Abstract
Background: In the Academic Medical Center (AMC) Amsterdam, locoregional hyperthermia for oesophageal tumours is applied using the 70 MHz AMC-4 phased array system. Due to the occurrence of treatment-limiting hot spots in normal tissue and systemic stress at high power, the thermal dose achieved in the tumour can be sub-optimal. The large number of degrees of freedom of the heating device, i.e. the amplitudes and phases of the antennae, makes it difficult to avoid treatment-limiting hot spots by intuitive amplitude/phase steering.
Aim: Prospective hyperthermia treatment planning combined with high resolution temperature-based optimization was applied to improve hyperthermia treatment of patients with oesophageal cancer.
Methods: All hyperthermia treatments were performed with ‘standard’ clinical settings. Temperatures were measured systemically, at the location of the tumour and near the spinal cord, which is an organ at risk. For 16 patients numerically optimized settings were obtained from treatment planning with temperature-based optimization. Steady state tumour temperatures were maximized, subject to constraints to normal tissue temperatures. At the start of 48 hyperthermia treatments in these 16 patients temperature rise (ΔT) measurements were performed by applying a short power pulse with the numerically optimized amplitude/phase settings, with the clinical settings and with mixed settings, i.e. numerically optimized amplitudes combined with clinical phases. The heating efficiency of the three settings was determined by the measured ΔT values and the ΔT-ratio between the ΔT in the tumour (ΔToes) and near the spinal cord (ΔTcord). For a single patient the steady state temperature distribution was computed retrospectively for all three settings, since the temperature distributions may be quite different. To illustrate that the choice of the optimization strategy is decisive for the obtained settings, a numerical optimization on ΔT-ratio was performed for this patient and the steady state temperature distribution for the obtained settings was computed.
Results: A higher ΔToes was measured with the mixed settings compared to the calculated and clinical settings; ΔTcord was higher with the mixed settings compared to the clinical settings. The ΔT-ratio was ∼1.5 for all three settings. These results indicate that the most effective tumour heating can be achieved with the mixed settings. ΔT is proportional to the Specific Absorption Rate (SAR) and a higher SAR results in a higher steady state temperature, which implies that mixed settings are likely to provide the most effective heating at steady state as well. The steady state temperature distributions for the clinical and mixed settings, computed for the single patient, showed some locations where temperatures exceeded the normal tissue constraints used in the optimization. This demonstrates that the numerical optimization did not prescribe the mixed settings, because it had to comply with the constraints set to the normal tissue temperatures. However, the predicted hot spots are not necessarily clinically relevant. Numerical optimization on ΔT-ratio for this patient yielded a very high ΔT-ratio (∼380), albeit at the cost of excessive heating of normal tissue and lower steady state tumour temperatures compared to the conventional optimization.
Conclusion: Treatment planning can be valuable to improve hyperthermia treatments. A thorough discussion on clinically relevant objectives and constraints is essential.
Introduction
In the Academic Medical Center (AMC) at the University of Amsterdam locoregional hyperthermia is applied using the 70 MHz AMC-4 phased array system Citation[1]. For optimal treatment outcome a uniform tumour temperature is pursued, which can be difficult to achieve Citation[2–4]. The goal tumour temperature Citation[5] is usually 43°C for 1 h, but the thermal dose achieved in the patient can be sub-optimal due to the occurrence of treatment-limiting hot spots in normal tissue and systemic stress at high power. Such limitations are difficult to avoid intuitively due to the large number of degrees of freedom of the heating device, i.e. the amplitudes and phases of the four antennae.
Several numerical optimization techniques have been described in the literature to obtain amplitude/phase settings for optimal tumour heating. Optimization methods based on Specific Absorption Rate (SAR) Citation[6–9] are more widely applied than temperature-based methods Citation[7], Citation[10], Citation[11]. However, temperature-based optimization is clinically more relevant than SAR optimization since hot spots will correspond to temperature rather than to SAR and several important factors like perfusion, conduction and bolus cooling are not taken into account when optimizing a SAR distribution Citation[12], Citation[13]. For adequate treatment planning it is also necessary to perform simulations at high resolution. At low resolution small anatomical structures can be modelled incorrectly or even missed, which can result in an erroneous prediction of hot spots Citation[13], Citation[14]. Computer limitations hamper E-field calculations at high resolution and thereby direct high resolution optimization. A quasi-static zooming technique has been developed to calculate the power density at high resolution Citation[14]. This quasi-static zooming has been combined with a temperature-based optimization method to perform high resolution optimization Citation[13].
Oesophageal tumours are more difficult to heat than for example pelvic tumours due to the cooling capabilities of the lungs, heart and aorta. Moreover, because of the close anatomical relation between the oesophagus and the spinal cord, more prudence is needed to prevent neurological toxicity due to overheating of the spinal cord. To overcome these difficulties treatment planning could be of clinical value.
Purpose
To improve locoregional hyperthermia treatment of patients with oesophageal cancer, prospective treatment planning combined with high resolution temperature-based optimization Citation[13] was applied in the present study. SAR measurements were performed at the beginning of hyperthermia treatments to compare the heating efficiency of numerically optimized and clinical amplitude/phase settings.
