrequency ablation of porcine liver in vivo: Effects of blood flow and treatment time on lesion size. Ann Surg 1998; 227: 559–565
- Mertyna P, Peddi H, Pirani N, Liu Z, Ahmed M, Goldberg SN. Marked differences in tumor sensitivity to RF ablation with and without liposomal chemotherapy. Radiology 2005; 235: 544
- Goldberg SN, Gazelle GS, Compton CC, Muller PR, Tanabe KK. Treatment of intrahepatic malignancy with radiofrequency ablation: Radiologic-pathologic correlation. Cancer 2000; 88: 2452–2463