Furthermore, the relation between local SAR measurements and the amplitude/phase settings of the antennae together with the patients’ dorsoventral and lateral diameters was examined to obtain more insight in the value of treatment planning and the power steering capabilities of the heating device.
Patients and methods
Patients
In 2002 a feasibility study Citation[15] was completed, applying neo-adjuvant locoregional hyperthermia combined with chemotherapy (cisplatinum + etoposide) in patients with operable oesophageal cancer. Since it was concluded that hyperthermia was feasible for oesophageal cancer, a phase II study for the treatment of stage II or III oesophageal cancer was started in August 2003, applying 41.4 Gy radiotherapy in 1.8 Gy daily fractions in combination with weekly locoregional hyperthermia for 5 weeks and concurrent chemotherapy (carboplatin + paclitaxel). An oesophageal resection was planned 4–6 weeks after completion of the neo-adjuvant thermochemoradiation. A total of 28 patients have been treated so far, with a median tumour length of 6 cm (range 2–12 cm) at the start of the first hyperthermia treatment. All tumours were located below the carina level with mostly no cardia extension or an extension less than 2 cm. Pre-treatment tumour stage consisted mainly of stage III tumours; 75% of the patients had an adenocarcinoma and 25% had a squamous cell carcinoma.
Treatment planning
The treatment planning software has been developed at the Department of Radiotherapy of the University Medical Center Utrecht Citation[16] and was expanded at the Department of Radiation Oncology of the AMC. The planning was performed for 16 patients and was based on a CT scan of the patient in treatment position (i.e. prone position) on a water bolus and mattresses, identical to those used during hyperthermia treatment. A total length of 60 cm was scanned with a slice thickness of 5 mm. The tumour, heart, aorta and spinal cord were outlined manually by a radiation oncologist. The rest of the data set was segmented semi-automatically into muscle-like tissue, fatty tissue, bone, lung and inner air (e.g. in bowels) using a histogram of the Hounsfield Units of the CT data set Citation[17]. The segmented data set was downscaled using the ‘winner take all’ method Citation[18], which means that a low resolution voxel is assigned the dielectric and thermal properties of the most frequently occurring tissue type of the corresponding high resolution voxels. Dielectric and thermal properties, including perfusion, used for the simulations were obtained from literature Citation[19], Citation[20] (see ). The volumetric perfusion rate Wb used in the simulations was constant, but an increase due to hyperthermia Citation[21] was taken into account by using increased values Citation[19], Citation[20] compared to resting tissue. The E-fields were calculated at a resolution of 1 × 1 × 1 cm3. Quasi-static zooming Citation[14] was combined with a temperature-based optimization method to perform high resolution optimization Citation[13] at 2 × 2 × 5 mm3. The goal tumour temperature was 43°C and constraints to normal tissue were set to 42°C. During hyperthermia treatment the temperature measured in the back musculature, close to the spinal cord, is not allowed to exceed 41°C to prevent over-heating of the spinal cord. The temperature in the spinal cord is likely to be somewhat lower than the temperature in the back musculature, so a constraint of 40°C was chosen for the spinal cord in the numerical optimization. In addition, power constraints were set to prevent clinically irrelevant settings. No antenna was allowed to supply less than 10% or more than 40% of the total power. The heart and aorta were kept at a constant temperature of 37.5°C (the systemic temperature, being slightly elevated due to the hyperthermia) during the simulations, which is realistic due to their very high blood flow. The temperature of the waterbolus was kept constant at 12°C.
Table I. Values of the dielectric and thermal properties used in the simulations, for different tissue types at 70 MHz and under hyperthermia conditions; conductivity (σ(S m−1)), relative permittivity (εr (−)), density (ρ (kg m−3)), specific heat capacity (c (J kg−1°C−1)), thermal conductivity (k (W m−1°C−1)) and perfusion (Wb (kg m−3 s−1)).
Treatment set-up and measurements
Patients were treated in prone position in the AMC-4 waveguide system. Clinical experience has led to standard power ratios for the four antennae for different tumour locations, which are considered clinically optimal for each specific tumour site. For oesophageal tumours, this has led to standard power ratios for the top, bottom, left and right antenna of 1 : 3 : 3 : 3. Power is proportional to the square of the amplitude of the E-field. The top antenna, which is closest to the spinal cord, delivers less power to prevent over-heating of the spinal cord. Phase settings are clinically optimized by maximizing the E-field, measured at the tumour site using an E-field probe, where the top antenna is the reference (i.e. phase 0°). The standard clinical amplitude settings, together with the clinically optimized phases are referred to as ‘clinically optimized settings’. Tumour, spinal cord and systemic temperatures were monitored using multi-sensor thermocouple probes. A thermometry catheter in the back musculature at the level of the tumour was inserted under local anaesthetics to monitor the temperature near the spinal cord, which was not allowed to exceed 41°C. Tumour temperatures were measured using two thermocouple probes mounted on opposite sides of an inflatable balloon catheter in the oesophagus. The systemic temperature was measured rectally. shows a patient during a hyperthermia treatment and a schematic representation of the thermometry.
Figure 1. Patient set up in the 70 MHz AMC-4 phased array locoregional hyperthermia system during treatment (left) and a schematic representation of the applied thermometry (right).
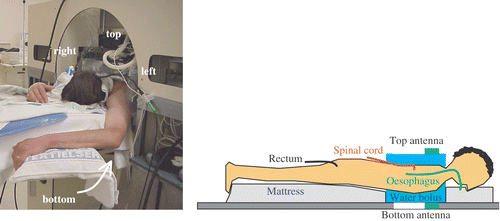
At the start of treatment ΔT-measurements Citation[22] were performed by applying a 30 s power pulse of 800 W (see ). The ΔT is the temperature rise in these 30 s. A pre-pulse of 5 s was applied, followed by a power-off period of 5 s. Next the 30 s power pulse was applied, also followed by a power-off period of 5 s. The ΔT is the difference between the measured temperatures after both power-off periods. Such a technique has the advantage that any contribution of self-heating of the thermocouple is equal for both measurements and is, thus, eliminated by subtraction Citation[22].
Amplitude/phase settings applied for ΔT-measurements
At the start of all hyperthermia treatments ΔT-measurements were performed with clinically optimized amplitude/phase settings. For 48 treatments in 16 patients, two additional settings were applied for the ΔT-measurements. The following settings were tested in random order:
Calculated: The numerically optimized amplitude/phase settings;
Clinical: The clinically optimized amplitude/phase settings; and
Mixed: The numerically optimized amplitudes with the clinically optimized phases.
Hyperthermia treatment
The hyperthermia treatment was performed with the clinically optimized settings for 1 h at steady state temperature. Temperatures were measured every 30 s after a 5 s power-off period. Steady state tumour temperatures were expressed in terms of T10, T50 and T90, i.e. the temperature at least registered by 10%, 50% and 90% of thermosensors in the target area, respectively, over the duration of the steady state period.
Analysis
The clinical merit of the different amplitude/phase settings was evaluated by analysis of the results of the ΔT-measurements. The mean value of ΔT measured at the oesophageal tumour is ΔToes. The deepest thermocouple sensor in the back musculature, i.e. the sensor closest to the spinal cord, represents ΔTcord, the ΔT near the spinal cord. In addition to the ΔT values the ratio ΔToes/ΔTcord was determined to provide a criterion for tumour heating efficiency. A high ΔT-ratio means a high temperature rise in the tumour compared to the spinal cord and, thus, effective tumour heating.
The ΔT values and ΔT-ratios measured for the three different settings were compared using ANOVA with a Bonferroni post-hoc test and are presented as mean ± SEM, averaged over the 48 treatments. A value of p < 0.05 was considered statistically significant.
To obtain more insight in the value of treatment planning and the power steering capabilities of the heating device, the relation between ΔToes, ΔTcord and the amplitude/phase settings of the different antennae was studied for the 48 treatments, applying multi-variate analysis. The patients’ dorsoventral (AP) and lateral (LAT) diameters at the tumour location, determined using the planning CT-scan (), were also incorporated in the multi-variate analyses to account for a possible influence of patient dimensions on the achieved temperature rise. Partial correlations were determined with SPSS and a value of p < 0.05 was considered statistically significant.
Computed ΔT values and steady state temperature distributions
The calculated settings were obtained by numerically optimizing the tumour temperature, subject to normal tissue constraints, while the clinical and mixed settings were acquired by adjusting the phases at the start of treatment in order to optimize the measured E-field at the tumour for the amplitudes concerned. Since the clinical and numerical optimization objectives are different, the clinical, calculated and mixed settings will probably be quite different as well as the corresponding SAR and temperature distributions.
To illustrate this, the SAR distribution resulting from the clinical, calculated and mixed settings was computed for patient number 12 and normalized to the amount of power applied in the clinic (800 W). The ΔT values and their ratio after a 30 s power pulse as well as the steady state temperature distributions were compared. Perfusion values of tissue under normothermic conditions Citation[21] were used for calculation of the ΔT values, since the start of treatment was modelled in this case. The perfusion values were chosen a factor five lower than listed in . Heart and aorta were kept at a constant systemic temperature of 37°C instead of 37.5°C.
In the clinic, ΔToes is the mean value of the ΔTs measured at the location of the oesophageal tumour. Temperatures are measured intra-luminally at the oesophageal wall and not invasively in the tumour. Since a homogeneous tumour temperature is generally difficult to achieve and the creation of the SAR focus is also based on intra-luminal E-field measurements, the measured ΔToes is probably closer to the maximum than to the average temperature rise in the tumour. Therefore, in this computed example of patient 12, the maximum tumour temperature rise was taken as ΔToes. ΔTcord was represented by the temperature rise of a voxel of the back musculature near the spinal cord.
Numerical optimization on ΔT-ratio
To illustrate that the choice of the optimization strategy is decisive for the obtained settings, optimization on ΔT-ratio was performed for patient 12. The ratio Toes/Tcord after 30 s was maximized, where Tcord is the average temperature in a rectangular volume in the back musculature and Toes is the average temperature in the tumour. This is similar to maximizing ΔToes/ΔTcord, but has the advantage that of two different settings yielding the same ΔT-ratio, the one generating the highest temperature rise will be preferred, which is advantageous for the steady state tumour temperature. No constraints to normal tissue were imposed. Again, perfusion values of tissue under normothermic conditions were used and the heart and aorta were kept at a constant temperature of 37°C.
The ΔT-ratio was evaluated using the maximum tumour temperature rise (ΔToes) and the temperature rise in one voxel of the rectangular volume, close to the spinal cord (ΔTcord). The steady state temperature distribution was computed and compared with the temperature distributions computed for the clinical, calculated and mixed settings.
Results
shows the clinical, calculated and mixed power ratios and phase settings that were tested at the beginning of a hyperthermia treatment of patient 12. The top antenna is the reference, i.e. phase 0°. The applied power was set to 800 W for all ΔT-measurements. Note that the calculated settings are quite different from the clinical settings. Moreover, a strong asymmetry can be observed between the power applied by the left and right antenna. Similar asymmetric amplitudes were observed in almost all numerically optimized settings. The measured ratios ΔToes/ΔTcord were 0.49/0.25 = 2.0, 1.04/0.31 = 3.4 and 1.16/0.23 = 5.0 for the calculated, mixed and clinical settings, respectively.
Table II. Example of calculated, clinical and mixed power ratios (P) and phase settings (Φ) for patient 12, normalized to the top antenna. The top antenna is the reference, i.e. phase 0°.
shows three orthogonal slices of the steady state temperature distribution resulting from the calculated settings of . The heart and the aorta are clearly visible as large volumes of 37.5°C. The patient is in prone position and left and right in these figures correspond to left and right of the patient. In the right flank the constraint of 42°C was met, while in the left flank the temperature remains below this constraint. However, in the coronal slice of , an arising hot spot of 42°C can be observed in the stomach between the left applicator and the tumour, which acts as a limitation for the left antenna, so these settings are optimal in compliance with the constraints set to normal tissue.
Figure 4. Transversal, coronal and sagittal slices of the numerically optimized temperature distribution and the corresponding anatomy of patient 12. The tumour temperature was maximized, taking constraints of 42°C to normal tissue and 40°C to the spinal cord into account.
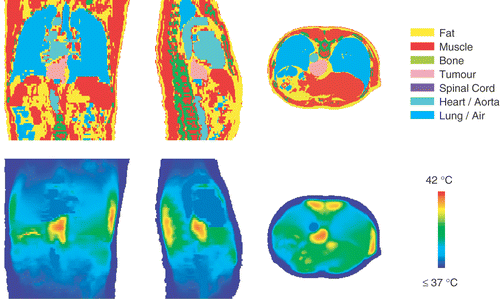
ΔT-measurements
The average results of the ΔT-measurements for 48 treatments in 16 patients are shown in . The measured ΔToes was significantly higher with the mixed settings than with the other two settings. A significantly higher ΔTcord was found with the mixed settings compared to the clinical settings, while the ratio ΔToes/ΔTcord was not significantly different for the three settings.
Table III. Results of ΔT-measurements performed with calculated, clinical and mixed settings, averaged over 48 treatments (mean ± SEM) in 16 patients.
Multi-variate analysis
The ΔT near the spinal cord was mainly dependent on the power of the top antenna. The partial correlation coefficient was R = 0.34 (p < 0.05). The patients’ diameters also had a significant influence on ΔTcord. The partial correlations for the dorsoventral and lateral diameters were R = −0.22 and R = −0.31 (p < 0.05), respectively. The ΔT in the oesophagus depended mainly on the patient's dimensions. A partial correlation coefficient of R = −0.22 and R = −0.21 (p < 0.05) was found for the dorsoventral and lateral diameters, respectively. The partial correlations between diameters and ΔT values were negative, implying that larger diameters resulted in lower ΔT values.
Computed ΔT values and steady state temperature distributions
For the case in , the computed ΔT values and steady state temperature distributions were compared for the calculated, clinical and mixed settings. The amount of power was normalized to the amount applied in the clinic (800 W). The measured ratios ΔToes/ΔTcord were 2.0, 3.4 and 5.0 for the calculated, mixed and clinical settings, respectively. Treatment planning calculations yielded ΔT-ratios of 2.4, 2.8 and 3.5.
For this example, both simulations and clinical measurements showed the highest ratio ΔToes/ΔTcord with the clinical settings, followed by the mixed settings. The calculated settings showed the lowest ΔT-ratio. shows that the computed ΔToes as well as the computed steady state tumour temperatures resulting from the calculated, clinical and mixed settings were comparable. However, computed steady state temperature distributions showed that the clinical and mixed settings yielded a maximum normal tissue temperature of 45.1°C and 44.9°C, respectively. The calculated settings resulted in a maximum of 43.2°C (before power scaling this maximum just reached the enforced constraint of 42°C). This demonstrates that the numerical optimization did not prescribe the mixed or clinical settings, because it had to comply with the constraints set to the normal tissue temperatures.
Table IV. The clinically measured (M) and computed (C) ratios ΔToes/ΔTcord for patient 12, resulting from the calculated, clinical and mixed settings. The simulated steady state tumour temperatures T10, T50 and T90 are also listed, together with the maximum temperature in normal tissue (Tmax) and the temperature in the back musculature, near the spinal cord (Tcord).
Numerical optimization on ΔT-ratio
The power ratios returned by optimization on ΔT-ratio were 1.0 : 4.0 : 2.4 : 2.6 for the top, bottom, left and right antenna. The top antenna delivers 10% of the total power, which was a constraint for the minimal amount of power supplied by each antenna. The bottom antenna is more effective for tumour heating than the left and right antenna and reaches the maximum of 40% of the total power. The remaining power (50%) is distributed almost evenly over the left and right antenna. When using the top antenna as reference (i.e. phase 0°), the phases of the bottom, left and right antenna were approximately equally negative: −110°, −120° and −117°, respectively. This corresponds to a phase shift of ∼110° for the top antenna only, thereby shifting the power focus more to the bottom antenna and preventing power absorption in the back musculature. The computed ratio ΔToes/ΔTcord was 6.67 × 10−1/1.755 × 10−3 = 380. With these settings and the total power normalized to the amount applied in the clinic (800 W), the simulated steady state temperatures T10, T50 and T90 were 40.3, 39.4 and 38.2°C, respectively. The maximum normal tissue temperature was 47.3°C, whereas the back musculature near the spinal cord was only 37.02°C at steady state. The maximum temperature in the spinal cord itself was 37.6°C.
shows transversal slices of the steady state temperature distribution resulting from the settings prescribed by optimization on ratio as well as the temperature distribution resulting from the calculated, clinical and mixed settings.
Figure 5. Transversal slices of the steady state temperature distribution of patient 12 resulting from the calculated, clinical and mixed settings as well as the steady state temperature distribution resulting from the settings prescribed by optimization on ΔT-ratio. In all cases, the total power was normalized to the amount applied in the clinic (800 W).
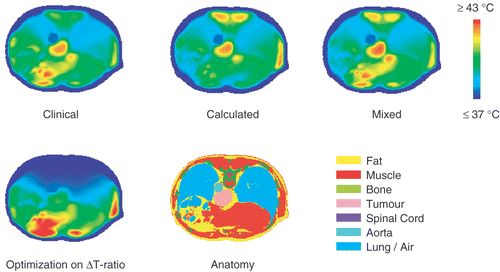
Discussion
Clinical applications of hyperthermia treatment planning so far were of retrospective nature Citation[23–26]. In the present study prospective hyperthermia treatment planning combined with high resolution temperature-based optimization was applied to patients with oesophageal cancer, treated with hyperthermia combined with chemoradiation. At the start of hyperthermia treatments temperature rise (ΔT) measurements were performed to compare the heating efficiency of numerically optimized amplitude/phase settings with clinically optimized settings and with a combination of clinically and numerically optimized settings, i.e. mixed settings. The ratio between the ΔT in the oesophagus and the ΔT near the spinal cord was not significantly different for these three settings, while the highest ΔT values were observed with the mixed settings. This implies that with the mixed settings the same tumour heating can be achieved with less applied power, indicating more effective heating.
The mixed settings combine numerically optimized amplitudes with clinically optimized phases. Another candidate for mixed settings would have been the combination of standard clinical amplitudes and numerically optimized phase settings. However, because ΔT-measurements are time consuming, it is not feasible to examine too many different settings.
SAR and steady state temperature
The initial ΔT is proportional to SAR, since perfusion and conduction can be neglected during the short power pulse at the start of treatment. However, a high SAR does not always result in a high steady state temperature, or a low SAR in a low temperature, mainly due to the varying perfusion values of different tissue types. Especially at tissue boundaries the relation between SAR and steady state temperature is complicated because of inhomogeneous dielectric and thermal properties. Specifically at superficial locations temperatures are affected by bolus cooling.
In the present study, a correlation of ∼0.5 was found between ΔT and steady state temperatures, indicating that the steady state temperature is not fully determined by SAR. The oesophagus is in close vicinity to the heart, aorta and lungs, which have a large cooling capability affecting the steady state tumour temperature. Nevertheless, a higher power deposition will result in a higher temperature, so the results of the ΔT-measurements can to some extent be extrapolated to steady state.
Optimization techniques
The location of the power focus in the tumour is created mainly by phase steering. In the clinic phases are optimized by maximizing the E-field, measured at the tumour site using an E-field probe. Clinical optimization of amplitude settings is difficult and time-consuming. Therefore, standard settings based on clinical experience are applied for different tumour locations.
The ultimate clinical goal in hyperthermia treatment planning is a reliable planning system that provides the opportunity to find amplitude/phase settings yielding optimal temperatures in the target area, while respecting user-defined constraints to normal tissue. Therefore, the calculated settings were not optimized for the best ΔT-ratio, but for the highest possible tumour temperature while respecting normal tissue constraints. In fact, none of the calculated, clinical and mixed settings were optimized for the highest ΔT-ratio, but the ΔT values and their ratio were convenient criteria to judge the quality of the three different settings, since clinical thermometry during treatment was limited to the tumour location, the rectum and the back musculature near the spinal cord.
Settings very different from those listed in were prescribed with the ΔT-ratio as the objective of the numerical optimization and without temperature constraints to normal tissue. Additional constraints on the amplitudes are particularly necessary for optimization on ΔT-ratio. Without these constraints it might be optimal to simply switch off the top antenna and deliver almost all power by the bottom antenna, which is not regarded a clinically relevant strategy. Moreover, it is not really necessary to pursue such a high ΔT-ratio that the spinal cord temperature remains close to 37°C.
The computed steady state tumour temperatures resulting from the settings prescribed with optimization on ΔT-ratio were lower than with the settings prescribed by the conventional optimization strategy, despite the very high ΔT-ratio of 380. This example demonstrated that the optimization is quite capable of optimizing any required objective, but the choice of this objective and other input parameters and constraints is crucial for the resulting settings and steady state temperatures.
ΔT-ratio and steady state temperature distribution
For patient 12 the ΔT-ratio and steady state temperatures were computed for the calculated, clinical and mixed settings. The calculated settings yielded the distribution with the lowest ΔT-ratio, but the computed steady state temperature distributions for the clinical and mixed settings exceeded the normal tissue constraints used in the optimization. This explains why neither clinical nor mixed settings were prescribed by the numerical optimization.
The calculated and mixed settings differ in phase setting only, so apparently the phases of the mixed settings are more favourable for ΔToes, indicating a better focus of the local SAR in the target. The clinical and mixed settings differ in amplitude setting only, so apparently the calculated amplitudes are more favourable for ΔToes. The top and bottom antenna are more effective for tumour heating than the left and right antenna. For the example shown in the top and bottom antenna together delivered 40% and 62% of the total power for the clinical and mixed settings, respectively. This might explain why the mixed settings yielded the highest ΔToes, averaged over all 48 treatments.
The clinical relevance of the computed hot spots is uncertain. Clinical thermometry is limited and hot spots will only be observed when pain complaints occur, with the exception of the location of the thermocouple probe near the spinal cord. In the clinical practice pain complaints generally do not occur, so whether the restrictions imposed on the numerical optimization are clinically relevant remains subject of investigation. Possibly, the constraints applied in this study were too strict and/or the computed temperature levels were not fully correct. This also supports the conclusion of the previous paragraph that the choice of objective and constraints is important for the achieved settings and temperatures.
Uncertainties in treatment planning
The difference in computed ΔT-ratio for the three settings showed the same trend as the clinical measurements, but quantitatively the values deviated considerably. This can be explained as follows. There was an uncertainty between the location of the temperatures used for the computed ΔT-ratios and the location of temperature measurements in the clinic. The CT scan was a pre-treatment scan and, therefore, the exact locations of thermometry were not visible on this CT. Consequently, an exact correspondence can hardly be expected. Furthermore, deviations in SAR can be introduced by uncertainties in the dielectric properties. Dielectric properties were obtained from literature and the reported values show a large spread. Apart from the organs which were outlined manually, all tissue was assigned the dielectric properties of either fat, muscle, bone, lungs or inner air. Dielectric imaging techniques, such as MRI-based techniques, can be useful to obtain a complete map with dielectric tissue properties Citation[27], Citation[28]. In the future, these techniques will probably have an important role in hyperthermia treatment planning.
The numerical amplitude/phase optimization was based on steady state temperatures and accurate temperature calculations are complicated by uncertainties in thermal properties, especially the perfusion rate. The impact of the normal tissue constraints in the optimization is strongly determined by local tissue perfusion. Perfusion values were obtained from the literature, but these values show a large spread and can be different for individual patients. Furthermore, different organs were segmented as the same tissue type, while their perfusion might be totally different (e.g. muscle and liver). Availability of a complete perfusion map for individual patients can improve the reliability of temperature calculations. Contrast-enhanced multi-slice CT techniques can be applied to obtain information about the local perfusion Citation[29], but the values obtained by these techniques are only valid under normothermic conditions, so elevated perfusion levels under hyperthermic conditions remain unknown. Perfusion values can also be estimated from thermal decay measurements at the end of treatment Citation[30–32]. This method provides an estimation of the local perfusion under hyperthermic conditions, but it is limited to the locations of clinical thermometry.
Asymmetry in numerically optimized settings
The settings prescribed by the numerical optimization showed a strong asymmetry in the power supplied by the left and right antenna (). Despite the relatively low amount of power supplied by the left antenna, an arising hot spot of 42°C was observed between the tumour and the left antenna. In addition to the left antenna, the top and bottom antenna also contribute to this arising hot spot. The distance to the tumour is shorter for the bottom and top antenna than for the left and right antenna; therefore, it is more optimal for tumour heating to set the amplitude of the top and bottom antenna as high as possible, resulting in a lower amount of power supplied by the left antenna. The anatomy in shows that the left side of the patient has a higher inhomogeneity in tissue properties than the right side. The liver is a relatively large organ, which is situated at the right side and was segmented as homogeneous muscle tissue. Hot spots will generally occur at tissue boundaries, so the asymmetric anatomy may provide an explanation for the asymmetric settings in . The numerical optimization prescribed similar asymmetric amplitude settings for the left and right antenna for almost all 16 patients planned. Optimization on ΔT-ratio, however, did not take normal tissue hot spots into account (with exception of the spinal cord). This example showed that the asymmetry between the power supplied by the left and right antenna disappears when asymmetries in the anatomy are not taken into account.
Multi-variate analysis
Examination of the relation between initial ΔT and the amplitude/phase settings of the different antennae together with the patients’ diameters yielded little results. The relatively low partial correlation coefficients may be due to the limited spread in input, since only calculated and clinical amplitudes and phases were included in this analysis. The correlation coefficients mentioned in the Results should, therefore, be regarded as indication only. The ΔT at the oesophageal tumour depended mainly on the patient's diameters. The partial correlations with the diameters were negative, which implies that larger diameters result in lower ΔT values. This is due to the fact that the amount of power reaching the tumour decreases with increasing diameters, because of the fast decay of the electromagnetic field. The temperature rise near the spinal cord depended predominantly on the power of the top antenna, which is closest to the spinal cord. In the clinical setting, the power of the top antenna is initially set to 10% of the total power to prevent over-heating of the spinal cord and often over-heating is indeed a limitation during treatment. However, the numerical optimization frequently prescribes a higher power for the top antenna. This suggests that during the optimization procedure destructive interference is applied to suppress the spinal cord temperature. Since the other antennae do contribute to ΔTcord, the patient's dimensions also had a significant influence.
The research presented here is a first step in the clinical application of hyperthermia treatment planning. In the near future, more aspects of the clinical application of treatment planning are to be studied. Numerical optimization on ΔT-ratio can possibly improve tumour temperatures. In the demonstrated example, steady state tumour temperatures were not very impressive, but the power of the top antenna can be substantially increased without over-heating the spinal cord, since the steady state temperature in the spinal cord was predicted to remain below 38°C. Such a strategy may lead to an improvement of tumour temperatures in the clinic, since the top antenna contributes more effectively to tumour heating than the left and right antennae. However, when considering optimization on ratio it would be more appropriate for clinical application to optimize the ratio of the steady state temperatures instead of the ΔT-ratio. A demerit of optimization on ratio is that hot spots in normal tissue are generally not taken into account, so this will still not provide the real clinically optimal amplitude/phase settings. The desired clinically optimal amplitude/phase settings have to yield the highest possible tumour temperatures, while preventing toxicities in normal tissue. Therefore, a thorough discussion on clinically relevant objectives and constraints is essential.
Conclusion
Treatment planning can be useful to improve hyperthermia treatments by more effective tumour heating. The same tumour heating with less applied power is expected to be of benefit for the patient, since it probably results in a reduction of systemic stress and possibly fewer treatment limiting hot spots. Consequently, higher tumour temperatures can be achieved in the clinic without increasing the amount of applied power. The applied objective function and constrains for numerical optimization are crucial for the obtained settings, so a thorough discussion on clinically relevant objectives and constraints is essential.
Acknowledgement
This study is supported by the Dutch Cancer Society grant 2002-2622.
References
- Van Dijk JDP, Schneider CJ, Van Os RM, Blank LE, Gonzalez DG. Results of deep body hyperthermia with large waveguide radiators. Adv Exp Med Biol 1990; 267: 315–319
- Oleson JR, Samulski TV, Leopold KA, Clegg ST, Dewhirst MW, Dodge RK, George SL. Sensitivity of hyperthermia trial outcomes to temperature and time: Implications for thermal goals of treatment. Int J Radiat Oncol Biol Phys 1993; 25: 289–297
- Wust P, Stahl H, Loffel J, Seebass M, Riess H, Felix R. Clinical, physiological and anatomical determinants for radiofrequency hyperthermia. Int J Hyperthermia 1995; 11: 151–167
- Hand JW, Machin D, Vernon CC, Whaley JB. Analysis of thermal parameters obtained during phase III trials of hyperthermia as an adjunct to radiotherapy in the treatment of breast carcinoma. Int J Hyperthermia 1997; 13: 343–364
- Sapareto SA, Dewey WC. Thermal dose determination in cancer therapy. Int J Radiat Oncol Biol Phys 1984; 10: 787–800
- Bardati F, Borrani A, Gerardino A, Lovisolo GA. SAR optimization in a phased array radiofrequency hyperthermia system. Specific absorption rate. IEEE Trans Biomed Eng 1995; 42: 1201–1207
- Wust P, Seebass M, Nadobny J, Deuflhard P, Monich G, Felix R. Simulation studies promote technological development of radiofrequency phased array hyperthermia. Int J Hyperthermia 1996; 12: 477–494
- Das SK, Clegg ST, Samulski TV. Electromagnetic thermal therapy power optimization for multiple source applicators. Int J Hyperthermia 1999; 15: 291–308
- Wiersma J, Van Maarseveen RAM, Van Dijk JDP. A flexible optimization tool for hyperthermia treatments with RF phased array systems. Int J Hyperthermia 2002; 18: 73–85
- Das SK, Clegg ST, Samulski TV. Computational techniques for fast hyperthermia temperature optimization. Med Phys 1999; 26: 319–328
- Nikita KS, Maratos NG, Uzunoglu NK. Optimal steady-state temperature distribution for a phased array hyperthermia system. IEEE Trans Biomed Eng 1993; 40: 1299–1306
- Wiersma J, Van Wieringen N, Crezee H, Van Dijk JDP. Delineation of potential hot spots for hyperthermia treatment planning optimisation. Int J Hyperthermia 2006, in press
- Kok HP, Van Haaren PMA, Van de Kamer JB, Wiersma J, Van Dijk JDP, Crezee J. High-resolution temperature-based optimization for hyperthermia treatment planning. Phys Med Biol 2005; 50: 3127–3141
- Van de Kamer JB, De Leeuw AAC, Kroeze H, Lagendijk JJW. Quasistatic zooming for regional hyperthermia treatment planning. Phys Med Biol 2001; 46: 1017–1030
- Albregts M, Hulshof MCCM, Zum Vörde Sive Vörding PJ, van Lanschot JJB, Richel DJ, Crezee H, Fockens P, van Dijk JDP, González González D. A feasibility study in oesophageal carcinoma using deep loco-regional hyperthermia combined with concurrent chemotherapy followed by surgery. Int J Hyperthermia 2004; 20: 647–659
- Van de Kamer JB, De Leeuw AAC, Hornsleth SN, Kroeze H, Kotte ANTJ, Lagendijk JJW. Development of a regional hyperthermia treatment planning system. Int J Hyperthermia 2001; 17: 207–220
- Hornsleth SN, Mella O, Dahl O. A new segmentation algorithm for finite difference based treatment planning systems. Hyperthermic oncology 1996, C Franconi, G Arcangeli, R Cavaliere. Tor Vergata, RomeItaly 1996; 2: 521–523
- James BJ, Sullivan DM. Creation of 3-dimensional patient models for hyperthermia treatment planning. IEEE Trans Biomed Eng 1992; 39: 238–242
- Gabriel C, Gabriel S, Corthout E. The dielectric properties of biological tissues: I. Literature survey. Phys Med Biol 1996; 41: 2231–2249
- ESHO Taskgroup Committee. Treatment planning and modelling in hyperthermia, a task group report of the European society for hyperthermic oncology. Tor Vergata, RomeItaly 1992
- Song CW. Effect of local hyperthermia on blood flow and microenvironment: A review. Cancer Res 1984; 44: 4721s–4730s
- De Leeuw AAC, Crezee J, Lagendijk JJW. Temperature and SAR measurements in deep-body hyperthermia with thermocouple thermometry. Int J Hyperthermia 1993; 9: 685–697
- Gellermann J, Wust P, Stalling D, Seebass M, Nadobny J, Beck R, Hege H, Deuflhard P, Felix R. Clinical evaluation and verification of the hyperthermia treatment planning system hyperplan. Int J Radiat Oncol Biol Phys 2000; 47: 1145–1156
- Sreenivasa G, Gellermann J, Rau B, Nadobny J, Schlag P, Deuflhard P, Felix R, Wust P. Clinical use of the hyperthermia treatment planning system HyperPlan to predict effectiveness and toxicity. Int J Radiat Oncol Biol Phys 2003; 55: 407–419
- Van Haaren PMA, Van den Berg CAT, Kok HP, Zum Vörde Sive Vörding PJ, Oldenborg S, Stalpers LJA, De Leeuw AAC, Crezee J. Verification of hyperthermia treatment planning in cervix carcinoma patients using invasive thermometry. ESHO-2005: Abstracts. Graz. European Society for Hyperthermic Oncology, Austria 2005; 36–37
- De Leeuw AAC, Van den Berg CAT, Van de Kamer JB, Van Vulpen M, Lagendijk JJW. Regional hyperthermia treatment planning: Comparison with clinical derived absorbed power profiles. Physica medica. European Federation of Organisations for Medical Physics, EindhovenThe Netherlands 2003; 50–51
- Farace P, Pontalti R, Cristoforetti L, Antolini R, Scarpa M. An automated method for mapping human tissue permittivities by MRI in hyperthermia treatment planning. Phys Med Biol 1997; 42: 2159–2174
- Van Wieringen N, Crezee J, Van de Kamer JB, Bakker CJG, Lagendijk JJW. Estimation of dielectric tissue properties for hyperthermia treatment planning. ESHO-98: Abstracts. European Society for Hyperthermic Oncology, NancyFrance 1998; 46
- Van den Berg CAT, van de Kamer JB, De Leeuw AAC, Jeukens CRLPN, Raaymakers BW, Van Vulpen M, Lagendijk JJW. Towards patient specific thermal modelling of the prostate. Phys Med Biol 2006; 51: 809–825
- Waterman FM, Nerlinger RE, Moylan DJ, III, Leeper DB. Response of human tumor blood flow to local hyperthermia. Int J Radiat Oncol Biol Phys 1987; 13: 75–82
- Diederich CJ, Clegg S, Roemer RB. A spherical source model for the thermal pulse decay method of measuring blood perfusion: A sensitivity analysis. J Biomech Eng 1989; 111: 55–61
- Van Vulpen M, Raaymakers BW, Lagendijk JJW, Crezee J, De Leeuw AAC, Van Moorselaar JRA, Ligtvoet CM, Battermann JJ. Three-dimensional controlled interstitial hyperthermia combined with radiotherapy for locally advanced prostate carcinoma—a feasibility study. Int J Radiat Oncol Biol Phys 2002; 53: 116–126